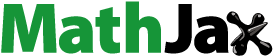
ABSTRACT
In this work, we reported a novel emulsion copolymerization of vinyl chloride monomer (VCM) with amphipathic monomer poly (ethylene glycol) methyl ether methacrylate (PEGMA) by using sodium dodecyl sulfate (SDS) as emulsifier and K2S2O8/NaHSO3 as redox initiator. Owing to the multifunctionality of PEGMA that can serve as co-monomer and ‘emulsifier’, the emulsion copolymerization is achieved successfully and the interesting results demonstrate quite different features such as:(1) the stable P[(VC)-co-(PEGMA)] latex is obtained only when the mass ratio of PEGMA > 10% (to total monomer mass), (2) a small amount of ionic emulsifier SDS is required to achieve a stable latex, and (3) the mechanism of the micellar formation is different from the typical emulsion polymerization. Unexpectedly, the obtained copolymer formed high content of gel fraction which is mainly caused by the chain transfer reaction of the PVC growth chain radical to the hydrogen atom of the methylene from alkoxy (-OCH2CH2-) of the PEGMA oligomer chain. However, it could be inhibited effectively by the addition of chain transfer agent 2-mercaptoethanol (2-MCE). Subsequently, the properties of as-obtained P[(VC)-co-(PEGMA)] copolymer are evaluated carefully and it exhibited unique features such as self-plasticization, lower Tg (decreased from 83.1 to 59.6 °C), higher heat resistance (increased from 270 to 290 °C), hydrophilic, and high transparent in comparison to pure PVC. These outstanding features of the P[(VC)-co-(PEGMA)] copolymer offered a potential route for the functional modifications of PVC and broadened its further use.
1. Introduction
Poly(vinyl chloride) (PVC) is one of the world’s major thermoplastics and produced worldwide for its superior mechanical and physical properties and is widely applicated in consumer products ranging from packaging to construction materials to health care devices [Citation1–3]. PVC resin is mainly produced by suspension polymerization, emulsion polymerization, and bulk polymerization. The production processes are geared to the end-usage and applications. For example, the resin produced by suspension polymerization, which accounted for more than 80% of PVC products, is widely used as pipes, window profiles, house siding, wire cable insulation and flooring etc. While PVC resin produced by emulsion polymerization is mainly used as artificial leather, medical glove, coating, and inks [Citation4,Citation5].
The advantage of emulsion polymerization is that the obtained latex can be directly used as product without further treatment. Commonly, water is the reaction medium, which can reduce the use of volatile organic solvents and improve the transfer of reaction heat [Citation6]. On the other hand, both of high conversion and molecular weight of polymer latex can be achieved concurrently stemming from its polymerization mechanism features [Citation7]. In 1940s, Harkins, Smith, and Ewart assumed the classical theory of emulsion polymerization based on micellar nucleation [Citation8]. They proposed the polymerization mainly occurred in the micelle, which requires the monomer with extremely low water solubleness. The fact that radicals are compartmentalized within particles and hence cannot terminate with a polymeric radical within another particle under certain circumstances, relative higher polymerization rates and molecular weights can be achieved in comparison to that of bulk or suspension polymerizations [Citation6,Citation7]. While with the in-depth study of theory, it is found that the mechanism of emulsion polymerization of vinyl chloride monomer (VCM) does not agree with the classical theory well [Citation9–12]. Actually, the VCM has a certain solubility (~1.8 g/L) in water phase at ambient temperature. Moreover, the amount of VCM in water phase was further increased with the decrease of temperature. In the case of emulsion polymerization, water soluble initiators are applied and polymerization takes place either in the micelles or in water. Thus, homogeneous nucleation cannot be negligible, which leading to a remarkable difference in reaction kinetics in comparison to those of water insolvable monomers such as styrene [Citation13]. With the further development of theoretical research and industrial practices, several novel approaches such as seed emulsion polymerization [Citation14,Citation15], microemulsion [Citation16], and soap-free emulsion polymerization [Citation17,Citation18] have been derived from conventional emulsion polymerization.
As we all know that the fluid plasticity and thermal stability of PVC are inferior to those of other commodity plastics such as polyethylene, polypropylene, and polystyrene, mainly related to its inherent structural features such as strong interaction in polymer chains and structural defects [Citation19,Citation20]. Emulsion copolymerization of VCM with other monomers is a simple but effective technology to achieve desirable high-performance PVC-based materials. It should be noted that VCM is classified as a non-conjugated weak electron-withdrawing vinyl monomer; it can be copolymerized readily with non-conjugated monomers (eg., olefins, vinyl ether, vinyl ester). The binary copolymers of poly[(vinyl chloride)-co-(isobutyl vinyl ether)] [Citation21], poly[(vinyl chloride)-co-(vinyl acetate)] [Citation22,Citation23], and ternary copolymer poly[(vinyl chloride)-co-(vinyl acetate)-co-(maleic anhydride)] [Citation24] have been produced successfully by emulsion polymerization. VCM is not easy to copolymerize with conjugated monomers like styrene and acrylates [Citation25,Citation26]. It is widely accepted that the copolymerization of vinyl chloride with acrylate monomer inevitably formed acrylate homopolymers due to the much higher reactivity ratio of acrylate monomers. Seed emulsion copolymerization [Citation27] and emulsion grafting polymerization [Citation28] provided routes to deal with the copolymerization issues. Zhang et al [Citation29] synthesized poly[(butyl acrylate)-co-(ethylhexyl acrylate)]/poly(vinyl chloride) [P(BA-EHA)/PVC] core-shell structured particles, exhibiting potential as an impact modifier for PVC.
