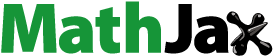
Abstract
This research studies the deposition ability of Cu2ZnSnS4 from a mixed element powder and without sulfurization by a novel flash single thermal evaporator technique. Elemental powders of the source, S, Sn, Zn, and Cu, are held in an evaporation boat to mix their vapour together and to evaporate the mixture at a high temperature. The effect of substrate temperature on the thin layers structure properties, optical properties and surface morphology is explored by X-ray diffraction, Raman spectroscopy, scanning electron microscopy, energy dispersive X-ray, atomic force microscopy, and UV-Vis -NIR spectroscopy. Cu2ZnSnS4 films of kesterite structures are fabricated successfully at various substrate temperatures by this technique in one step without a sulfurization process. The substrate temperatures affect the composition and optical properties of the prepared layers by changing the concentration of elements and crystallinity. The layer prepared at 250°C exhibits an optimal stoichiometry and an optical bandgap value of 1.51 eV.
1. Introduction
Cu2ZnSnS4 (CZTS) layers have been assumedfavourable layers solar cells devices due to many aspects, such as a high absorption coefficient of 104·cm−1, earth-abundant and suitable bandgap(1.0–1.5 eV), [Citation1,Citation2], low-cost and environmentally friendly materials. The maximum efficiency of CZTS solar cells is theoretically expected to be over 30% [Citation3], whereas the highest experimental efficiency is 11.3 ± 0.3% [Citation4]. Therefore, CZTS solar cells will be economically competitive compared to other conventional solar cells devices in the coming years.
CZTS thin layers can be achieved by either chemical or physical techniques. Chemical procedures include sol–gel methods [Citation5,Citation6], electrodeposition [Citation7,Citation8], spray pyrolysis [Citation9,Citation10], dip-coating [Citation11] and spin-coating [Citation12]. Physical vapour deposition techniques include pulse laser deposition [Citation13], sputtering [Citation14,Citation15], electron beam [Citation16], plasma-assisted coevaporation [Citation17] and thermal evaporation [Citation18].
Most chemical methods are cheaper than physical vapour deposition due to the cost of vacuum systems and their accessories. In contrast, the physical vapour deposition techniques provide very good films in terms of impurity and controlling the uniformity of the thickness and reduce toxic gases. Therefore, most industrial production and high-efficiency solar cells use vacuum processes.
The thermal evaporation system is the simplest among the physical vapour deposition techniques because it does not need an expensive high power supply generator or laser source for evaporating the materials. However, the source for a single thermal evaporation should be a compound of the elements [Citation19]. Nevertheless, there is a possibility that the compound breaks into its elements, which may evaporate sequentially according to their evaporation temperature and vapour pressure, which in turn creates multiple layers rather than one layer of the compound. Therefore, coevaporation systems [Citation20] have been used, which consist of more than one thermal evaporation source.
CZTS thin films can be either deposited by coevaporation only, which is a one-step process, or followed by sulfurization at high temperature.
In this study, we investigated the ability to prepare stoichiometric CZTS thin layers by a single thermal evaporator process direct from elemental powder and without sulfurization. A single thermal evaporation boat is designed to prevent the ejection of materials during raising the boat temperatures and to allow the components to mingle with each other before they sublimate simultaneously at high vapour pressure. Then, the prepared layers’ surface morphology and optical and structural properties at different substrate temperatures are inspected.
2. Experimental procedure
Copper, zinc, tin, and sulfur powders (99.99%) were weighed to 0.124, 0.023, 0.013 and 0.207 g, respectively. The powders were put in a directional evaporation boat (Testbourne Ltd, S17B-.010Ta). After that, the element powders (Cu, Sn, Zn and S) were mixed with each other by shaking the boat and then placed in the end of the boat from the inside, and 10 3-mm-diameter balls of high purity aluminium oxide (Al2O3) were inserted between the mixture and the exit hole. Then, the hole of the directional boat was closed by one ball. A weight of 0.725 g of bulk molybdenum metals was placed on the ball, as shown in Figure .
