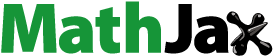
Abstract
This study compares and contrasts the desalination efficiency of tubular solar still using cylindrical and rectangular troughs when operating under a vacuum. For this, a two-dimensional code was created using the Comsol Multiphysics. The mass and heat transfer, inside the tubular solar still with the two different types of troughs, was simulated parametrically. The effect of the radius of the cylindrical trough, the square rib of the rectangular trough and the vertical elevation of the trough on heat and mass transfer were investigated at the same volume of seawater introduced initially. The numerical results revealed that the efficiency of the water productivity using cylindrical trough is significantly better than with rectangular trough when it is placed in the middle of the tubular shell. The opposite case of the 2 types of troughs is established when the trough advances towards the upper side of the glass cover where the condensation occurs.
Nomenclature
A | = | area, m2 |
Br | = | buoyancy ratio |
c | = | concentration, kg.m −3 |
C | = | dimensionless concentration |
D AB | = | mass diffusion coefficient, m2.s −1 |
di | = | diffusion volume, cm3.mol−1 |
g | = | acceleration of gravity, m.s−2 |
Lc | = | characteristic length (average distance between the evaporation and condensation surfaces), m |
Le | = | Lewis number |
Lw | = | width of water surface, m |
M | = | molar mass, kg/mol |
mhourly | = | distillation rate, kg.m−2.h−1 |
Nu | = | Nusselt number |
P | = | dimensionless pressure |
P | = | pressure, kPa |
pop | = | operating pressure, kPa |
Pr | = | Prandtl number |
R | = | radius of the outer tube (tubular shell), m |
r | = | radius of the cylindrical trough, m |
RaT | = | thermal Rayleigh number |
SR | = | square rib |
T | = | temperature, °C |
t | = | time, s |
U, V | = | dimensionless velocity components |
u, v | = | velocity components in x, y directions, m.s−1 |
x, y | = | coordinate directions, m |
X, Y | = | dimensionless coordinate directions |
Greek symbols
α | = | thermal diffusivity, m2.s−1 |
βc | = | coefficient of concentration expansion, m3.kg−1 |
βT | = | coefficient of thermal expansion, K−1 |
θ | = | Dimensionless temperature |
λ | = | thermal conductivity, W.m−1.K−1 |
ν | = | kinematic viscosity, m2.s−1 |
ρ | = | density, kg.m−3 |
τ | = | dimensionless time |
Subscripts
A | = | component A in a binary diffusion system |
a | = | air |
w | = | water |
1. Introduction
There is no way to exaggerate the importance of supplying fresh water. Lack of access to clean drinking water has impacted many people, particularly in rural and arid locations. If these areas have a lot of solar energy, solar distillation might be a good solution for the paucity of water sources in these areas. In dry areas with plenty of renewable energy sources, sunlight has actually been considered one of the finest ways to power the desalination process. Solar stills are still the most popular solar desalination method in rural areas because of their simplicity in construction and maintenance [Citation1–5].
The most common cover designs for solar stills are tubular [Citation6], triangular [Citation7], single-slope [Citation8] and double-slope [Citation9]. Despite numerous improvements in solar technology, their low productivity continues to be the principal obstacle to their widespread usage. As a result, more up-to-date solar stills are being developed, such as multi-stage systems [Citation10] and outside reflectors [Citation11], to maximize the output of freshwater.
The tubular solar still is a conventional method with a cylindrical shape that employs the same distillation process as conventional basin solar still. The use of tubular solar stills for solar distillation has attracted a lot of interest in practical and theoretical investigations [Citation12,Citation13].
Kumar et al. [Citation14] found that the evacuated tubular collector is generally used to achieve higher collector efficiency. A higher rate of vapourization is achieved by integrating many series-connected evacuated tubes with solar still. The overall performance of the system depends on the heat transfer rate from the absorber tube to the working fluid. Heat transfer rate can further be improved by adding various sizes of nanoparticles to working fluid and acting as a heat-absorbing device during less sunshine or cloudy days.