It is worthy noted that poly(ethylene oxide) (PEO) is one of commonly used steric stabilizers to stabilize the polymer dispersions in aqueous phase by forming a dense protective layer on the latex particles [Citation30]. Among various functional comonomers, poly(ethylene glycol) methyl ether methacrylate (PEGMA) is an amphipathic acrylate monomer with non-toxicity and biocompatibility, and it is commonly utilized to modify PVC and endow it with unique properties such as antifouling and hydrophilicity [Citation31,Citation32]. Recently, Carlos et al. [Citation33] synthesized an internal plasticized PVC by free radical copolymerization of VCM with oligo(ethylene oxide) methyl ether acrylate (OEOA) [P(VC)-co-(OEOA)]. The reduction in Tg of typical PVC from 90 °C to −16.2 °C confirmed the plasticization efficiency of OEOA, and the single Tg values suggest excellent miscibility between PVC and POEOA segments. However, to our best knowledge, the emulsion copolymerization of PEGMA and VCM has not been reported in open literature yet. In this study, poly(ethylene glycol) methyl ether methacrylate (PEGMA), a novel macromonomer having a relatively shorter chain-length, is employed for the synthesis of poly[(vinyl chloride)-co-(poly(ethylene glycol) methyl ether methacrylate)] P[(VC)-co-(PEGMA)] copolymer via emulsion copolymerization, aiming to reveal the intrinsic features of the emulsion copolymerization of VCM with amphipathic comonomer. The effect of the polymerization conditions, such as comonomer concentration, emulsifier dosage, comonomer feed ratios, and chain transfer agent on the monomer conversion, particle size of latex, number-average molecular weight, chemical composition, were investigated systematically. According to the experimental data, a plausible mechanism of the emulsion copolymerization was proposed. Finally, the properties such as thermal stability and hydrophilic of the copolymer were also evaluated.
2. Experimental
2.1 Materials
Vinyl chloride monomer (VCM, 99.9 wt%) was supplied by Dalian Special Gases Co., Ltd. Potassium persulfate (K2S2O8, AR) was supplied by Sinopharm Chemical Reagent Co., Ltd. Sodium bisulfite (NaHSO3, AR) and sodium bicarbonate (NaHCO3, AR, used as pH buffer reagent) was supplied by Fuchen (Tianjin, China) Chemical Reagent Co., Ltd. Sodium dodecyl sulfate (SDS, CP) was supplied by Xilong Chemical Co., Ltd. Poly(ethylene glycol) methyl ether methacrylate (PEGMA, Mw ~475) was supplied by Shanghai Aladdin Bio-Chem Technology Co., Ltd. 2-Mercaptoethanol (2-MCE, >98.0 %) was purchased from TCI (Shanghai, China), Ltd. Ethanol (AR) and tetrahydrofuran (THF, AR) were supplied by Tianjin Fuyu Fine Chemical Co., Ltd and used as received.
2.2 Emulsion polymerization of VCM with PEGMA
The emulsion copolymerization of VCM and PEGMA was carried out at 45 °C in a 100 mL reaction kettle with a mechanical stirrer, pressure, and temperature sensors. The copolymerization recipes were showed in . Taking entry 10 as a typical run to describe the detailed procedure. Briefly, deionized water (60.0 mL), K2S2O8 (0.12 g, 0. 44 mmol), NaHSO3 (0.08 g, 0.77 mmol), NaHCO3 (0.10 g, 1.19 mmol), and the predetermined amount of SDS, 2-MCE, and PEGMA were successively added into a reaction kettle. The mixture was purged with argon several times to remove oxygen from the reactor. Then the reactor was cooled with liquid nitrogen and required amount of VCM was charged. After thawing the mixture and checking the leakage, pre-emulsification was carried out at 400 rpm for 30 min at room temperature to mix the emulsifier and the monomer droplets evenly. Then, the reactor was heated to 45 °C with a stirring rate at 400 rpm for 8 h. At the end of the polymerization, unreacted VCM was removed and the copolymer emulsion was poured into a beaker. By adding amount of ethanol into the beaker to make the demulsification, and then the sedimentation product was obtained by centrifugation at 6000 r/min for 5 min. The precipitates were dried to constant weight under vacuum for subsequent measurement.
Table 1. Recipes for the emulsion copolymerization of VCM with PEGMA*.