The vacuum system was pumped by a turbomolecular pump (Leybold TURBOVAC 191) to start a pressure of about 4.5 × 10−5 mbar. The temperature of the evaporation boat was increased quickly to around 1600°C. The ball and the weight fell off due to the high pressure of chemicals inside the boat. Then, the elements evaporated at the same time. The layers were prepared onto soda-lime glass at various substrate temperature (S.T.) of 100, 200, 250, and 350°C.
The diffractometer Shimadzu XRD-6000 was used to achieve the X-ray diffraction (XRD) of CZTS layers. The current and the high voltage of X-ray tube were 30 mA and 40 kV, respectively. Scanning electron microscopy (SEM) images were taken by the microscope Shimadzu SUPERSCAN SSX-550. Energy dispersive X-ray analysis (EDX) was achieved by a desktop SEM (JCM-6000). Surface morphology of layers was studied using Atomic force microscope (AFM) Veeco CP-II with Si tips in noncontact mode. The scan rate was 1 Hz.
The Shimadzu 3150 spectrophotometer was used to measure the optical absorption spectra. The spectral range of transmittance, T(λ) and the reflectance R(λ) was between 190 and 1500 nm. Raman spectroscopy was achieved by spectrometer Bruker Senterra. The wavelength of the laser was 532 nm and the power was 5 mW. The range of the spectra, which were collected from the samples, was between 200 and 600 cm−1 with and 3.5 cm−1 resolution.
3. Results and discussion
EDX analyses of the as-deposited layers of CZTS at different S.T. are illustrated in Figure . The variation in the atomic percentages of elements is shown in Figure . The elemental compositions are calculated in Table . It is obvious that increasing the S.T. reduces the atomic percentage of Zn and Sn significantly, which in turn increases the atomic percentage of Cu. The most stoichiometric films are deposited at 200 and 250°C. The optimal elements composition for solar cell device is Zn-rich and Cu-poor [Citation21], which is achieved at a S.T. of 200°C, Copper/(Zinc + Tin) = 0.8 and Zinc/Tin = 1.11. The atomic percentage of sulfur and the atomic ratio of S/metal are very good for all deposited films in terms of stoichiometry. Therefore, the films do not need a sulfurization process.
Figure 3. The variations in element composition of the prepared CZTS samples with substrate temperature.

Table 1. Elemental compositions of the deposited layers at various S. T.
XRD patterns of the as-prepared samples at different S.T are illustrated in Figure . It is clear that the layer prepared at 100°C is amorphous. Crystallinity appears at 200°C, but it is slight since there are no high and sharp peaks. The peaks at 28.4°, 32.7°, 47.3° and 56.1° related to the (112), (200), (220) and (312) planes, respectively. These planes confirm the structure of kesterite CZTS (JCPDS card number 26–0575) [Citation18,Citation22] and they are obvious in the layer deposited at 350°C. The preferred orientation in both layers, which were deposited at 250 and 350°C, is along the (112) crystallographic plane.
In the layer deposited at 350°C, the existence of other planes increases such as (200), (220) and (312). Some small peaks clearly appear due to the creation of secondary phases of either Cu2S or SnS [Citation23].
Lattice constants (a and c) and crystallite size (D) depend on the layer structure. Where the microstrain (ϵ) and dislocation density ρ are indicators of the existence of defects. These parameters are listed in Table . The lattice constants for the tetragonal phase structure are deduced using the following formula [Citation24]:
(1)
(1) d is the interplanar distance between planes and h, k, and l are the Miller indices.
Table 2. Diffraction of X-ray data of layers deposited at 200°C, 250 and 350°C.
Sherrer’s formula is used to calculate the crystallite size (D) [Citation25]:
(2)
(2)
β is the full width at half-maximum (FWHM) in radians, θ is the Bragg angle and λ is the wavelength of the X-ray.
The microstrain (ϵ) [Citation26] and dislocation density (ρ) [Citation27] are deduced by the following equations:
(3)
(3)
(4)
(4)
These values of lattice constants confirm that the structure is tetragonal since the value of c/2a is close to unity. The microstrain and the dislocation density decrease as the substrate temperature increases due to improving the crystallinity. Large grain size of absorber material have important impacts on the solar cell energy conversion solar cells [Citation28].
