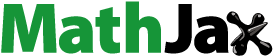
ABSTRACT
The thermoresponsive properties of poloxamine (tetra-branch PEO-PPO block copolymer) hydrogels are related to several variables. Of particular interest to this study were the molecular weight of the polymer, the molar ratio between PEO and PPO blocks, and the concentration of the aqueous solution. Accurately controlling the thermoresponsive behaviors of the polymer is critical to the application of such materials; therefore, the structure–property relationship of tetra-branch PEO-PPO block copolymer was studied by synthesis via anionic ring-opening polymerization (AROP). The structure–property relationships were studied by measuring the thermoresponsive behavior via differential scanning calorimetry (DSC) and developing an empirical model which statistically fit the collected data. This empirical model was then used for designing poloxamines that have critical micellization temperatures (CMT) between room temperature and physiological temperature. The model was validated with three polymers that targeted a CMT of 308 K (35°C). The empirical model showed great success in guiding the synthesis of poloxamines showing a temperature difference of less than 3 K between the predicted and the observed CMTs. This study showed a great potential of using an empirical model to set synthesis parameters to control the properties of the polymer products.
Introduction
The term ‘thermoresponsive polymers’ refers to a category of polymers that exhibit a sharp physical or chemical transition upon temperature change. Thermoresponsive polymers are favorable candidates for drug delivery, cell encapsulation, and tissue engineering [Citation1–3]. For example, a thermoresponsive polymer solution of a given concentration reaches a critical temperature, a phase transition occurs from a liquid solution to a gel [Citation4]. This property is useful for many thermally triggered medical applications due to the heating of the polymer solution once introduced to an ambient physiological temperature (the average internal human body temperature, 308 K, 37ºC) [Citation5].
Recently, thermoresponsive polymer hydrogels have been developed as tissue adhesives [Citation6–10]. The rapid physical gelation of these hydrogels provides a fast ‘hardening’ behavior which is characterized by an increase in both viscosity and strength when the hydrogels are introduced to a physiological environment with a sufficiently high temperature-one that is higher than both room temperature and the storage temperature. Given that this process is followed by a slower cross-linking reaction, such adhesives exhibit a ‘tandem’ gelation behavior which improves their ease of application and mechanical strength [Citation11].
Poly(ethylene oxide)-poly(propylene oxide)-poly(ethylene oxide) (PEO-PPO-PEO, poloxamer) block copolymers, also known as BASF Pluronic®, are widely studied thermoresponsive polymers of particular interest. The thermoresponsive properties and amphiphilic structure of these poloxamers make them good candidates for drug delivery [Citation12–14]. Unfortunately, this does not extend to the use of poloxamers as adhesives because the linear polymers can only undergo chemical crosslinking and cannot independently form the cross-linking network necessary for a tissue adhesive [Citation15–20]. Nagatomi and colleagues have developed a tissue adhesive using a tetra-branch PPO-PEO block copolymer (poloxamine, BASF Tetronic®) as the polymer matrix [Citation11,Citation21–23]. The four branches of the molecule allow for intermolecular crosslinking and tissue adhesion via chemically modified acrylate and N-hydroxysuccinimide ester moieties. Since both are amphiphilic PEO-PPO block copolymers, the physical properties of the star-shaped poloxamines are very similar to those of the linear poloxamers [Citation21].
The thermally triggered micellization and gelation behaviors of both poloxamers and poloxamines are well studied [Citation24–27]. Much like phase transition temperatures, the physical properties of the polymer hydrogels are related to various factors. These factors include the molecular weight of the polymer, the molar ratio of PEO to PPO repeat units, the concentration of the polymer in solution, and the pH of the mix. Therefore, a blend of polymers with different compositions may be used to change the properties of the hydrogel regardless of the environmental factors [Citation28]. This was proven in a previous study in which it was demonstrated that the gelation temperature of a hydrogel made from Tetronic® polymer T1107 may be modified by blending it with other Tetronic® polymers such as T304 and T904 [Citation21,Citation29]. In essence, the thermal behaviors of hydrogels were modified by using polymer blends to control the overall molecular weight, PEO to PPO molar ratio, and the polymer concentration. This alludes to the possibility that a single polymer of exact composition, rather than a polymer blend, could be made whose structure would directly result in targeted thermal properties. Ideally, a viable tissue adhesive stays liquid at room temperature and gels at the internal human body temperature. Therefore, there is a need to understand the structure–property relationships between molecular weight, the PEO to PPO molar ratio, and the polymer concentration and thermal properties. Unfortunately, the commercially available Tetronic® products provide an inadequate selection of polymer compositions, which is not ideal for an explicit study of the relationship between a polymer’s structure and its properties.