2.3 Characterization
The monomer conversions at certain reaction times were determined by gravimetric method, and the calculation formula is described as follows:
where WP is the weight of polymer (after dried) and WM is the weight of total monomers. The number average molecular weight (Mn) and dispersity (Ð=Mw/Mn) were determined by gel permeation chromatography (GPC) on a Waters 1515 system with Styragel HT 3, 4, and 5 columns in series with THF as the eluent at a flow rate of 1 mL/min. The GPC was calibrated with polystyrene standards. Fourier transform infrared spectroscopy (FTIR) was recorded on a Thermo Nicolet Nexus 670 spectrometer at ambient temperature with the resolution of 4 cm−1 and 32 times scanning. 1H NMR spectroscopy was conducted using THF-d8 as the solvent on a Bruker Avance 400 spectrometer. The morphology of the copolymer latex was observed by using scanning electron microscopy (SEM, S-4700, Hitachi). The drop of diluted emulsion was added onto a silicon wafer and the water was evaporated completely at room temperature. Finally, the dried sample was sputtered with Au for 15 s to improve the quality of the images. The thermal behavior of the copolymer was studied by differential scanning calorimetry (DSC, TA Q20). The samples were heated from 2 to 150 °C at 10 °C/min and then cooled to −60 °C for the elimination of thermal history. Then, the samples were heated again from −60 to 150 °C at a heat rate of 5 °C/min. The thermal stability of the copolymer was investigated by thermogravimetric analysis (TGA2, Mettler Toledo), the analysis was made between 40 and 600 °C under a nitrogen atmosphere with a heating rate of 10 °C/min. The transmittance of copolymer film was characterized by an UV-vis spectrophotometer (UV, CINRA20) with the wavelength range from 500 to 800 nm. The film was prepared by casting the copolymer/THF solution on a glass slide and then volatilize the solvent completely at 60 °C. The contact angles of water droplets on the copolymer films were recorded by Contact Angle system OCA (OCA20, U.S.A.). Gel content of the obtained copolymer was calculated by extracting an appropriate amount of the copolymer with THF for 48 h, and three specimens per copolymer were evaluated.
3. Results and discussions
3.1 The effect of monomer feed ratios and SDS contents on emulsion copolymerization of PEGMA with VCM
At first, the conventional emulsion polymerization of VCM was carried out by using SDS as emulsifier and K2S2O8/NaHSO3 as redox initiator. A stable PVC latex was synthesized successfully and at least 0.2 wt% SDS (to total mass of VCM) was required to afford sufficient emulsifying capacity. (). Subsequently, we conducted the emulsion copolymerization of VCM with PEGMA at a fixed content of SDS (1 wt%). Due to the unique features of PEGMA such as high reactivity and hydrophilicity, the first set of experiments were carried out to investigate the effect of monomer feed ratios on the emulsion copolymerization. As shown in , an interesting result demonstrated that the polymerization process was highly relent on the content of PEGMA. The reaction temperature was increased uncontrollably and the agglomeration polymer product was formed when the amount of PEGMA was lower than 10%. While a stable emulsion colloid was obtained successfully when the PEGMA content was increased to 10%. Continuously increasing the PEGMA to 15%, even to 20%, the emulsion colloid still maintained stable even after 60 days (). According to the kinetic curves (Figure S1), the high PEGMA content in the reactant can accelerate the polymerization rate dramatically, which in accordance with the dramatical decreases in the vapor pressure. showed the SEM micrographs and particle sizes distribution (DLS) of EPVC and copolymer latex. The morphology of EPVC was much smooth and uniform than that of P[(VC)-co-(PEGMA)]. However, the copolymer particles became rough and nonuniform with the increase of PEGMA mass fraction. PEGMA is an amphiphilic monomer and potentially act as a partial emulsifier during the emulsion copolymerization. Based on the thermodynamic criterion, the final morphology of the particles could minimize the Gibb’s interfacial free energy of the system, so the PEGMA oligomers were mainly distributed on the surface of the particles, which might cause the bulges on the particle surface. On the other hand, more PEGMA on the surface of latex led to the conglutination of particles, which was highly agreed with the date of DLS.
Figure 1. (a) EPVC with different SDS usage; (b) P[(VC)-co-(PEGMA)] with different PEGMA mass ratio in monomer feeds; (c) digital photos and (d) SEM images and DLS of EPVC and copolymer latex with varied PEGMA mass ratio.