Raman spectroscopy confirms the existence of the CZTS structure and the effect of substrate temperature on this structure. As shown in Figure , there is no peak for the layer deposited at 100°C, confirming that the layer does not have a CZTS structure, as proven by the XRD results. The peaks of the Cu2ZnSnS4 structure existed at 329 cm−1, 334 cm−1 and 337 cm−1 for films deposited at 200, 250 and 350°C, respectively. The main peak of CZTS is refer to the vibration of A1 mode arising from the sulfur atoms vibrations of in the Cu2ZnSnS4 lattice, whereas the rest of the atoms remain stationary [Citation22]. The intensity of the peak increased from 29.6 to 378 as the S.T. increased from 200 to 250°C and then decreased to 283 at a S.T. of 350°C. Increasing the intensity of the peak indicates that the CZTS structure improved in the layer. Therefore, the film prepared at 250°C has a relatively best CZTS structure. The weakness of the peak at a S.T. of 200°C indicates that CZTS has some structural disorder (e.g. Cu and Zn cation disorder) [Citation29,Citation30]. However, the smallest FWHM value of the film deposited at a S.T. of 350°C suggests a reduction in the structural disorder or anti-site defects and increased crystallinity. The Raman peak shift from 329 cm−1–337 cm−1 can be explained by increasing the bond strength or reducing the mass of the elements according to Keating’s model [Citation31,Citation32].
The SEM images with 2 kx magnification are shown in Figure . All films lack voids and cracks with changes in the shape of the grain size and roughness. Most of the surface of the film prepared at a S.T. of 100°C is smooth with some particles on the surface, confirming that the film is amorphous. The grains became larger and interconnected as the S.T. increases. This change is also observed by AFM images, as shown in Figure . While the crystallite size increased from 37.22–245.46 Å as listed in Table . The value of the root mean square (RMS) roughness increases from 35.4–57.8 nm as the S.T. increases from 100 to 350°C.
The optical absorbance (A) of the films was calculated from T and R as shown in Figure , by the following equation [Citation33]:
(5)
(5) In general, increasing the S.T. increases the absorbance of the layers due to improving the film crystallinity. The absorbance of the film prepared at 200°C is higher than that deposited at 250°C, possibly because of the differences in compositions, as illustrated in Table .
The following equation:[Citation34] was used to deduce the absorption coefficient, α,:
(6)
(6) where d is the layer thickness.
As shown in Figure , the absorption coefficient increases as the S.T. increases, especially in the visible range. The high increase in the film prepared at 350°C is not due to the substrate temperature only but is affected more by the change in the composition of the film, which in turn reduces the value of the band gap Eg.
Eg is deduced from the following equation:
(7)
(7) where hν is the photon energy and A is a constant. Extrapolation of the curves down to a zero (αhν)2 level yields Eg values of 1.76, 1.7, 1.51 and 1 eV for thin films prepared at 100, 200, 250 and 350°C, respectively, as shown in Figure . The variation in the bandgap depends on the S.T. which mainly affects the composition and crystallinity of the layers. The optimal band gap is ∼1.51 eV [Citation35], which for the layer prepared at 250°C had the best composition, as listed in Table . Then, thin films with Cu/(Zn + Sn) stoichiometry of ∼1 has promising potential for thin film solar cell application [Citation36]. The reduction of the Eg to approximately 1 eV could be due to the electronic states created in the band gap and serve as carrier trapping recombination centres. These carrier trapping centres existed as a result of the Cu2-xS secondary phase [Citation37]. This could be the case with the layer prepared at 350°C because the film was copper rich and had a low concentration of zinc and tin.
4. Conclusions
In this study, Cu2ZnSnS4 layers were prepared successfully by a new process of flash single thermal evaporation. The source was a mixture of the elements without ball milling or sintering. An evaporation boat was designed to confine the elements tightly at high temperatures before they evaporated simultaneously. The layers were prepared at S.T. of 100, 200, 250 and 350°C. The film that was deposited at 100°C was nonstoichiometric and amorphous since it had no X-ray or Raman peaks. Increasing the substrate temperature reduced the concentrations of Zn and Sn to obtain the best stoichiometry and kesterite structures at 200 and 250°C. The crystallite size and roughness increased as the substrate temperature increased, whereas the microstrain and dislocation density decreased. The peak of the Raman shift CZTS structure increased from 329 cm−1–337 cm−1 for layers as the S.T. increased from 200 to 350°C, whereas the FWHM value decreased. The absorbance and the absorption coefficient of the layers increased with S.T., but the Eg decreased as a result of modifications changes in the crystallinity and the elements concentration of the layers. The optimal band gap was achieved at approximately 1.51 eV for the film deposited at 250°C.