Raturia et al. [Citation15] found that the increase in the number of collectors leads to a raise in the values of freshwater yielding as well as daily exergy gain maintaining other parameters at the input of the system as constant.
Singh et al. [Citation16] conclude that the collector’s numbers lead to an increase in the values of both the potable water (PW) and electric power output (EPO). During design and installation, one needs to maintain the water temperature below the boiling point. So, a compromise between PW output and EPO is to be made while selecting the values.
Singh et al. [Citation17] found the proposed system has been compared with N-PVT-CPC-SS and CSSSS for the same basin area under similar climatic conditions. The yearly distillate output, thermal exergy, electrical exergy and UAC are the highest for N-FPCP-SS. However, the productivity of the proposed system is higher than that of the other two systems.
An analysis of Singh [Citation18] has been done at 0.14 m water depth. It has been concluded that kWh per unit cost based on exergy gain is higher by 40.73 and 54.63%; environmental cost is higher by 59.70 and 92.55%; however, the output per unit input based on productivity is higher by 9.25% and lower by 25.13% for N-PVT-FPC-DS than double slope solar still integrated with N identical PVT compound parabolic concentrator collector and conventional double slope solar still, respectively.
Dhivagar et al. [Citation19] conducted experimental and numerical studies and found the heat accumulated in crushed gravel sand and biomass evaporator has been used to preheat the inlet saline water and air vapour before entering into the solar still. This results in enhanced air vapour mixture temperature and evaporative heat transfer rate. The productivity, energy and exergy efficiencies of CGS-BSS were improved by 34.6%, 34.4%, and 35%; respectively.
The proposed still of Balachandran et al. [Citation20] produces a maximum yield of 0.98 L during 01:00pm, while the CSS produces only 0.57 L. The distillate yield of the PSS was 3.05 L/day, whereas the CSS produced 2.47 L per day. The thermal efficiency of the still reaches its maximum in the noon time between 12-00pm and 13-00pm, and the peak value attained is 65.46%.
Sharma et al. [Citation21] found the values of correlation coefficients for condensing glass temperature, water temperature and water yield and evaluated the energy metrics, productivity, cost of producing 1 kg of fresh water and exergoeconomic and enviroeconomic parameters.
Other results are found based on experimental theoretical work [Citation22–26]. It has been the goal of several earlier studies to increase the efficiency of solar stills, either by enhancing the construction of solar stills [Citation27–39] or by integrating materials for heat storage [Citation40–46]. Running solar stills in a vacuum [Citation47–49] is another option for enhancing their effectiveness.
Although some research has been done on the situation of tubular solar still operating with a rectangular trough under vacuum conditions [Citation10,Citation50,Citation51], the effects of the trough’s position and dimensions modifying inside the tubular shell have not yet been investigated. This study specifically uses both a rectangular trough and a cylindrical trough to achieve this goal. The efficiency of water productivity between the two types of troughs is also investigated.
2. Presentation of physical problem
2.1. Presentation and description of the studied tubular solar still
The design of a tubular solar still is shown in Figure . It includes a cylindrical and rectangular trough that contains seawater at a temperature and concentration of Tw and Cw. The temperature of the cover, which is always lower than the temperature of the water, is Ts. The cross-section of the trough is located in the centre of the cylindrical tubular shell.
The enclosure is emptied. The vacuum in the chamber is maintained, while the chamber is operating, using an external vacuum pump. The trough’s liquid keeps evaporating as solar energy is absorbed. Due to buoyancy forces, water that is in a vapor condition rises to the water’s surface and condenses on the top shell’s inner surface.
Gravity causes the condensate to drip down the interior shell surface of the still and collect at the bottom, where fresh water is obtained.
2.2. Simplifying assumptions
The following assumptions [Citation51] about the operating parameters of tubular solar still are established to study the thermal and flow properties of this two-dimensional model in an unsteady state.
The fluid is a combination of water vapour and air that is incompressible and Newtonian.
There is a two-dimensional, laminar flow of fluid.
The temperatures of the water and cover are constant.
The trough wall is adiabatic.
Heat transfer by radiation and viscous dissipation is negligible.