![Figure 1. (a) EPVC with different SDS usage; (b) P[(VC)-co-(PEGMA)] with different PEGMA mass ratio in monomer feeds; (c) digital photos and (d) SEM images and DLS of EPVC and copolymer latex with varied PEGMA mass ratio.](/cms/asset/adb123bf-9ca4-4130-9863-fe7571b8ff45/tdmp_a_2285087_f0001_oc.jpg)
To further investigate the role of SDS and PEGMA in the present system, we carried out the emulsion copolymerization of PEGMA with VCM at a fixed monomer feed ratio (PEGMA: VCM = 20:80) under varied SDS content. As showed in digital photos (Figure S2), it can be drawn that at least 0.2% SDS was required to form micelles and afford sufficient places for the co-polymerization of VCM. Interestingly, in the present of PEGMA, only 0.05% SDS can maintain the copolymer emulsion colloid with excellent stability, which is quite lower than the CMC value of SDS. Also, the copolymer latexes were uniform in micromorphology (). However, the emulsion colloid system was quite unstable and several latexes aggregated together and were covered with polymer film severely if without any SDS was used (). Meanwhile, we also tried to maintain the stability of emulsion copolymerization in virtue of vigorous stir by increasing the stir rate from 400 to 800 rpm, but the final mixture layered obviously when the external force was removed (). These results highly indicated the absolute necessity both of SDS and PEGMA in the present system. Maybe the nuclei formation mechanism based on the synergy effect of SDS and PEGMA resulted the unique features of the present emulsion copolymerization, which was discussed later.
3.2 The emulsion polymerization of PEGMA with VCM in the presence of chain transfer agent
Although PEGMA can copolymerize with VCM via emulsion polymerization in the present conditions, a relatively high gel content formed inevitably, which brought troubles for the further applications. This situation was also reported in the solution polymerization of PEGMA in water; the resulting poly-PEGMA could not be dissolved either in THF or in DMF [Citation34]. Maybe the relatively high molecular weight and the crosslink that mainly caused by the chain transfer reaction of the PVC growth chain radical to the hydrogen atom of the methylene from alkoxy (-OCH2CH2-) of the PEGMA oligomer chain generated the formation of gel (). To testify the assumption, the strategy by utilizing chain transfer agent to inhibit the formation of gel was adopted in the present work. Thiol compounds were usually used as chain transfer reagent in free radical polymerization [Citation35,Citation36]. Due to the weakness of the S-H bond and the high reactivity of the thiyl radicals, a growing chain radical abstracted a hydrogen atom from the chain transfer agent easily and thereby terminated the polymer growth.
Figure 3. The gel content of the P[(VC)-co-(PEGMA)] copolymer with different concentration of 2-mercaptoethanol.
![Figure 3. The gel content of the P[(VC)-co-(PEGMA)] copolymer with different concentration of 2-mercaptoethanol.](/cms/asset/10c15084-fe5f-4d8b-bf23-15299780cfa9/tdmp_a_2285087_f0003_oc.jpg)
Herein, 2-mercaptoethanol (2-MCE) was used in the present emulsion copolymerization system to tune the gel content of the final products. As showed in , the addition of 2-MCE can reduce the gel content dramatically. For instance, the gel content lower than 5% only 0.05 wt% 2-MCE was used. With continuously increase the amount of 2-MCE to 0.10 wt%, there was almost no detectable gel content after extraction, indicating the high efficiency of 2-MCE for the inhibition of gel content.
By measuring the GPC trances (), the number-average molecular weight (Mn) of obtained copolymer reduced obviously with the increase of 2-MCE. Additionally, the addition content of chain transfer agent exhibits diverse feature in the polymerization rate at different monomer feed ratio. At a fixed concentration of 2-MCE (0.1 wt%), inconspicuous influence on the polymerization rate when the PEGMA was 10 wt% (), while the polymerization rate reduced significantly, e.g., the monomer conversion decreased from 88% to 72%, and from 80% to 56%, with the PEGMA increased from 15 wt% to 20 wt% (), demonstrating that the chain transfer reaction mainly tuned the growth chain of PEGMA.
Figure 4. The monomer conversion (wt %) vs. reaction time (min) for the copolymerization at varied concentration of 2-MCE: (a) VCM/PEGMA=18: 2; (b) VCM/PEGMA=17: 3; (c) VCM/PEGMA=16: 4; the GPC traces of copolymer samples with different 2-MCE amounts: (d) VCM/PEGMA=18: 2; (e) VCM/PEGMA=17: 3; (f) VCM/PEGMA=16: 4.

Subsequently, the morphologies of P[(VC)-co-(PEGMA)] copolymer at different concentration of 2-MCE were presented in . Obviously, all the shapes of the P[(VC)-co-(PEGMA)] particles showed much smoother and more uniform in particle morphology with the lightly increase of 2-MCE content, while the size of copolymer latexes decreased and the PSD became narrow. At a fixed content of 2-MCE, the P[(VC)-co-(PEGMA)] latex has an increment in particle size from 150 to 300 nm and turned irregularity in morphology with the increase of PEGMA. It can be inferred that the PEGMA chain radical transferred to 2-MCE can significantly decrease the Mn of the P[(VC)-co-(PEGMA)] copolymer and regulate the polymer composition. The appropriate polymer composition was beneficial to form uniform latex. While the increase of PEGMA portion in the copolymer increased the critical chain length, leading to the fewer nuclei number in the reactant system, thereby causing the formation of big size of latex.
Figure 5. SEM micrographs of copolymer particles and the particle size and distribution with different concentration of 2-MCE (0.05 wt%, 0.10 wt%):(a)VCM: PEGMA=18: 2; (b) VCM: PEGMA=17: 3; (c) VCM: PEGMA=16: 4.

