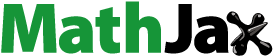
Abstract
The study theoretically investigates the dynamic of an injection-locked semiconductor laser with zero linewidth enhancement factor. The stability map of the injection locking process is drawn, and bifurcation diagrams are generated. Carrier density dynamics are also investigated. The study has reported different periodical and chaotic behaviour and revealed the dynamics of carriers with zero linewidth enhancement factor.
1. Introduction
Semiconductor lasers are considered class B lasers. These lasers have a distinguished feature regarding their linewidth. The dependence of the refractive index of the active materials on the carrier density causes coupling between phase change and intensity, broadening the laser signal’s linewidth. This coupling was first studied by C. Henry in 1982 [Citation1], where he introduced a crucial parameter called LED or Henry factor. This factor plays a significant role in determining the line width of the laser, mode stability, and the chirp under current modulation. Hence, several studies have been devoted to revealing the effect of this factor [Citation2–4].
The linewidth enhancement factor (LEF), also known as the Henry factor, helps to differentiate the behaviour of semiconductor lasers considering various types of lasers [Citation1,Citation5]. Extensive investigations have been conducted for LEF [Citation6–8]. A previous study by Guiliani et al. [Citation9] used the self-mixing method and revealed that the α-factor varies for some lasers with the emitted power. However, this variation is associated with the differences in laser linewidth. The α-factor significantly impacts fibre dispersion used in optical fibre communication systems [Citation10]. This factor can be measured using optical feedback techniques [Citation11] and self-mixing [Citation9,Citation12]. The device characteristics can be enhanced by observing a zero LEF in quantum dot lasers [Citation11]. Moreover, optical injection strongly changes the behaviour of the LEF in quantum dot lasers [Citation13].
This factor has also shown a significant effect in modern applications, including fibre dispersion transmission systems [Citation14], intensity noise in semiconductor laser sensors [Citation15], and mode-locked ring lasers [Citation16]. Generally, this factor takes higher values in bulk active material lasers, and its value becomes smaller as the quantum confinement increases (quantum wells QW to quantum dots QD) [Citation17]. Zero [Citation18,Citation19] and near zero [Citation20,Citation21] LEF have been reported for QD, QW, and Q-dash lasers. LEF also strongly depended on the injection current in tunnel-injection quantum dot lasers [Citation22]. The manipulation of the LEF in injection-locked Quantum-Dash Fabry–Perot Laser was demonstrated by the threshold gain shift resulting from high optical injection [Citation23]. In QD lasers, controllable [Citation24] and temperature-insensitive [Citation25] LEFs have been demonstrated. This factor was also found to increase with increasing carrier density and decreasing temperature and energy level separation [Citation26]. However, the most interesting results are the reporting of negative LEF [Citation24,Citation27]. Increasing the p-doping at a low injected current in a QD laser was found to tune the LEF to a negative value [Citation24]. The negative value of the LEF was theoretically attributed to the nonparabolicity and many-body effects [Citation27].
One of the previous theoretically demonstrated a significant impact of LEF on the stability map [Citation28]. The chaotic behaviour is enhanced when the LEF grows and can be used in cryptographic communications. The LEF also plays an important part in the time-delay signature (TDS) in chaotic semiconductor ring lasers (SRL) to benefit optical chaotic secure communications [Citation29]. Another recent study [Citation30] found that altering the LEF causes discontinuities to form due to disaggregation from so-called shrimp-like structures. The chaotic synchronization has been focused on recently because of its potential applications in broadband data transfer and communication security [Citation31,Citation32]. The dynamical system in semiconductor lasers, or any other system, experiences transitions to chaos from stability via a process known as routes to chaos [Citation33]. A recent study by Locquet [Citation34] provided a thorough assessment of experimental exploration of the paths to chaos in a semiconductor laser subjected to optical feedback from a distant reflector, including period-doubling, quasi-periodic, and subharmonic approaches. These routes, chaos, and other dynamical behaviours are likely to be examined using a bifurcation diagram to show the alteration and reaction of the system [Citation35,Citation36]. This bifurcation is used to analyze the dynamics of semiconductor lasers both empirically and theoretically [Citation37–40].