2.3. Governing equations
The following are the unsteady-state controlling equations [Citation51] for mass, momentum, concentration and energy conservation:
Equation of Continuity:
(1)
(1) The non-dimensional velocity components according to the X and Y axis are U and V, respectively.
The X-axis momentum equation:
(2)
(2) Pr is the Prandtl number.
and P are respectively the dimensionless time and pressure.
The Y-axis momentum equation:
(3)
(3) where RaT is the thermal Rayleigh number. Br is the buoyancy ratio.
and C are the dimensionless temperature and concentration, respectively.
Energy equation:
(4)
(4) Concentration equation:
(5)
(5) Le is the Lewis number.
The dimensionless variables are defined as follows in the previous equations:
(6)
(6) The characteristic length Lc is the average length between the edge of the water and the shells interior surface.
The coefficient for thermal expansion is defined as [Citation52]
(7)
(7) The coefficient for concentration expansion is defined as [Citation53]
(8)
(8)
The mass diffusion coefficient was determined using the following formula [Citation54]:
(9)
(9) For water and air, the volume of diffusion di values used was 12.7 and 20.1 cm3/mol, respectively [Citation51].
The rate at which fresh water is produced, also known as productivity was determined using the following equation [Citation51]:
(10)
(10) The mean Nusselt number
on the water surface was determined as follows [Citation51]:
(11)
(11) Lw is the width of the water surface.
2.4. Boundary conditions
The boundary conditions of our model are illustrated in Table .
Table 1. Boundary conditions for non-dimensional form.
3. Results and discussion
In this part and after the validation of our code, our numerical model is utilized to investigate how the rib, radius and vertical elevation of both the rectangular and cylindrical trough affect heat and mass transmission inside the tubular solar still, particularly concerning the productivity of fresh water.
3.1. Numerical method and validation
Using Comsol Multiphysics 5.5, the flow structure within the tubular solar still was modelled. It was decided to use a second-order upwind discretization technique. The species transport model for the air-vapour mixture was implemented in this simulation.
The density was changed to reflect that of an ideal, incompressible fluid. The mixing law was applied to calculate the specific heat capacity. The mass-weighted mixing equation was used to determine the viscosity and thermal conductivity.
The solution was deemed convergent when the scaled residuals for the energy equation were less than 10−6 and 10−4 for the others.
On 26 June 2016, in Ha'il, Saudi Arabia, Elashamwy [Citation55] conducted experimental work that is utilized to validate this model. The evolution of the water productivity is numerically determined for each hour of this day by our code, which is shown in Figure , based on the temporary temperature conditions of the trough, inside tube surface, and ambient. With the help of this graph, we can observe how well our code’s numerical findings and Elashamawy’s experimental findings [Citation55] agree on several key points. So, the mesh used is extremely fine.
3.2. Effect of the geometry of rectangular and cylindrical trough
We examine the impact of the radius of the cylindrical trough on heat and mass transport inside the tubular solar still in this section. To do this, we change this radius from 20 to 50 mm in the centre of the tubular shell while maintaining the same operating pressure, glass cover temperature and beginning seawater temperature, as shown in Table .
Table 2. Initial parameters of tubular solar still.
Figure displays the velocity contours for several radii of the cylindrical trough (0.02 m; 0.03 m; 0.04 mm and 0.05 m). This figure shows that the velocity reduces as the radius of the trough increases. In fact, the greatest velocity for a radius of 0.02 mm is 0.40 m/s. However, the velocity does not surpass 0.23 m/s in the case of the radius of the trough of 0.05 m. The highest velocities are 0.40, 0.35, 0.29 and 0.23 m/s, which are equivalent to the radius of the cylindrical trough of 0.02 m; 0.03 m; 0.04 mm and 0.05 m, respectively.
Figure 3. Contours of vorticity (left), velocity (middle) and temperature (right) for various radii of the cylindrical trough at Tw = 60°C and Pop = 101 kpa. (a) −20 mm; (b) −30 mm; (c) −40 mm; (d) −50 mm.