The successful copolymerization of P[(VC)-co-(PEGMA)] has been confirmed by using 1H NMR spectroscopy. From the 1H NMR spectra showed in , three peaks with chemical shifts at around 4.7–4.2 ppm are attributed to proton of -CHCl- in PVC. In the side chain structure of the copolymer, the chemical shift of the methylene hydrogen proton directly connected to the ester group (-COOCH2-) at around 4.2 ppm. The methylene hydrogen proton peak of the polyethylene glycol units at around 3.6 ppm, overlapping with one of the solvent peaks of THF. The peak at 3.3 ppm was attributed to the methoxy hydrogen proton peak in the end of PEGMA [Citation37]. Subsequently, the mass ratio of PEGMA in the P[(VC)-co-(PEGMA)] copolymers was calculated by comparing integral areas and the calculation formula was EquationEquation (2)(2)
(2) :
Figure 6. (a) 1H NMR spectra of P[(VC)-co-(PEGMA)] copolymers in THF-d8;The mass fraction of PEGMA and VCM in the copolymer vs. reaction time (h): (b) VCM: PEGMA=18: 2; (c) VCM: PEGMA=17: 3; (d) VCM: PEGMA=16: 4; (e) the final mass fraction curves of PEGMA in the copolymer composition.
![Figure 6. (a) 1H NMR spectra of P[(VC)-co-(PEGMA)] copolymers in THF-d8;The mass fraction of PEGMA and VCM in the copolymer vs. reaction time (h): (b) VCM: PEGMA=18: 2; (c) VCM: PEGMA=17: 3; (d) VCM: PEGMA=16: 4; (e) the final mass fraction curves of PEGMA in the copolymer composition.](/cms/asset/6f3379ae-dc3a-45a1-82af-4c3b052d2301/tdmp_a_2285087_f0006_oc.jpg)
where WPEGMA is the mass fraction of PEGMA in the copolymer, SPEGMA and SVCM are the integral areas of the two monomers in the 1H NMR spectra, respectively. The mass fraction of PEGMA and VCM in the copolymer versus reaction time with the different initial monomer feed ratio (2-MCE addition was 0.05 wt%) were shown in . It can be observed that the most PEGMA monomer participated in copolymerization at the early stage before 2 h. The dependence of final mass fraction of PEGMA in the copolymer at different 2-MCE addition were also summarized in . The proportion of PEGMA in the copolymer structure increased accordingly with the monomer feed ratio but lower than the theoretical values because of the water solubility of PEGMA. However, the content of PEGMA segment in the copolymer at a fixed monomer ration was higher with the increasing of 2-MCE content. When the ratio of VCM/PEGMA was 16:4, the maximum of WPEGMA in the copolymer was 14.81 wt%, which was lower than the monomer feed ratio. The reason might be attributed to the significant influence of chain transfer agent. Without chain transfer agent, more PEGMA monomer homopolymerized in the aqueous phase. While once 2-MCE was added, the homo-polymerization of PEGMA monomer was inhibited, and more PEGMA monomer entered the latex micelle to copolymerize with VCM.
Besides, the influence of SDS concentrations on the emulsion copolymerization in the presence of chain transfer agent was evaluated. At a fixed monomer feed ratio (VCM: PEGMA = 16:4) and concentration of 2-MCE (0.05 wt%), the polymerization rate was decelerated and the Mn decreased obviously with the reduction of SDS concentrations (as shown in ). Although, the SDS was lower to 0.1%, the emulsion colloid was still stable (). On the one hand, both decrease of SDS and the rapid consumption of PEGMA caused low diffusion of VCM in water phase and resulted to a low concentration of monomer droplet. On the other hand, the charge layer on the micelles was attenuated with the decrease of SDS and thereby caused ununiform in the particle morphology, which was highly verified with the SEM images and relative wide PDI of the polymer latex (). This result further evidenced the indispensable role of SDS in the present emulsion copolymerization system.
3.3 Assumed emulsion polymerization mechanism
According to the classical emulsion polymerization theory and the polymerization behaviors of the present system, a plausible mechanism that mainly arise from the homogeneous nucleation with the synergy effect of SDS was proposed (). Prior to the occurrence of the polymerization, PEGMA, initiator, and a little amount of VCM was completely dissolved in water. VCM mixed with a little amount of PEGMA formed monomer droplets and they were stabilized by absorbing SDS and partial PEGMA on their surface. When the primary radical was produced, it preferentially initiated the copolymerization of PEGMA with VCM in water phase. The produced copolymer precipitated from the water phase and formed nuclei due to its amphipathicity. The high polymerization activity of PEGMA resulted relatively high PPEGMA segments in the copolymer at the initial stage of polymerization, which increase the stickiness of the polymer latex, thereby necessarily required the help of SDS to maintain the stability of the polymer latex. The stable emulsion colloid, which can be obtained even below CMC of SDS, was the direct evidence for this assumption. When the initial amount of total PEGMA was low, the homogeneous nucleation was unstable and could be broken down when copolymerized with a little amount of VCM. The high PEGMA content in the system further increased the solubility of VCM in water phase and beneficial to form more stable nuclei, thereby can enhance the stability of the produced copolymer particles. As a result, the synergistic effect between SDS and PEGMA was the main way to maintain the stability of as resulted copolymer latex.