This study raises a simple question; what is the dynamic of an optically injection-locked semiconductor laser with zero LEF. This question was answered by theoretically investigating the optical injection dynamics of a semiconductor laser in terms of stability map, bifurcations, and carrier density. This could be of important uses in generating chaos in lasers with low LEF, such as quantum dots lasers.
2. Model
Linewidth enhancement factor (LEF) or α-factor can be expressed by the ratio of the changes in the refractive index (Δn) and mode gain (Δgm) caused by changes in carrier density (ΔN) as follows [Citation1]:
To study the dynamics of injected lasers, a simple model was used based on Lang’s approach, as described in [Citation19]. Our model consists of two lasers: Slave laser (SL) or the injected laser and the Master laser (ML) or the injecting laser with an isolator placed between them as shown in .
The rate equations were solved using the Runge–Kutta method to reveal the system’s dynamics. The stability map is drawn in two dimensions as a function of the injection strength (K) and frequency detuning (Δf). At the same time, the bifurcation diagram is recorded by taking the extrema of the electric field of the injected slave laser. The power spectra are taken by applying a fast Fourier transform (FFT) to a certain time window of the slave laser electric field. Finally, the carrier density is normalized to its value at transparency. The parameters used in our simulation are all experimentally characterized as shown in [Citation41].
Table 1. Parameters of the standard model.
3. Results and discussion
The investigation started by drawing the stability map (injection level K vs. frequency detuning Δf) of the slave laser (SL) under a single optical injection, called master laser (ML). shows a map where colours denote the system’s dynamics. The white colour indicates the stable locking region.
Stability in injection locking can be defined as the SL being nicely locked to the ML, with a side peak or relaxation oscillation frequency (ROF) less than – 20 dB relative to the main locked peak [Citation42]. An example of this behaviour is shown in e. The yellow colour represents the period-one (P1) dynamics, where the SL is not locked to the ML with the strong presence of the latter with other signals at the same spacing of the frequency detuning. Such a dynamic is shown in a and a. The orange region indicates the quasi-periodic dynamics (QP), where the system exhibits semi-periodic behaviour, as shown in c. Finally, the dark red colour indicates the chaotic behaviour, which is confined in very small dots as shown in the figure.
The two arrows show the operating points where the bifurcations in Figures and are taken. The first observation is the symmetrical nature of the locking bandwidth (the white region). The asymmetrical locking bandwidth was previously attributed to the non-zero value of the LEF [Citation43]. The fluctuation in carrier density modifies the refractive index, leading to a shift in the resonance frequency. As this effect is eliminated by setting the LEF equal to zero, the locking bandwidth appears symmetrical. This symmetry is not only for the locking bandwidth but also shown for the rest of the map, where the behaviour is exactly mirrored between the negative and positive detuning side, which is not reported before, to the best of our knowledge. The disappearance of chaotic islands reported with non-zero LEF can also be noticed [Citation44,Citation45]. Although these islands are not shown, there is still some evidence of semi-chaotic behaviour in the dark red dots shown in the figure. This behaviour is shown in c, as will be discussed shortly.
Instead of the chaotic dynamics, the unlocking region seems to be covered by the quasi-periodic behaviour (the orange). These spots are centred around the ROF of the free-running laser (around 8 GHz), as the injected signal enhances the ROF and creates such behaviour.
To further reveal the dynamics of the SL, the bifurcation diagram was generated on the positive detuning side (since both sides of the map are identical) at Δf = 7.5 and 12.5 GHz, as indicated by the arrows in . This bifurcation is taken by recording the extrema of the SL electric field at each specific injection level. Such a bifurcation diagram at 7.5 GHz is shown in . It can be seen that the system undergoes P1 dynamics [Citation44], where the ML is strongly evident without locking the SL. This behaviour is shown in a (at K = 0.1 as indicated by the label (a) in ). The SL’s power spectrum shows the SL’s location and ML. In contrast, the electric field time series and the trajectory of the electric field-population inversion indicate P1 behaviour. For a qualitative analysis the largest Lyapunov Exponents (LLE) [Citation46] were calculated as shown in the lower box in . As can be seen in the figure, the LLE takes positive values when the system undergoes chaotic dynamic, and negative values when the system is in a stable state, including periodic behaviours. This is in very good consistency with the bifurcation diagram.