In the present study, we investigated this structure–property relationship to control the thermal gelation property without blending different polymers. A series of PPO-PEO block copolymers with customized structural parameters were synthesized via anionic ring-opening polymerization (AROP), which resembled the structure of Tetronic® polymers, as a means to determine the structural dependence of the phase transition temperatures. In a previous study, the effects of pH and concentration on the thermoresponsive properties of poloxamine T701 were studied [Citation30]. These properties were monitored via differential scanning calorimetry (DSC). Herein, we take advantage of fast DSC measurements to study how the change in polymer structure affected a polymer’s thermoresponsive properties. Since it has been shown in previous studies that there is a linear-like relationship between the polymer concentration and the phase transition temperature for each PEO-block-PPO copolymer [Citation25], an empirical model was built to fit the data obtained via DSC. The empirical model was then challenged through the synthesis of three targeted polymers that had a predicted phase transition at 308 K (35°C) with a 30% concentration. The results showed that the difference between the designed phase transition temperature and the measured transition temperature was less than 3 K, indicating the capability of using the empirical model to guide further syntheses of customized poloxamines.
Experimental
Materials for synthesis
Tetrahydrofuran (THF, BDH, 99%) was refluxed and stored under a dry nitrogen atmosphere in the presence of sodium and benzophenone, and the solution was kept purple indicating a lack of water. THF was distilled before use. Ethylene oxide (EO, Praxair, 99%), propylene oxide (PO, ACROS Organics, 99.5%), potassium metal (Aldrich,98%), and N,N,N′,N′-Tetrakis(2-hydroxyethyl)ethylenediamine (ACROS Organics, 99%) were used as received. All the flasks and the reaction vessel were dried in a drying oven at 200°C before use.
Polymerization procedures
As seen in , a series of nine block copolymers with three levels of molecular weights (three levels of monomer molar ratios for each molecular weight) were made to investigate the impact of structural parameters on their thermal properties. demonstrates the general synthetic route used in this study and the details are described below.
Table 1. Target structural parameters of the synthesis of tetra-branch poloxamines.
The anionic ring-opening polymerization was initiated by potassium salt of N,N,N’,N’-Tetrakis (2-hydroxyethyl)ethylenediamine. All reactions were done in a Parr Instrument Company series 4520 benchtop reactor with a mechanical stirrer under nitrogen. The nitrogen was desiccated before use. All gas lines and the reaction vessel were heated and purged with dry nitrogen to eliminate moisture before use. The synthesis procedure is as follows: first, 0.5 g (12.82 mmol) potassium metal was added directly into the reaction vessel, and the vessel was then purged with nitrogen and pulled with a vacuum (<0.1 psi). Next, 0.5 g (2.14 mmol) N,N,N’,N’-Tetrakis(2-hydroxyethyl)ethylenediamine was dissolved in a flask with 150 ml THF, and the flask was capped with a rubber stopper. The solution was purged by nitrogen and then transferred into the reaction vessel via a syringe. The reagents were mechanically stirred and heated at 100°C for 5 h.