3.4 Property of P[(VC)-co-(PEGMA)] copolymer
It is reported that the introduced copolymer segments in the PVC main chain can afford ‘free volume’ for the movement of PVC polymer chains [Citation38,Citation39]. As displayed in , the Tg of the copolymer shifted to the low temperature gradually with the increase of PEGMA mass fraction in copolymers, while the 2-MCE has no significant influence on the Tg . Thus, as a ‘soft’ monomer with a low Tg (~-70°C), the introduction of PEGMA segment in the PVC main chains can endow PVC copolymer with ‘internal plasticization’ features. On the other hand, the copolymerized segments can hinder the zippered elimination of HCl and enhance heat resistance. As control (), the thermogravimetric curves of PVC show two definite weight losses. The first stage occurred in a 250–375 °C with the maximum temperature rate at 270 °C attributed to dehydrochlorination. The mass loss of this stage was about 60%, which was in good agreement with typical reports in the literature [Citation40,Citation41]. The second degradation stage occurred in the temperature range of 380–560 °C with a maximum degradation rate of 460 °C was ascribed to the degradation of the non-volatile products. After being copolymerized with PEGMA and with the tuning molecular weight with the addition of 2-MCE, the difference in the thermogravimetric curve of the copolymer was mainly reflected on the first weight loss stage. The temperature of the maximum rate increased from 270 °C to 290 °C, and the mass loss fraction increased from around 60% to 70%. With the increasing of 2-MCE concentration, the rate of dehydrochlorination reduced slowly. According to the summarized data in , we can draw the conclusion that the initial decomposition temperature of all the copolymers was higher than that of EPVC. This was mainly benefited from the reduction of interchain polarity by introducing the PEGMA segment in PVC. However, the T5 and T10 were a little low in comparison to EPVC. The thermal stability of the P[(VC)-co-(PEGMA)] copolymer was slightly reduced after the addition of 2-MCE because the Mn of the copolymer decreased.
Figure 9. Characterization of copolymer properties: (a) the Tg of the copolymers; (b) the water contact angles of the polymer particle films; (c) the thermogravimetric curves.

Table 2. The thermal decomposition temperature of P[(VC)-co-(PEGMA)] copolymer with different 2-MCE concentration.
It was well known that the transmittance can directly reflect the miscibility of copolymer if phase separation occurred in the micromorphology. Interestingly, the transmittance of all the copolymers were higher than that of EPVC (shown in Figure S4). Meanwhile, the transmittance was further increased with the addition of 2-MCE. This was mainly due to the excellent inter-plasticization of PEGMA, which can significantly disrupt the microcrystal of PVC and enhance the flexibility, motivation, and high compatibility of polyether chain segments with PVC. Subsequently, we investigated the surface water contact angle of P[(VC)-co-(PEGMA)] copolymer film and the measurement results were presented in . Mainly owing to the inherent hydrophilic of PEGMA, the water CA of P[(VC)-co-(PEGMA)] copolymer film decreased dramatically from 92 ° (for EPVC) to 63 ° when the PEGMA was 14.8 wt% in the copolymer.
4. Conclusions
In this study, we successfully synthesized P[(VC)-co-(PEGMA)] copolymers containing varying amounts of polyethylene glycol side chains via the facile and efficient emulsion radical polymerization. The particle size of the emulsion copolymer was between 100–300 nm. Different reaction conditions (VCM/PEGMA monomer ratio, emulsifier concentration, chain transfer agent content) were explored, and different polymerization systems were compared. Structural analysis was performed by calculating monomer conversion, 1H NMR, FTIR, SEM, and GPC characterization of the emulsion copolymers. DSC and TGA analysis revealed that the single Tg values of the copolymers range from 59.6 ℃ to 71.7 ℃, indicating that PEGMA and VCM were random copolymers, and P[(VC)-co-(PEGMA)] had a good compatibility with PVC. And the thermal stability of the copolymers can be improved by adding PEGMA. At the same time, in view of the emulsifier concentration used in the obtained P[(VC)-co-(PEGMA)] copolymer emulsion can be reduced, it is expected to provide a new method of the PVC modification and achieve the desirable levels for more potential applications.
Supplemental Material
Download Zip (1.3 MB)Acknowledgments
This work was supported by the National Natural Science Foundation of China (Nos. 51988102), and Fundamental Research Funds for the Central Universities (XK2020-01).
Data availability statement
The authors confirm that the data supporting the findings of this study are available within the article and its supplementary materials.
Disclosure statement
No potential conflict of interest was reported by the authors.