Disclosure statement
No potential conflict of interest was reported by the author(s).
References
- Yang KJ, Sim JH, Son DW, et al. Effects of the compositional ratio distribution with sulfurization temperatures in the absorber layer on the defect and surface electrical characteristics of Cu2ZnSnS4 solar cells. Prog Photovoltaics. 2015;23:1771–1784.
- Yang KJ, Son DH, Sung SJ, et al. A band-gap-graded CZTSSe solar cell with 12.3% efficiency. J Mater Chem A. 2016;4:10151–10158.
- Shockley W, Queisser HJ. Detailed balance limit of efficiency of p-n junction solar cells. J Appl Phys. 1961;32:510–519.
- Green M, Dunlop E, Levi D, et al. Solar cell efficiency tables (version 54). Prog Photovoltaics Res Appl. 2019;27:565–575.
- Orletskyi IG, Solovan MM, Brus VV, et al. Structural, optical and electrical properties of Cu2ZnSnS4 films prepared from a non-toxic DMSO-based sol–gel and synthesized in low vacuum. J Phys Chem Solids. 2017;100:154–160.
- Kunihiko Tanaka N, Moritake Uchiki H. Preparation of Cu2ZnSnS4 thin films by sulfurizing sol–gel deposited precursors. Sol Energy Mater Sol Cells. 2007;91:1199–1120.
- Ahmed S, Reuter KB, Gunawan O, et al. A high efficiency electrodeposited Cu2ZnSnS4 solar cell. Adv Energy Mater. 2012;2:253–259.
- Jiang F, Ikeda S, Harada T, et al. Pure Sulfide Cu2ZnSnS4 thin film solar cells fabricated by preheating an electrodeposited metallic stack. Adv Energy Mater. 2014;4:Article 1301381.
- Orletskii IG, Mar’yanchuk PD, Solovan MN, et al. Optical properties and mechanisms of current flow in Cu2ZnSnS4 films prepared by spray pyrolysis. Phys Solid State. 2016;58:1058–1064.
- Orletskii IG, Mar’yanchuk PD, Solovan MN, et al. Peculiarities in electrical and optical properties of Cu2Zn1–x Mn x SnS4 films obtained by spray pyrolysis. Tech Phys Lett. 2016;42:291–294.
- Ahmed Z, Bouchaib H, Hicham L, et al. Effect of copper concentration on physical properties of CZTS thin films deposited by dip-coating technique. Appl Phys A. 2019;218.
- Zhang X, Fu E, Zheng M, et al. Fabrication of Cu2ZnSnS4 thin films from ball-milled nanoparticle inks under various annealing temperatures. Nanomaterials. 2019;9:1615.
- Vanalakar SA, Agawane GL, Shin SW, et al. A review on pulsed laser deposited CZTS thin films for solar cell applications. J Alloys Compd. 2015;619:109–121.
- Scragg JJ, Ericson T, Kubart T, et al. Chemical insights into the instability of Cu2ZnSnS4 films during annealing, Chem. Mater. 2011;23:4625–4633.
- Liu F, Li Y, Zhang K, et al. In situ growth of Cu2ZnSnS4 thin films by reactive magnetron co-sputtering. Sol Energy Mater Sol Cells. 2010;94:2431–2434.
- Mkawi EM, Al-Hadeethi Y, Shalaan E, et al. Substrate temperature effect during the deposition of (Cu/Sn/Cu/Zn) stacked precursor CZTS thin film deposited by electron-beam evaporation. J Mater Sci: Mater Electron. 2018;29:20476–20484.
- Ye L, Junfang C, Junhui M. Properties of Cu2ZnSnS4 (CZTS) thin films prepared by plasma assisted co-evaporation. J Mater Sci: Mater Electron. 2015;26:6546–6551.