The first monomer, propylene oxide, was injected into the reaction vessel at room temperature. The reaction was kept at 100°C for 24 h and the reactor was then cooled down to room temperature (22°C) with cooling water. The second monomer, ethylene oxide, was transferred and condensed from a gas cylinder to the reaction vessel by cooling the reactor to −40°C with liquid nitrogen. The flow rate and total mass of ethylene oxide were carefully monitored and controlled via an Aalborg-GFM17 gas flow meter/totalizer. After a 24-h reaction at room temperature, the product was then filtered and concentrated via a rotary evaporator followed by 24-h dialysis in deionized water (DI water) with Thermo Scientific-SnakeSkin® 3,500 MWCO dialysis tubing. The resulting solution was then freeze-dried using a Labconco® benchtop freeze dryer.
Characterization methods
The structures of the polymers were verified by 1 H nuclear magnetic resonance (NMR) spectroscopy. The 1 H NMR spectra were obtained using JEOL ECX-300 at 300 MHz, with CDCl3 (Cambridge Isotope Laboratories, Inc., 99.96%) as the solvent. The molecular weight and the degree of polymerization of each polymer sample were calculated from the corresponding peaks, and the PEO-PPO ratio was then calculated based on the degree of polymerization.
The DSC measurement of the polymers was carried out using a similar method as reported previously [Citation28,Citation31]. The thermal property of the polymers was measured by differential scanning calorimetry (DSC) using a TA-Q1000, and the results were analyzed via TA Universal Analysis software. Samples with different concentrations were made by mixing copolymer and DI water before the measurement. Briefly, 0.05 g copolymer samples were mixed with DI water to make the solutions at concentrations of 10 wt%,15 wt%, 20 wt%, 25 wt%, and 30%. The vials were then sonicated in an ice bath (0°C) for 30 min to dissolve the polymer. The solutions were kept in a fridge (4°C) overnight before measurement. Ten milligrams of each polymer solution was added into hermetic pans, respectively, and measured under constant helium flow (20 mL/min) at atmospheric pressure with a heating/cooling rate of 5°C/min from 0°C to 70°C.
Gel Permeation Chromatography (GPC) and rheology measurements were conducted at Eurofins EAG Laboratories (St. Louis, U.S.A.). GPC analysis was done on a Malvern OMNISEC system using Agilent PolarGel columns and N,N-dimethylformamide (DMF) with 0.02 M lithium bromide (LiBr) as mobile phase/solvent. The molecular weight distribution was calculated based on a calibration curve (Agilent PEG EasiVial standard). The samples were dissolved to 1 g/mL in the mobile phase twice, and each preparation was injected twice.
The rheology analysis was done with 30% solution of the synthesized sample. An oscillation temperature ramp at 5°C/min from 20°C to 60°C was used. Each sample was run three times to ensure reproducibility.
Results and discussion
Synthesis of tetra-branch poly (propylene oxide-block-ethylene oxide)
Given the emphasis on structural parameters such as molecular weight and ratio of repeat units, a living anionic polymerization was utilized. Using this method allowed for the controlled addition of the desired ratios of repeat units at separate stages at any given polymerization site, in this case, any given arm of a molecule of the starting material, via the careful balance of monomers with the initiator. Once synthesized, the products were highly viscous (see Table S1, supporting information) with samples becoming more similar to waxy solids at room temperature with increasing molecular weights and ratios of PEO to PPO. For this reason, Sample 6, Sample 8, and Sample 9 were near solids at room temperature.
1H NMR spectroscopy was performed to confirm the successful synthesis and determine the molecular weights (see Table S2, supporting information). The spectrum (see representative spectrum in Figure S1, supporting information) used for the analysis was normalized by the peaks which correspond to the di-amine core of each star polymer, δ = 2.5ppm-δ = 2.7ppm (-N-CH2-).
Measurement of thermoresponsive temperature with DSC
To show the polymer’s capability for reversible transition, a cyclic measurement was used as seen in . Exothermic and endothermic peaks were observed upon heating and cooling, respectively. At 5°C/min ramping rate, the peak width was about 15–20°C, while change in the concentration did not significantly affect the peak width. The peak temperatures of the heating and cooling curves will be influenced by the DSC heating and cooling rates. Therefore, using these two peaks, an average peak temperature was obtained for each sample as an unbiased transition temperature. This phase transition is due to the micellization of the polymer in an aqueous solution and the peak temperature represents the critical micellization temperature (CMT) at a certain concentration.