Supplementary material
Supplemental data for this article can be accessed online at https://doi.org/10.1080/15685551.2023.2285087
Additional information
Funding
References
- Abreu CM, Fonseca AC, Rocha NM, et al. Poly(vinyl chloride): current status and future perspectives via reversible deactivation radical polymerization methods. Prog Polym Sci. 2018;87:34–69. doi: 10.1016/j.progpolymsci.2018.06.007
- Choi W, Chung JW, Kwak SY. Unentangled star-shape poly(epsilon-caprolactone)s as phthalate-free PVC plasticizers designed for non-toxicity and improved migration resistance. ACS Appl Mater Interfaces. 2014;6(14):11118–11128. doi: 10.1021/am500740v
- Navarro R, Perrino MP, Tardajos MG, et al. Phthalate plasticizers covalently bound to PVC: plasticization with suppressed migration. Macromolecules. 2010;43(5):2377–2381. doi: 10.1021/ma902740t
- Barroso EG, Duarte FM, Couto M, et al. A rheological study of the ageing of emulsion and microsuspension-based PVC plastisols. J Appl Polym Sci. 2008;109(1):664–673. doi: 10.1002/app.28173
- Howick C. New developments in emulsion-PVC polymerisation to produce polymers with the potential of reduced or zero VOC requirements when used in plastisol applications. Green Chem. 2007;9(3):243–246. doi: 10.1039/B610722B
- Thickett SC, Gilbert RG. Emulsion polymerization: state of the art in kinetics and mechanisms. Polymer. 2007;48(24):6965–6991. doi: 10.1016/j.polymer.2007.09.031
- Chern CS. Emulsion polymerization mechanisms and kinetics. Prog Polym Sci. 2006;31(5):443–486. doi: 10.1016/j.progpolymsci.2006.02.001
- Smith WV, Ewart RH. Kinetics of emulsion polymerization. J Chem Phys. 1948;16(6):592–599. doi: 10.1063/1.1746951
- Saeki Y, Emura T. Technical progresses for PVC production. Prog Polym Sci. 2002;27(10):2055–2131. doi: 10.1016/S0079-6700(02)00039-4
- Vidotto G, Crosato AA, Talamini G. Kinetic study of emulsion polymerization of vinyl chloride. Macromol Chem Phys. 1970;134(1):41–55. doi: 10.1002/macp.1970.021340104
- Ugelstad J, Mork PC, Dahl P, et al. A kinetic investigation of the emulsion polymerization of vinyl chloride. J Polym Sci Polym Symp. 1969;27(1):49–68. doi: 10.1002/polc.5070270106
- Klaus T, Gerhard R, Herbert G, et al. Modeling of emulsion polymerization of vinyl chloride. J Macromol Sci Chem. 1991;28(3–4):431–460. doi: 10.1080/00222339108052152
- Lin SY, Chern CS, Hsu TJ, et al. Emulsion polymerization of styrene: double emulsion effect. Polymer. 2001;42(4):1481–1491. doi: 10.1016/S0032-3861(00)00526-7
- Kobayashi C, Watanabe T, Murata K, et al. Localization of polystyrene particles on the surface of poly(N-isopropylacrylamide-co-methacrylic acid) microgels prepared by seeded emulsion polymerization of styrene. Langmuir. 2016;32(6):1429–1439. doi: 10.1021/acs.langmuir.5b03698
- Pusch J, Herk AM. Emulsion polymerization of transparent core-shell latices with a polydivinylbenzene styrene and vinyl acetate. Macromolecules. 2005;38(16):6909–6914. doi: 10.1021/ma050691p
- Zhu T, Kang WL, Yang HB, et al. Advances of microemulsion and its applications for improved oil recovery. Adv Colloid Interface Sci. 2022;299:102527. doi: 10.1016/j.cis.2021.102527
- Shibuya K, Nagao D, Ishii H, et al. Advanced soap-free emulsion polymerization for highly pure, micron-sized, monodisperse polymer particles. Polymer. 2014;55(2):535–539. doi: 10.1016/j.polymer.2013.12.039
- Kim G, Lim S, Lee BH, et al. Effect of homogeneity of methanol/water/monomer mixture on the mode of polymerization of MMA: soap-free emulsion polymerization versus dispersion polymerization. Polymer. 2010;51(5):1197–1205. doi: 10.1016/j.polymer.2009.12.038
- Endo K. Synthesis and structure of poly(vinyl chloride). Prog Polym Sci. 2002;27(10):2021–2054. doi: 10.1016/S0079-6700(02)00066-7
- Wang KT, He Y, Song XL, et al. Effects of the metakaolin-based geopolymer on high-temperature performances of geopolymer/PVC composite materials. Appl Clay Sci. 2015;114:586–592. doi: 10.1016/j.clay.2015.07.008
- Chen F, Ye FY, Chu GC, et al. Synthesis of acrylate modified vinyl chloride and vinyl isobutyl ether copolymers and their properties. Prog Org Coat. 2010;67(1):60–65. doi: 10.1016/j.porgcoat.2009.09.014
- Mobarakeh HS, Roudboneh MH. Study of vinyl acetate partitioning in emulsion copolymerization of vinyl chloride-vinyl acetate by FTIR and HNMR spectroscopy. J Polym Res. 2006;13(5):421–426. doi: 10.1007/s10965-006-9062-x
- Capek I, Mrázek Z. Emulsion copolymerization of vinyl chloride with butyl acrylate. Makromol Chem. 1992;193(5):1165–1179. doi: 10.1002/macp.1992.021930514
- Xiang HW, Fang HL, Pan CM, et al. Conversion of vinyl chloride-vinyl acetate-maleic anhydride emulsion copolymerization and characteristics of copolymer latexs. Chem React Eng Techn. 2014;30(6):522–527.