The vorticity contours for various trough radiuses (20 mm; 30 mm; 40 and 50 mm) are shown in Figure . This image shows that there are two cells sandwiched between the cylindrical troughs. Additionally, as the radius of the cylindrical trough grows, there is a notable increase in vorticity between the upper rib of the square and the upper tubular shell.
The temperature contours for various trough radiuses (20; 30; 40 and 50 mm) are shown in Figure . It is evident from the figure that the hot zone is restricted to the area between the upper face of the cylindrical trough and the upper surface of the tubular shell. This is a result of the seawater evaporation phase. The lower part is cold because that is where condensed water is recovered after cooling.
To compare the effectiveness of the two types of cylindrical and rectangular troughs, we took the same volume of seawater corresponding to the studied radii (0.02 m; 0.03 m; 0.04 mm and 0.05 m) and we deduced the corresponding dimensions of the square trough, which will be 0.026; 0.038; 0.051 and 0.063 m.
Figure shows the contours of vorticity, velocity and temperature for various ribs (26; 38; 51 and 63 mm) of the rectangular trough at Tw = 60°C and Pop = 101 kpa.
Figure 4. Contours of vorticity (left), velocity (middle) and temperature (right) for various ribs of the rectangular trough at Tw = 60°C and Pop = 101 kpa. (a) −26 mm; (b) −38 mm; (c) −51 mm; (d) −63 mm.

According to this figure, we see the same physical phenomenon as in the case of a cylindrical trough while it is very remarkable that the maximum velocity is lower than the case of the cylindrical trough of the same seawater volume.
To examine the impact of distillate rate, or the productivity of distillate water from the tubular solar still with both cylindrical and rectangular troughs, we plot the distillate rate according to each radius (20; 30; 40 and 50 mm) and each square rib of the trough (26; 38; 51 and 63 mm) at Tw = 60°C and Pop = 101 kpa, as shown in Figure . We can see from this graph that when the radius and rib of the two types of troughs are increased, so does the production of distillate water.
Figure 5. Evolution of the Water Productivity according to square rib/Radius of the trough at Tw = 60°C and Pop = 101 kpa.

Indeed, the distillate rate is 0.38122 kg/(m2*h) for the case of the radius of the cylindrical trough is 20 mm. This quantity increases by 5.77; 18.96; 46.47% when the radius of the cylindrical trough is 0.030; 0.04 and 0.05 m, respectively.
For the case of the rectangular trough, the productivity is 0.23432 kg/(m2*h) when the rib square trough is 26 mm. This amount of fresh water increases by 20.77; 46.65; 82.67% when the rib of the square trough becomes 0.038; 0.051 and 0.063 m, respectively.
In addition, we deduce that the water productivity of the cylindrical trough is better than the rectangular trough case for the same volume of seawater from the start. Indeed, the efficiency of the water productivity in the cylindrical case is between 30.4 and 62.69% when the trough is placed in the middle of the tubular shell.
3.3. Effect of the vertical elevation of the rectangular and cylindrical trough
This section looks into the effects of vertical elevation on heat and mass transfer in cylindrical and rectangular troughs inside a tubular solar still. To maintain consistent working pressure, glass cover temperature and beginning seawater temperature, we move them through the position of the centre of the tubular shell from 5 to 30 mm vertically and upwards.
Figures and show the contours of the velocity of four various radii of the cylindrical trough (r = 0.02; 0.03; 0.04 and 0.05 m) and four square ribs of the rectangular trough (SR = 0.026; 0.038; 0.051 and 0.063 m) at Tw = 60°C and Pop = 101 kPa for different vertical elevation of the trough (5–30 mm with a gap of 5 mm).
Figure 6. Contours of the velocity of four various radii of the cylindrical trough (r = 0.02; 0.03; 0.04 and 0.05 m) at Tw = 60°C and Pop = 101 kpa for different vertical elevations of the trough (5–30 mm with a gap of 5 mm).

Figure 7. Contours of the velocity of four various square ribs of the rectangular trough (SR = 0.026; 0.038; 0.051 and 0.063 m) at Tw = 60°C and Pop = 101 kpa for different vertical elevations of the trough (5–30 mm with a gap of 5 mm).