Figure 2. Representative DSC results of sample 5 at varying concentrations (Mw = 1.5 k, EO: PO = 3:2).
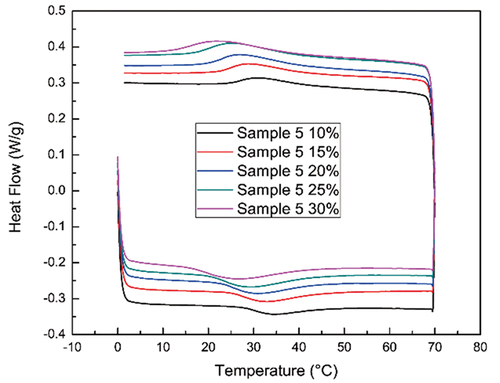
Previous studies have shown that CMT was an appropriate way to describe the phase transition of poloxamers (from aqueous solutions into hydrogels) and have also reported on the CMTs of poloxamer solutions [Citation30,Citation32]. Such studies helped illustrate the relationship between polymer structure and thermal properties. To observe this relationship for poloxamine samples, samples were synthesized and tested in two sets: one in which the number of PO units was an independent variable and another where the number of EO units was an independent variable. In both cases, the other repeat unit was kept constant as a control variable so that the effects of the amount of only one monomer on the CMT were observed.
All of these results lead to the understanding that the CMT is heavily dependent on the respective combination of the molecular weight, the molar ratio of degree of polymerization of each block (number of repeating units in each block), and the concentration of the polymer solution. Specifically, the peak temperature increases with decreasing molecular weight, increasing EO:PO ratio, and decreasing concentration of polymer in solution. Therefore, the thermal behavior of a given poloxamine sample can be altered with a fair amount of precision by adjusting all three of these parameters. In addition, since the molar ratio of the number of the repeating units and the molecular weight are intrinsically connected, these factors are not truly independent of each other. To better understand these relationships, an empirical model was developed to guide future syntheses in designing poloxamine solutions with targeted CMTs.
Statistical analysis and modeling
Recent studies have developed plausible mechanisms for the gelation of linear amphiphilic block copolymer hydrogels and attempted to explain this mechanism physically via modelling [Citation33,Citation34]. This strategy was adopted for these 4-armed systems, using a simple model followed by validation. Using previously known information about material properties and a comprehensive analysis of experimental data led to an equation which approximates the relationship between the peak temperature and the concentration percentage of poloxamine samples. This equation is as follows:
where is the expected absolute peak temperature in K and
is the concentration (as percent by weight). The slope (
) of the equation is dependent solely on the molar ratio (r), which may be interpreted to mean r of a specific sample may dictate the expected change of a given enthalpic peak per unit increase in concentration. The intercept term (
) in this equation is much more complex as it is dependent on both the number average molecular weight (
) and the molar ratio of the sample. Beyond this observation, the intercept term is quite difficult to interpret other than the fact that it centers the linear relationship where appropriate. For details concerning the data analysis which led to this model and the associated formula, please refer to the supplemental information.
Using experimental data gathered from several samples, the models for the intercept and slope are estimated as follows:
The empirical models and observed data are displayed in , where it may be observed that the data follows the estimated surface quite closely for the intercept term but is less precise for the slopes. This is a visual representation of how strongly gathered statistical evidence supports both terms – it shows strong support for the intercept term ( but only marginally supports the slope (
.
Figure 3. The estimated intercepts and slopes obtained from fitting the model Equationequation (1)(1)
(1) to the experimental data (left) along with an estimated regression line, also called an estimated regression surface, for describing the relationship between the intercepts, or slopes, and sample properties (right).