Figure 3. Bifurcation diagram (upper box) of the system at 7.5 GHz, the labels (a-e) indicate the points at which the spectra and trajectories in are taken. The lower box shows LLE of the system.

Figure 4. Power spectra (first column), SL electric field time series (second column), and trajectories of the electric field – population inversion (third column) at the points indicated in for K and Δf = 7.5 GHz.

In , the bifurcation diagram is drawn at 12.5 GHz. The system, in this case, maintains P1 behaviour up to nearly 0.4 injection level, as shown in a and b, with the increase of the electric field amplitude as the injection level is enhanced. At K = 0.4 (c in ), the system exhibits period-doubling dynamics, as shown in c, in a semi-chaotic manner, with damped peaks. Further increase in injection level drives the system back to the P1 dynamic, as shown in d and e. In some cases, this P1 was chaotic (around K = 0.55, not shown in ).
Figure 5. Bifurcation diagram of the system (upper box) at 12.5 GHz, the labels (a-e) indicate the points at which the spectra and trajectories in are taken. The lower box shows LLE of the system.

Figure 6. Power spectra (first column), SL electric field time series (second column), and trajectories of the electric field – population inversion (third column) at the points indicated in for K and Δf = 12.5 GHz.

As the injection power increased (at K = 0.2, point (b) in ), period-doubling behaviour (sometimes referred to as period-two P2) was noted [Citation44]. This dynamic is shown in b. Lower secondary peaks are shown in the middle of P1 spacing as shown in the power spectrum. The time series of the electric field and its trajectory with population inversion show the period-doubling behaviour. At K = 0.35 (point c in ), the system exhibits some chaotic behaviour while maintaining in general P1 dynamic, as shown in c. Further increase in injection level (K = 0.5, point d in ) drives the system back to a nice and smooth P1 dynamics as shown in d. Finally, at K = 0.6 (e in ), the system enters the stable locking region as shown in e. The LLE is again can determine the stability and chaos in the system as shown in the lower box in .
As the relation between the LEF and carrier density is very crucial, the investigation revealed the dynamics of carrier density in optically injected semiconductor lasers with zero-LEF. To do so, the stability map was scanned vertically (at constant K) and horizontally (at constant Δf). shows the normalized carrier density as a function of the frequency detuning at different injection levels. As can be seen from the figure, the dynamics of the carriers seem to be very identical, with the enhancement of carrier variation at zero-detuning (when the ML coincides with SL free-running signal) and at the ROF (≈8 GHz). This symmetrical behaviour is attributed to the zero-LEF as it was found that non-zero LEF gives asymmetrical characteristics for carrier density, as shown in the inset of the figure where carrier dynamics is shown with LEF = 1. These results are under publication at the time of writing this submission and are consistent with previous studies [Citation41,Citation47]. It is also clear that the more power injected, the more carriers vary.
Figure 7. Normalized carrier density as a function of frequency detuning at different injection levels. The inset shows the carrier dynamics at LEF = 1.

The other observation was that the carrier variation maxima shifted towards the resonance frequency with increasing injection levels. The previous study [Citation48] has shown that ROF increases with LEF = 3. This can be understood as a result of the gain shift resulting from the change in carrier density and hence the change in refractive index as previously mentioned. However, this effect is almost eliminated with zero-LEF, and this sort of shift is not reported before to the best of our knowledge.
In , the normalized carrier density was drawn as a function of the injection level for various frequency detuning. As can be seen in the figure, away from the ROF (8 GHz), the variation of carriers is almost negligible (1,5,10 and 12.5 GHz), indicating that carriers show no noticeable dynamics except around the ROF.