- Choudhari NJ, George SD, Raviprakash Y. Influence of sulfurization time and Cu-ZnS-Sn stack order on the properties of thermally evaporated CZTS thin films. J Mater Sci: Mater Electron. 2022.
- Shi C, Shi G, Chen Z, et al. Deposition of Cu2ZnSnS4 thin films by vacuum thermal evaporation from single quaternary compound source. Mater Lett. 2012;73:89–91.
- Schubert B-A, Marsen B, Cinque S, et al. Cu2ZnSnS4 thin film solar cells by fast coevaporation. Prog Photovoltaics Res Appl. 2011;19:93–96.
- Byungha S, Oki G, Yu Z, et al. Thin film solar cell with 8.4% power conversion efficiency using an earth-abundant Cu2ZnSnS4 absorber. Prog Photovolt Res Appl. 2013;21:72–76.
- Ghediya PR, Chaudhuri TK. Electrical conduction of CZTS films in dark and under light from molecular solution ink. J Alloys Compd. 2016;685:498–506.
- Kalinauskas P, Norkus E, Mockus Z, et al. The influence of removal of secondary phases and dissolution By-product from the surface of Cu2ZnSnS4film on the photoelectrochemical response of this film. J Electrochem Soc. 2020;167:0026513.
- Cullity BD. Elements of X-Ray diffraction. London: Addison-Wesley Publishing Company, Inc; 1956.
- Scherrer P. Bestimmung der Grösse und der Inneren Struktur von Kolloidteilchen Mittels Röntgenstrahlen, Nachrichten von der Gesellschaft der Wissenschaften, Göttingen. Math Phys. 1918;2:98.
- Bedira M, Öztaş M, Bakkaloğlu ÖF, et al. Investigations on structural, optical and electrical parameters of spray deposited ZnSe thin films with different substrate temperature. Eur Phys J B. 2005;45:465–471.
- Gadallah A-S, El-Nahass MM. Structural Optical constants and photoluminescence of ZnO thin films grown by Sol-Gel spin coating. Adv Condens Matter Phys. 2013;2013.
- Tumbul A, Aslan F, Goktas A, et al. Highly stable ethanol-based Cu2ZnSnS4 (CZTS) low-cost thin film absorber: Effect of solution aging. Mater Chem Phys. 2021;258:Article 123997.
- Valakh MY, Kolomys OF, Ponomaryov SS, et al. Raman scattering and disorder effect in Cu2ZnSnS4. Phys Status Solidi (RRL)—Rapid Res Lett. 2013;7(4):258–261.
- Scragg JJS, Choubrac L, Lafond A, et al. A low-tempera-ture order-disorder transition in Cu2ZnSnS4thin films. Appl Phys Lett. 2014;104(4):Article 0041911.
- Papadimitriou D, Esser N, Xue C. Structural properties of chalcopyrite thin films studied by Raman spectroscopy. Phys Status Solidi (B). 2005;242:2633–2643.
- Lai F-I, Yang J-F, Wei Y-L, et al. High quality sustainable Cu2ZnSnSe4(CZTSe) absorber layers in highly efficient CZTSe solar cells. Green Chem. 2017;19:795–802.
- Demichelis F, Kaniadakis G, Tagliferro A, et al. New approach to optical analysis of absorbing thin solid films. Appl Opt. 1987;26:1737–1740.
- Al-Ani S, Al-Ramadin Y, et al. The optical properties of polymethylmethacrylate polymer dispersed liquid crystals. Polym Test. 1999;18(8):611–619.
- Zhuk S, Kushwaha A, Wong TKS, et al. Critical review on sputter-deposited Cu2ZnSnS4 (CZTS) based thin film photovoltaic technology focusing on device architecture and absorber quality on the solar cells performance. Sol Energy Mater Sol Cells. 2017;171:239–252.
- Tumbul A, Göktaş A, Zarbali MZ, et al. Structural, morphological and optical properties of the vacuum-free processed CZTS thin film absorbers. Mater Res Express. 2018;5:Article 0066408.
- Liu W-S, Chen S-Y, Huang C-S, et al. Investigation of Zn/Sn ratio for improving the material quality of CZTS thin films with the reduction. J Alloys Compd. 2021;853:Article 157237.