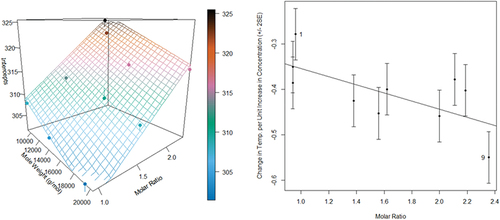
In light of the apparent discrepancy between the assumed model and statistical analysis, another approach was taken to ensure the validity of the model. Using the molecular weight and molar ratio of each sample as a baseline, the samples’ approximate relationship between their observed enthalpic peaks and the percent concentrations was derived and resulted in the plot presented in . This new plot shows a strong correlation between the data collected during experimentation and the assumed model.
Figure 4. Fitted regression lines relating average peak temperature to concentration percentage for each experimental sample.
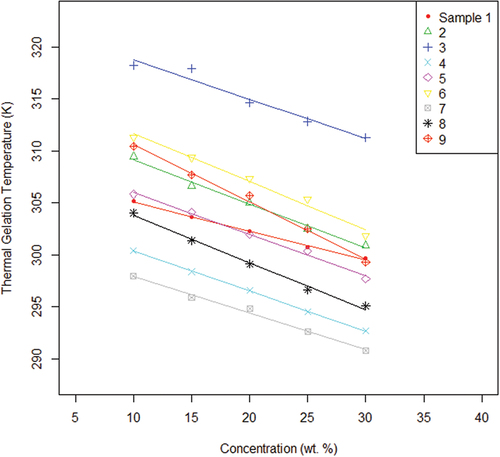
To further validate the empirical model, three new polymers were synthesized with different parameters to test three molecular weights, approximately 8000, 11000, and 16,000 g/mol, on the expected peak temperature of the polymer sample. Because the goal of this study is to make a polymer that is used in a physiological environment, the targeted transition temperature was designed at 308.15 K (35°C), below body temperature (37°C). The measured molecular parameters and the measured transition temperatures are shown in . shows a representative GPC chromatogram of sample TS-3 against a polyethylene glycol (linear PEG) standard calibration curve. The results showed that TS-3 has Mn = 10241 ± 155 g/mol and Mw = 14040 ± 277 g/mol (4 injections) which were close to the NMR results. The NMR estimated molecular weight was higher because the polydispersity index (PDI) was assumed as 1 in the calculation, however the PDI turned out to be 1.37 measured by GPC. shows a representative thermogram of the rheology measurement of TS-3. The results showed a max tan(delta) = 31.73 ± 0.75°C (304.88 ± 0.75 K) and a cross point = 36.47 ± 1.45°C (309.62 ± 1.45 K) (n = 3). The rheology results match very well with the DSC results, which confirms that the model successfully predicted the measured peak temperature within a 95% prediction interval (α = 0.05). More details were discussed in the supporting information.
Table 2. Structure parameters of test samples for model validation and the comparison between experimentally measured transition temperatures and model estimated transition temperatures.
Conclusions
The foremost purpose of this study was to investigate the influence of structural parameters, namely molecular weight, molar ratio of EO:PO, and solution concentration on the thermal property of four-arm poloxamines. In the course of this study, an empirical model was developed and validated which will prove invaluable for future syntheses of customized poloxamine with predictable target critical micellization temperatures. This newfound control on the thermal behavior of poloxamine will allow significant improvement in the application of poloxamine-based hydrogel adhesives in comparison to methods currently being used. From here, now that their thermal behavior is more easily controlled, the next logical step is to turn the synthesized tetra-branch poloxamines with designed structure to adhesives and sealants.
Disclosure statement
No potential conflict of interest was reported by the author(s).
Additional information
Funding
References
- Lai PL, Hong DW, Ku KL, et al. Novel thermosensitive hydrogels based on methoxy polyethylene glycol-co-poly(lactic acid-co-aromatic anhydride) for cefazolin delivery. Nanomed Nanotechnol Biol Med. 2014;10(3):553–560. doi: 10.1016/j.nano.2013.09.005
- Peppas NA, Bures P, Leobandung W, et al. Hydrogels in pharmaceutical formulations. Eur J Pharm Biopharm. 2000;50(1):27–46. doi: 10.1016/S0939-6411(00)00090-4
- Roy D, Brooks WLA, Sumerlin BS. New directions in thermoresponsive polymers. Chem Soc Rev. 2013;42:7214. doi: 10.1039/c3cs35499g
- Nagatomi J, Webb CK, Mefford OT, et al. Compliant surgical adhesive U.S. patent 9,283,298. 2016 Mar 15.