These 2 figures show that the velocity reduces when the two types of troughs are elevated from the tubular shell'’s centre to its upper surface.
Indeed, when the cylindrical trough is in the centre of the tubular trough and its radius is 0.05 m, the maximum velocity is 0.23 m/s while it is 0.14 m/s for the same volume corresponding to a rectangular trough.
However, when it is raised to the upper surface of 5; 10; 15; 20; 25 and 30 mm, the maximum velocity drops by 8.7; 21.47; 34.78; 47.83; 56.52 and 65.22% for the case of the cylindrical trough, respectively. It dropped by 21.43; 35.71; 50; 67.14; 78.57 and 87.86% in rectangular trough case.
We conclude that the increase of the velocity in the case of rectangular is more significant than that for the cylindrical trough. Therefore, the trough geometry is a determining factor for the velocity and the heat transfer effect inside the tubular shell.
Figures and show the contours of vorticity of four various radii of the cylindrical trough (r = 0.02; 0.03; 0.04 and 0.05 m) and four square ribs of the rectangular trough (SR = 0.026; 0.038; 0.051 and 0.063 m) at Tw = 60°C and Pop = 101 kPa for different vertical elevations of the trough (5–30 mm with a gap of 5 mm).
Figure 8. Contours of vorticity of four various radii of the cylindrical trough (r = 0.02; 0.03; 0.04 and 0.05 m) at Tw = 60°C and Pop = 101 kpa for different vertical elevations of the trough (5–30 mm with a gap of 5 mm).

These figures show that there are two cells situated in between the rectangular and cylindrical troughs. The vorticity between the upper rib of the square/circular trough and the upper tubular shell both greatly increases when the two types of troughs travel up and reduce in the lower region.
Figures and show the contours of the temperature of four various radii of the cylindrical trough (r = 0.02; 0.03; 0.04 and 0.05 m) and four square ribs of the rectangular trough (SR = 0.026; 0.038; 0.051 and 0.063 m) at Tw = 60°C and Pop = 101 kPa for different vertical elevation of the trough (5–30 mm with a gap of 5 mm).
Figure 9. Contours of vorticity of four various square ribs of the rectangular trough (SR = 0.026; 0.038; 0.051 and 0.063 m) at Tw = 60°C and Pop = 101 kpa for different vertical elevations of the trough (5–30 mm with a gap of 5 mm).

Figure 10. Contours of Temperature of four various radii of the cylindrical trough (r = 0.02; 0.03; 0.04 and 0.05 m) at Tw = 60°C and Pop = 101 kpa for different vertical elevations of the trough (5–30 mm with a gap of 5 mm).

In these figures, there is a distinct hot zone between the top of the rectangular and cylindrical troughs and the top of the tubular shell. The seawater’s evaporation is to blame for this because purified water is recovered after condensation in the bottom section, it is cold Figure .
Figure 11. Contours of Temperature of four various square ribs of the rectangular trough (SR = 0.026; 0.038; 0.051 and 0.063 m) at Tw = 60°C and Pop = 101 kpa for different vertical elevations of the trough (5–30 mm with a gap of 5 mm).

To study the effect of the productivity of fresh water of the tubular solar still with both rectangular and cylindrical troughs, we plot the distillate rate for each type of trough according to the vertical elevation of the trough (5–30 mm with a gap of 5 mm) at Tw = 60°C and Pop = 101 kpa shown in Figures and , respectively. From these figures, we see that the productivity of distillate water rises with the increase of the vertical elevation of the trough in the two cases. This can be understood by the fact that as the trough dimension grows, the space between the hot and cold zones closes, allowing for more substantial convective transfer. In addition, when the rectangular trough is vertically offset from the centre by 30 mm; water production improves much better than for the cylindrical trough case. Indeed, the efficiency of production water for a rectangular trough of dimensions 0.026; 0.038; 0.051 and 0.063 m is respectively: 17.42, 30.31, 53.22 and 105.82%. Whereas for the case of the cylindrical trough and the same conditions, these percentages are 12.43, 17.14, 22.05 and 27.12%.