- Marvel CS, Schwen R. Some reactivity ratios of esters of acrylic acid. J Am Chem Soc. 1957;79(22):6003–6005. doi: 10.1021/ja01579a042
- Teyssié P, Smets G. Polymers and group interactions. II. Friedel-crafts reactions on polyvinyl chloride, a route to poly-1,3-methyleneindans 1. J Polym Sci. 1956;20(95):351–369. doi: 10.1002/pol.1956.120209510
- Chang YN, Pan MW, Yuan JF, et al. Morphology and film performance of phthalate-free plasticized poly(vinyl chloride) composite particles via the graft copolymerization of acrylate swelling flower-like latex particles. RSC Adv. 2015;5(50):40076–40087. doi: 10.1039/C5RA04747A
- Pan MW, Zhang LC, Wan LZ, et al. Preparation and characterization of composite resin by vinyl chloride grafted onto poly(BA-EHA)/poly(MMA-St). Polymer. 2003;44(23):7121–7129. doi: 10.1016/j.polymer.2003.08.022
- Pan MW, Xing SN, Yuan JF, et al. Synthesis and characterization of poly(butyl acrylate-co-ethylhexyl acrylate)/poly(vinyl chloride)[P(BA-EHA)/PVC] novel core-shell modifier and its impact modification for a poly(vinyl chloride)-based blend. Polym Eng Sci. 2010;50(6):1085–1094. doi: 10.1002/pen.21626
- Brown R, Stützel B, Sauer T. Steric stabilization by grafting and copolymerization of water-soluble oligomers and polymers. Macromol Chem Phys. 1995;196(6):2047–2064. doi: 10.1002/macp.1995.021960624
- Liu BC, Chen C, Li T, et al. High performance ultrafiltration membrane composed of PVDF blended with its derivative copolymer PVDF-g-PEGMA. J Membr Sci. 2013;445:66–75. doi: 10.1016/j.memsci.2013.05.043
- Shao XS, Li JH, Zhou Q, et al. Amphiphilic poly(vinyl chloride)-g-poly[poly(ethylene glycol) methylether methacrylate] copolymer for the surface hydrophilicity modification of poly(vinylidene fluoride) membrane. J Appl Polym Sci. 2013;129(5):2472–2478. doi: 10.1002/app.38891
- Abreu CM, Rezende TC, Serra AC, et al. Convenient and industrially viable internal plasticization of poly(vinyl chloride): copolymerization of vinyl chloride and commercial monomers. Polymer. 2023;267:125688. doi: 10.1016/j.polymer.2023.125688
- Ng YH, Lena FD, Chai CL. Metalloenzymatic radical polymerization using alkyl halides as initiators. Polym Chem. 2011;2(3):589–594. doi: 10.1039/C0PY00139B
- Henrı́quez C, Bueno C, Lissi EA, et al. Thiols as chain transfer agents in free radical polymerization in aqueous solution. Polymer. 2003;44(19):5559–5561. doi: 10.1016/S0032-3861(03)00581-0
- Valdebenito A, Encinas MV. Chain transfer agents in vinyl polymerizations photoinduced by bimolecular photoinitiators. J Photochem Photobio A. 2008;194(2–3):206–211. doi: 10.1016/j.jphotochem.2007.08.012
- Ahn SH, Seo JA, Kim JH, et al. Synthesis and gas permeation properties of amphiphilic graft copolymer membranes. J Membr Sci. 2009;345(1–2):128–133. doi: 10.1016/j.memsci.2009.08.037
- Gao G, Luo GZ, Xu MY, et al. Synthesis and characterization of multiple-crosslinkable polyacrylate emulsion for PVC film ink. Prog Org Coat. 2020;138:105190. doi: 10.1016/j.porgcoat.2019.06.036
- Moulay S. Chemical modification of poly(vinyl chloride)-still on the run. Prog Polym Sci. 2010;35(3):303–331. doi: 10.1016/j.progpolymsci.2009.12.001
- Simões PN, Coelho JF, Gonçalves PM, et al. Comparative non-isothermal kinetic analysis of thermal degradation of poly(vinyl chloride) prepared by living and conventional free radical polymerization methods. Eur Polym J. 2009;45(7):1949–1959. doi: 10.1016/j.eurpolymj.2009.04.021
- Zhu HM, Jiang XG, Yan JH, et al. TG-FTIR analysis of PVC thermal degradation and HCl removal. J Anal Appl Pyrolysis. 2008;82(1):1–9. doi: 10.1016/j.jaap.2007.11.011