- Matanović MR, Kristl J, Grabnar PA. Thermoresponsive polymers: insights into decisive hydrogel characteristics, mechanisms of gelation, and promising biomedical applications. Int J Pharm. 2014;472(1–2):262–275. doi: 10.1016/j.ijpharm.2014.06.029
- Klouda L. Thermoresponsive hydrogels in biomedical applications a seven-year update. Eur J Pharm Biopharm. 2015;97:338–349. doi: 10.1016/j.ejpb.2015.05.017
- Chiappetta DA, Sosnik A. Poly(ethylene oxide)–poly(propylene oxide) block copolymer micelles as drug delivery agents: improved hydrosolubility, stability and bioavailability of drugs. Eur J Pharm Biopharm. 2007;66(3):303–317. doi: 10.1016/j.ejpb.2007.03.022
- Bromberg LE, Ron ES. Temperature-responsive gels and thermogelling polymer matrices for protein and peptide delivery. Adv Drug Deliv Rev. 1998;31(3):197–221. doi: 10.1016/S0169-409X(97)00121-X
- Burke SA, Ritter-Jones M, Lee BP, et al. Thermal gelation and tissue adhesion of biomimetic hydrogels. Biomed Mater. 2007;2(4):203–210. doi: 10.1088/1748-6041/2/4/001
- Bouten PJM, Zonjee M, Bender J, et al. The chemistry of tissue adhesive materials. Prog Polym Sci. 2014;39(7):1375–1405. doi: 10.1016/j.progpolymsci.2014.02.001
- Sanders L, Stone R, Webb K, et al. Society for biomaterials student award winner in the graduate degree category, 2015 annual meeting and exposition, charlotte, NC, April 15-18, 2015: mechanical characterization of a bifunctional Tetronic hydrogel adhesive for soft tissues. J Biomed Mater Res - Part A. 2015;103(3):861–868. doi: 10.1002/jbm.a.35310
- Chu C-C, Von Fraunhofer JA, Greisler HP. Wound closure biomaterials and devices. Boca Raton, FL: CRC Press; 1996.
- Toriumi DM, O’grady K, Desai D, et al. Use of octyl-2-cyanoacrylate for skin closure in facial plastic surgery. Plast Reconstr Surg. 1998;102(6):2209–2219. doi: 10.1097/00006534-199811000-00062
- Bot GM, Bot KG, Ogunranti JO, et al. The use of cyanoacrylate in surgical anastomosis: an alternative to microsurgery. J Surg Tech Case Rep. 2010;2(1):44–48. doi: 10.4103/2006-8808.63727
- Glickman M, Gheissari A, Money S, et al. A polymeric sealant inhibits anastomotic suture hole bleeding more rapidly than gelfoam/thrombin results of a randomized controlled trial. Arch Surg. 2002;137(3):326–331. doi: 10.1001/archsurg.137.3.326
- Foster K, Greenhalgh D, Gamelli RL, et al. Efficacy and safety of a fibrin sealant for adherence of autologous skin grafts to burn wounds: results of a phase 3 clinical study. J Burn Care Res. 2008;29(2):293–303. doi: 10.1097/BCR.0b013e31816673f8
- Küçükaksu DS, Akgül A, Çağli K, et al. Beneficial effect of BioGlue® surgical adhesive in repair of iatrogenic aortic dissection. Texas Hear Inst J. 2000;27:307.