Figure 12. Evolution of Water productivity according to the vertical elevation of the trough for different radii of the cylindrical trough at Tw = 60°C and Pop = 101 kpa.

Figure 13. Evolution of Water productivity according to the vertical elevation of the trough for the different square ribs of the rectangular trough at Tw = 60°C and Pop = 101 kpa.

We conclude that the vertical elevation of the trough is a crucial factor in improving distillate water productivity. It is much more significant in the case where the trough is rectangular.
4. Conclusion
The purpose of this research is to study the tubular solar still with a rectangular trough by comparing it with the cylindrical trough. After the validation of the code with the experimental results using Comsol Multiphysics, it’s used to examine the effect of the dimensions and the vertical elevation of the two types of the trough on water productivity.
The significant findings are summarized as follows:
✓ The velocity reduces when the two types of troughs are elevated from the tubular shell’s centre to its upper surface.
✓ The productivity of distillate water is significantly increased with the increase of the dimensions of the 2 types of trough situated in the middle of the tubular shell.
✓ The productivity of distillate water increases as the vertical elevation of the trough moves towards the upper surface.
✓ The enhancement of the water productivity in the tubular solar still with rectangular trough improves much better than with the cylindrical trough.
Disclosure statement
No potential conflict of interest was reported by the author(s).
References
- Singh DB. Sensitivity analysis of N identical evacuated tubular collectors integrated double slope solar distiller unit by incorporating the effect of exergy. Int J Exergy. 2021;34-4:424–447.
- Boukhriss M, Khemili S, Ben Hamida MB, et al. Simulation and experimental study of an AGMD membrane distillation pilot for the desalination of seawater or brackish water with zero liquid discharged. Heat Mass Transfer. 2018;54:3521–3531.
- Boukhriss M, Ben Hamida MB, Maatoug MA, et al. The design of a unit sweeping gas membrane distillation: experimental study on a membrane and operating parameters. Appl Water Sci. 2020;10:110.
- Jrad A, Ben Hamida MB, Ghnay R, et al. Contribution to the study of combined adsorption–ejection system using solar energy. Adv Mech Eng. 2017;9(7):1–9.
- Ben Hamida MB, Belghaieb J, Hajji N. Numerical study of heat and mass transfer enhancement for bubble absorption process of ammonia-water mixture without and with nanofluids. Therm Sci. 2018;22:3107–3120.
- Xie G, Sun L, Mo Z, et al. Conceptual design and experimental investigation involving a modular desalination system composed of arrayed tubular solar stills. Appl Energy. 2016;179:972–984.
- Ahsan A, Imteaz M, Thomas UA, et al. Parameters affecting the performance of a low cost solar still. Appl Energy. 2014;114:924–930.
- Rashidi S, Bovand M, Esfahani JA. Optimization of partitioning inside a single slope solar still for performance improvement. Desalination. 2016;395:79–91.
- Rahbar N, Gharaiian A, Rashidi S. Exergy and economic analysis for a double slope solar still equipped by thermoelectric heating modules - an experimental investigation. Desalination. 2017;420:106–113.
- Xie G, Chen W, Yan T, et al. Three-effect tubular solar desalination system with vacuum operation under actual weather conditions. Energy Convers Manage 2020;205:112371.
- Huang CH, Chang TR. Determination of optimal inclination function for external reflector of basin type still for maximum distillate productivity. Energy. 2017;141:1728–1736.
- Nebbia G. An experiment with plastic tubular solar still, Symposium on Saline Water Conversion, Washington, D. C., Proceedings; 1957, p. 175–176 (illus.).
- Tleimat BW, Howe ED. Nocturnal production of solar distillers, Solar Energy Conference, Phoenix, Arizona, 1965 Mar 15–17. vol. l0 (2), 1966, p. 61–66.
- Kumar R, Singh DB, Dewangan A, et al. Performance of evacuated tube solar collector integrated solar desalination unit — a review. Desalin Water Treat. 2021;230:92–115.