Figure 8. Normalized carrier density as a function of injection level at different frequency detunings.

The highest variation is noticed at the ROF (green curve) and then around it (purple and blues), as shown in the figure. That means that carrier density variation in the case of zero-LEF is mostly affected by the frequency detuning rather than the injection level. The same investigation was performed for negative frequency detuning values (−2, −5, −7.5 GHz … etc.) and found the same dependence, which indicates that the behaviour of the carrier density is identical on both sides of the frequency detuning.
It is observed that paths to chaos nearly always retain themselves despite changes in the stability map. This raises further issues concerning the dynamics and carrier density inside the laser cavity. However, these issues need to be researched further in the future, along with an attempt to validate these findings experimentally. The proposed future model should also include the effect of spontaneous emission noise.
4. Conclusion
The study has theoretically investigated the dynamics of an injection-locked semiconductor laser with zero-LEF. A very symmetrical map, in terms of stability, chaos, and other behaviours, is reported. In contrast to non-zero LEF, the disappearance of large chaotic islands was noticed. Instead of the chaotic behaviour, most of the unlocking area seems to be covered by quasi-periodic dynamics. The system exhibits different routes to chaos, including the PD and QP routes.
Regarding carrier density dynamics, carriers show maximum variation around the ROF and seem to be enhanced with increasing injection levels. Finally, a slight shift in the maximum variation of carriers was reported towards the resonance frequency with increasing injection level. These results need experimental validation along with theory development. Future studies need to experimentally verify the relation between LEF and routes to chaos.
Acknowledgment
Author acknowledged Dr. Sami Al-Harthi for his help.
Disclosure statement
No potential conflict of interest was reported by the author(s).
Additional information
Funding
References
- Henry C. Theory of the linewidth of semiconductor lasers. IEEE J Quantum Electron. 1982;8(2):259–264.
- Osinski M, Buus J. Linewidth broadening factor in semiconductor lasers–an overview. IEEE J Quantum Electron. 1987;23(1):9–29.
- Agrawal G, Bowden C. Concept of linewidth enhancement factor in semiconductor lasers: its usefulness and limitations. IEEE Photonics Technol Lett. 1993;5(6):640–642.
- Simpson T, Doft F, Strzelecka E, et al. Gain saturation and the linewidth enhancement factor in semiconductor lasers. IEEE Photonics Technol Lett. 2001;13(8):776–778.
- Al-Hosiny NM. Effect of linewidth enhancement factor (LEF) on routes to chaos in optically injected semiconductor lasers. Opt Appl. 2021;51(4). doi:10.1109/JQE.1982.1071522.
- Harder C, Vahala K, Yariv A. Measurement of the linewidth enhancement factor a of semiconductor lasers. Appl Phys Lett. 1983;42(4):328–330. doi:10.1063/1.93921.
- Henning ID, Collins JV. Measurements of the semiconductor laser linewidth broadening factor. Electron Lett. 1983;19(22):927–929. doi:10.1049/el:19830633.
- Yu Y, Giuliani G, Donati S. Measurement of the linewidth enhancement factor of semiconductor lasers based on the optical feedback self-mixing effect. IEEE Photonics Technol Lett. 2004;16(4):990–992. doi:10.1109/LPT.2004.824631.
- Giuliani G, Donati S, Elsässer W. Measurement of linewidth enhancement factor of different semiconductor lasers in operating conditions. Proceedings of SPIE 6184. 2006. doi:10.1117/12.665178.
- Liu ZY, Zhao TG. Effect of linewidth enhancement factor in semiconductor laser on fiber dispersion transmission system. 2015 fifth international conference on instrumentation and measurement, computer, communication and control (IMCCC); IEEE.
- Jumpertz L, Michel F, Pawlus R, et al. Measurements of the linewidth enhancement factor of mid-infrared quantum cascade lasers by different optical feedback techniques. AIP Adv. 2016;6(1). doi:10.1063/1.4940767.