- Kumar A, Maartens NF, Kaye AH. Evaluation of the use of BioGlue® in neurosurgical procedures. J Clin Neurosci. 2003;10(6):661–664. doi: 10.1016/S0967-5868(03)00163-2
- Spotnitz WD, Burks S. Hemostats, sealants, and adhesives: components of the surgical toolbox. Transfusion. 2008;48(7):1502–1516. doi: 10.1111/j.1537-2995.2008.01703.x
- Annabi N, Zhang Y.N., Assmann A. et al. Engineering a highly elastic human protein-based sealant for surgical applications. Sci Transl Med. 2017;9. doi: 10.1126/scitranslmed.aai7466
- Cho E, Lee JS, Webb K. Formulation and characterization of poloxamine-based hydrogels as tissue sealants. Acta Biomater. 2012;8(6):2223–2232. doi: 10.1016/j.actbio.2012.03.003
- Xinyue L, Khanna A, Luzinov I, et al. Surface modification of polypropylene surgical meshes for improving adhesion with poloxamine hydrogel adhesive. J Biomed Mater Res, Part B. 2019;107(4):1047–1055.
- Melinda H, Champaigne K, Cobb W, et al. A novel bio-adhesive mesh system for medical implant applications: In vivo assessment in a rabbit model. Gels. 2023;9(5):372.
- Derakhshandeh K, Fashi M, Seifoleslami S. Thermosensitive pluronic? hydrogel: prolonged injectable formulation for drug abuse. Drug Des Devel Ther. 2010;4:255–262. doi: 10.2147/DDDT.S13289
- Herzberger J, Niederer K, Pohlit H, et al. Polymerization of ethylene oxide, propylene oxide, and other alkylene oxides: synthesis, novel polymer architectures, and bioconjugation. Chem Rev Acs Chemrev. 2015;5b00441(4):2170–2243. doi: 10.1021/acs.chemrev.5b00441
- Alejos MF, Webb K, Mefford OT, et al. Controlling thermal gelation properties of novel tetronic hydrogel-based tissue adhesive. Conference Abstract: 10th World Biomaterials Congress; 2016. doi: 10.3389/conf.FBIOE.2016.01.00890
- Team RC. R: a language and environment for statistical computing; 2013.
- Yu G-E, Deng Y, Dalton S, et al. Micellisation and gelation of triblock copoly(oxyethylene/oxypropylene/oxyethylene), F127. J Chem Soc Faraday Trans. 1992;88(17):2537. doi: 10.1039/ft9928802537
- Alejos MF. Controlling thermal gelation properties of novel Tetronic® hydrogel-based tissue adhesive [ PhD diss]. Clemson University; 2016.
- Bromberg L. Properties of aqueous solutions and gels of poly (ethylene oxide) - b -poly (propylene oxide) - b -poly (ethylene oxide) - g -poly (acrylic acid). 1998;pp. 10736–10744. doi: 10.1021/jp983162e
- Lin HH, Cheng YL. In-situ thermoreversible gelation of block and star copolymers of poly(ethylene glycol) and poly(n-isopropylacrylamide) of varying architectures. Macromolecules. 2001;34(11):3710–3715. doi: 10.1021/ma001852m
- Alexandridis P, Holzwarth JF, Hatton TA. Micellization of poly(ethylene oxide)-poly(propylene oxide)-poly(ethylene oxide) triblock copolymers in aqueous solutions: thermodynamics of copolymer association. Macromolecules. 1994;27(9):2414–2425. doi: 10.1021/ma00087a009
- Gao X, Ji G, Peng T. The state equation of aggregation behaviours for poly (oxyethylene) - poly (oxypropylene) -poly (oxyethylene) tri-block copolymers in aqueous solution physica E: low-dimensional systems and nanostructures the state equation of aggregation behaviours for poly (oxyethylene) - poly (oxypropylene) -poly (oxyethylene) tri-block copolymers in aqueous solution. Phys E Low-Dimensional Syst Nanostruct. 2018;97:308–313.
- Cui S, Yu L, Ding J. Semi-bald micelles and corresponding percolated micelle networks of thermogels. Macromolecules. 2018;51(16):6405–6420. doi: 10.1021/acs.macromol.8b01014