- Raturia A, Singh DB, Patil PP, et al. Sensitivity analysis of a solar still of a single slope type included with N similar evacuated tubular collectors having series connection. Desalin Water Treat. 2021;234:309–323.
- Singh DB, Bansal G, Prasad H, et al. Sensitivity analysis of N undistinguishable photovoltaic thermal compound-parabolic-concentrator collectors (partly covered, 50%) integrated single-slope solar distiller unit. J SolEnergy Eng. 2021;134:021003–021001.
- Singh DB, Kumar N, Kumar S, et al. Enhancement in exergoeconomic and enviroeconomic parameters for single slope solar still by incorporating N identical partially covered pvt collectors. J Sol Energy Eng. 2017;17:1198.
- Singh DB. Exergoeconomic and enviroeconomic analyses of N identical photovoltaic thermal integrated double slope solar still. Int J Exergy. 2017;23-3:347–362.
- Dhivagar R, Mohanra M, Belyaye Y. Performance analysis of crushed gravel sand heat storage and biomass evaporator-assisted single slope solar still. Environ Sci Pollut Res. 2021;28:65610–65620.
- Balachandran GB, David PW, Alexander AB, et al. A relative study on energy and exergy analysis between conventional single slope and novel stepped absorbable plate solar stills. Environ Sci Pollut Res. 2021;28:57602–57618.
- Sharma K, Mallick A, Singh DB, et al. Experimental study of solar energy–based water purifier of single-slope type by incorporating a number of similar evacuated tubular collectors. Environ Sci Pollut Res. 2022;29:6837–6856.
- Singh D, Yadav R, Chaturbedi Y, et al. Study on effect of dissimilarity of mass flow rate on energy metrics of solar energy based double slope water purifier by incorporating N alike PVT compound parabolic concentrator collectors. Desalin Water Treat. 2021;231:27–43.
- Kabeel AE, Sharshir SW, Abdelaziz GB, et al. Improving performance of tubular solar still by controlling the water depth and cover cooling. J Clean Prod. 2019;233:848–856.
- Elshamy SM, El-Said EMS. Comparative study based on thermal, exergetic and economic analyses of a tubular solar still with semi-circular corrugated absorber. J Clean Prod. 2018;195:328–339.
- Goshayeshi HR, Safaei MR. Effect of absorber plate surface shape and glass cover inclination angle on the performance of a passive solar still. Int J Numer Methods Heat Fluid Flow. 2019;30(6):3183–3198.
- Arunkumar T, Velraj R, Denkenberger DC, et al. Productivity enhancements of compound parabolic concentrator tubular solar stills. Renew Energy. 2016;88:391–400.
- Sathyamurthy R, El-Agouz SA, Nagarajan PK, et al. A review of integrating solar collectors to solar still. Renewable Sustainable Energy Rev. 2017;77:1069–1097.
- Omara ZM, Kabeel AE, Abdullah AS. A review of solar still performance with reflectors. Renewable Sustainable Energy Rev. 2017;68:638–649.
- Alaian WM, Elnegiry EA, Hamed AM. Experimental investigation on the performance of solar still augmented with pin-finned wick. Desalination. 2016;379:10–15.
- Rajaseenivasan T, Srithar K. Performance investigation on solar still with circular and square fins in basin with CO2 mitigation and economic analysis. Desalination. 2016;380:66–74.
- Malaeb L, Aboughali K, Ayoub GM. Modeling of a modified solar still system with enhanced productivity. Sol Energy. 2016;125:360–372.
- Rabhi K, Nciri R, Nasri F, et al. Experimental performance analysis of a modified single-basin single-slope solar still with pin fins absorber and condenser. Desalination. 2017;416:86–93.
- Fathy M, Hassan H, Ahmed MS. Experimental study on the effect of coupling parabolic trough collector with double slope solar still on its performance. Sol Energy. 2018;163:54–61.
- El-Maghlany WM, Abdelaziz AH, Hanafy AA, et al. Effect of continuous and discrete makeup water on the productivity of conventional solar still. J Energy Storage. 2020;28:101223.