- Fan Y, Yu Y, Xi J, et al. Simple method for measuring the linewidth enhancement factor of semiconductor lasers. Appl Opt. 2015;54(34):10295–10298. doi:10.1364/AO.54.010295.
- Wang C, Chaibi ME, Huang HM, et al. Frequency-dependent linewidth enhancement factor of optical injection-locked quantum dot/dash lasers. Opt Express. 2015;23(17):21761–21770. doi:10.1364/OE.23.021761.
- Liu Z, Zhao T. Effect of linewidth enhancement factor in semiconductor laser on fiber dispersion transmission system. 2015 fifth international conference on instrumentation and measurement, computer, communication and control (IMCCC); 2015; IEEE, pp. 702-706.
- Shrestha J, Blom S, Witzigmann B, et al. Investigation of relative intensity noise in asymmetric external cavity semiconductor laser sensors: influence of dual-line spectral separation and linewidth enhancement factor. IEEE Sensors J. 2015;15(11):6619–6624.
- Takada A, Takada A, Saika M, et al. Effect of linewidth enhancement factor in actively mode-locked ring laser. In: Fiber lasers XI: technology, systems, and applications. San Francisco, CA: SPIE; Vol.8961; 2014. p. 592–598.
- Ukhanov A, Stintz A, Eliseev P, et al. Comparison of the carrier induced refractive index, gain, and linewidth enhancement factor in quantum dot and quantum well lasers. Appl Phys Lett. 2004;84(7):1058–1060.
- Xu Z, Birkedal D, Juhl M, et al. Submonolayer InGaAs/GaAs quantum-dot lasers with high modal gain and zero-linewidth enhancement factor. Appl Phys Lett. 2004;85(15):3259–3261.
- Zubov F, Maximov M, Moiseev E, et al. Observation of zero linewidth enhancement factor at excited state band in quantum dot laser. Electron Lett. 2015;51(21):1686–1688.
- Kondratko P, Chuang S, Walter G, et al. Observations of near-zero linewidth enhancement factor in a quantum-well coupled quantum-dot laser. Appl Phys Lett. 2003;83(23):4818–4820.
- Naderi N, Grillot F, Kovanis V, et al. Simultaneous low linewidth enhancement factor and high bandwidth quantum-dash injection-locked laser. IEEE photonic society 24th annual meeting; 2011; IEEE, pp. 115-116.
- Mi Z, Bhattacharya P. Analysis of the linewidth-enhancement factor of long-wavelength tunnel-injection quantum-dot lasers. IEEE J Quantum Electron. 2007;43(5):363–369.
- Naderi N, Pichet M, Grillot F, et al. Manipulation of the linewidth enhancement factor in an injection-locked quantum-dash fabry-perot laser at 1550 nm. 23rd annual meeting of the IEEE photonics society; 2010; IEEE.
- Alexander R, Childs D, Agarwal H, et al. Zero and controllable linewidth enhancement factor in p-doped 1.3µm quantum dot lasers. Jpn J Appl Phys. 2007;46(4S):2421.
- Sobhani SA, Childs D, Nishi K, et al. Temperature-insensitive zero linewidth enhancement factor in state-of-the-art In (Ga) As/GaAs quantum-dot lasers (conference presentation). Physics and simulation of optoelectronic devices XXVI; 2018, Vol.10526, p. 1052602.
- Vazquez J, Nilsson H, Zhang J, et al. Linewidth enhancement factor of quantum-dot optical amplifiers. IEEE J Quantum Electron. 2006;42(10):986–993.
- Pereira M. The linewidth enhancement factor of intersubband lasers: from a two-level limit to gain without inversion conditions. Appl Phys Lett. 2016;109(22):222102.
- Al-Hosiny NM. Effect of linewidth enhancement factor on the stability map of optically injected distributed feedback laser. Opt Rev. 2014;21(3):261–264. doi:10.1007/s10043-014-0038-5.
- Li JF, Xiang SY, Wen AJ, et al. Role of linewidth enhancement factor on time-delay signature concealment of chaos in mutually-coupled semiconductor ring lasers. 2016 25th wireless and optical communication conference (WOCC); 2016; Chengdu, China, pp. 21–23.
- Chávez CAT, Curilef S. Discontinuous spirals of stability in an optically injected semiconductor laser. Chaos. 2020;30(5). doi:10.1063/1.5119808.
- Al Bayati BM, Ahmad AK, Al Naimee AM. Effect of control parameters on chaos synchronization by means of optical feedback. Opt Commun. 2020;472; doi:10.1016/j.optcom.2020.126032.
- Senlin Y. Chaotic synchronization of mutually coupled lasers with another laser and its encoding application in secret communication. J Opt Commun. 2020. doi:10.1515/joc-2019-0225.
- Al-Hosiny NM. Sensitivity of routes to chaos in optically injected semiconductor lasers. J Nonlinear Opt Phys Mater. 2014;23(3). doi:10.1142/S0218863514500362.
- Locquet A. Routes to chaos of a semiconductor laser subjected to external optical feedback: a review. Photonics. 2020;7(1). doi:10.3390/photonics7010022.
- Wieczorek S, Krauskopf B, Lenstra D. A unifying view of bifurcations in a semiconductor laser subject to optical injection. Opt Commun. 1999;172(1–6):279–295. doi:10.1016/S0030-4018(99)00603-3.
- Wieczorek S, Chow WW. Bifurcations and chaos in a semiconductor laser with coherent or noisy optical injection. Opt Commun. 2009;282(12):2367–2379. doi:10.1016/j.optcom.2009.02.060.
- Wang H, Jiang W, Ding Y. Bifurcation phenomena and control analysis in class-B laser system with delayed feedback. Nonlinear Dyn. 2015;79:2421–2438. doi:10.1007/s11071-014-1822-2.
- Jahanpanah J, Rezazadeh M, Rahdar AA. Formation mechanism of bifurcation in mode-locked class-B laser. Chin Phys B. 2014;23(12). doi:10.1088/1674-1056/23/12/124205.
- Kim B, Locquet A, Li N, et al. Bifurcation-cascade diagrams of an external-cavity semiconductor laser: experiment and theory. IEEE J Quantum Electron. 2014;50(12):965–972. doi:10.1109/JQE.2014.2363568.
- Mercier E, Wolfersberger D, Sciamanna M. Bifurcation to chaotic low-frequency fluctuations in a laser diode with phase-conjugate feedback. Opt Lett. 2014;39(13):4021–4024. doi:10.1364/OL.39.004021.
- Al-Hosiny N, Henning I, Adams M. Correlation of electron density changes with optical frequency shifts in optically injected semiconductor lasers. IEEE J Quantum Electron. 2006;42(6):570–580.
- Hui R, D’Ottavi A, Mecozzi A, et al. Injection locking in distributed feedback semiconductor lasers. IEEE J Quantum Electron. 1991;27(6):1688–1695.
- Henry C, Olsson N, Dutta N. Locking range and stability of injection-locked 1.54µm InGaAsP semiconductor lasers. IEEE J Quantum Electron. 1985;21(8):1152–1156.
- Kovanis V, Gavrielides A. Instabilities and chaos in optically injected semiconductor lasers. Appl Phys Lett. 1995;67(19):2780–2782.
- Al-Hosiny N, Henning I, Adams M. Tailoring enhanced chaos in optically injected semiconductor lasers. Opt Commun. 2007;269(1):166–173.
- Wolf A, Swift JB, Swinney HL, et al. Determining lyapunov exponents from a time series. Phys D, Nonlinear Phenom. 1985;16:285–317. doi:10.1016/0167-2789(85)90011-9.
- Van Exter M, Biever C, Woerdman J. Effect of optical injection on bias voltage and spectrum of a semiconductor laser. IEEE J Quantum Electron. 1993;29(11):2771–2779.
- Jones R, Spencer P, Shore K. Influence of detuned injection locking on the relaxation oscillation frequency of a multimode semiconductor laser. J Mod Opt. 2000;47(11):1977–1986.