- Abdullah AS, Younes MM, Omara ZM, et al. New design of trays solar still with enhanced evaporation methods – comprehensive study. Sol Energy. 2020;203:164–174.
- Selvaraj K, Natarajan A. Factors influencing the performance and productivity of solar stills - a review. Desalination. 2018;435:181–187.
- Kabeel AE, Abdelgaied M. Performance enhancement of modified solar still using multi-groups of two coaxial pipes in basin. Appl Therm Eng. 2017;118:23–32.
- Rahbar N, Asadi A, Fotouhi-Bafghi E. Performance evaluation of two solar stills of different geometries: tubular versus triangular: experimental study, numerical simulation, and second law analysis. Desalination. 2018;443:44–55.
- Arani RP, Sathyamurthy R, Chamkha A, et al. Effect of fins and silicon dioxide nanoparticle black paint on the absorber plate for augmenting yield from tubular solar still. Environ Sci Pollution Res. 2021;28:35102–35112. doi:10.1007/s11356-021-13126-y
- Mousa H, Naser J, Gujarathi AM, et al. Experimental study and analysis of solar still desalination using phase change materials. J Energy Storage. 2019;26:100959.
- Abu-Arabi M, Al-harahsheh M, Ahmed M, et al. Theoretical modeling of a glass-cooled solar still incorporating PCM and coupled to flat plate solar collector. J Energy Storage. 2020;29:101372.
- Vigneswaran VS, Kumaresan G, Dinakar BV, et al. Augmenting the productivity of solar still using multiple PCMs as heat energy storage. J Energy Storage. 2019;26:101019.
- Kabeel AE, Teamah MA, Abdelgaied M, et al. Modified pyramid solar still with v-corrugated absorber plate and PCM as a thermal storage medium. J Clean Prod. 2017;161:881–887.
- Dumka P, Sharma A, Kushwah Y, et al. Performance evaluation of single slope solar still augmented with sand-filled cotton bags. J Energy Storage. 2019;25:100888.
- Voropoulos K, Mathioulakis E, Belessiotis V. Solar stills coupled with solar collectors and storage tank—analytical simulation and experimental validation of energy behavior. Sol Energy. 2003;75(3):199–205.
- Kabeel AE, Abdelgaied M, Eisa A. Effect of graphite mass concentrations in a mixture of graphite nanoparticles and paraffin wax as hybrid storage materials on performances of solar still. Renew Energy. 2019;132:119–128.
- Ahmed MI, Hrairi M, Ismail AF. On the characteristics of multistage evacuated solar distillation. Renew Energy. 2009;34:1471–1478.
- Gude VG, Nirmalakhandan N, Deng S, et al. Feasibility study of a new two-stage low temperature desalination process. Energy Convers Manage. 2012;56:192–198.
- Zheng H, Chang Z, Chen Z, et al. Experimental investigation and performance analysis on a group of multi-effect tubular solar desalination devices. Desalination. 2013;311:62–68.
- Xie G, Sun L, Yan T, et al. Model development and experimental verification for tubular solar still operating under vacuum condition. Energy. 2018;157:115–130.
- Yan T, Xie G, Liu H, et al. CFD investigation of vapor transportation in a tubular solar still operating under vacuum. Int J Heat Mass Transfer. 2020;156:119917.
- Ben Jaballah R, Ben Hamida MB, Almeshaal MA, et al. The influence of hybrid nanofluid and coolant flow direction on bubble mode absorption improvement. Math Methods Appl Sci. 2020;1–15. doi:10.1002/mma.6605.
- Talukdar P, Iskra CR, Simonson CJ. Combined heat and mass transfer for laminar flow of moist air in a 3D rectangular duct: CFD simulation and validation with experimental data. Int J Heat Mass Transf. 2008;51:3091–3102.
- Ben Hamida MB, Belghaieb J, Hajji N. Heat and mass transfer enhancement for falling film absorption process in vertical plate absorber by adding copper nanoparticles. Arab J Sci Eng. 2018;43(9):4991–5001.
- Elashmawy M. An experimental investigation of a parabolic concentrator solar tracking system integrated with a tubular solar still. Desalination. 2017;411:1–4.