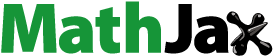
Abstract
Green synthesis of nanoparticles (NPs) has attracted the researcher's attention due to its rapid, cost-effective, sustainable and eco-friendly nature. Current studies were carried out to perform and investigate the green synthesis of NiO NPs with aqueous (NiO(aqueous)) and ethanolic extracts (NiO(ethanolic)) of Acacia nilotica leaves, respectively. The NPs were characterized by spectroscopic, microscopic and thermometric techniques including FTIR, Raman, XRD, SEM, TGA and DSC. The electrochemical properties, antibacterial potential and haemolytic activities of the synthesized NPs were also examined. XRD patterns revealed the face-centred cubic structures of NiO NPs and a high degree of crystallinity. The average crystallite size of NiO(aqueous) NPs was significantly smaller (16 nm) than that of NiO(ethanolic) NPs (28 nm). SEM images show that NiO NPs are spherical. The synthesized NiO NPs demonstrate good electrochemical stability at an operating potential of −0.5-0.5 V. The inhibition effect of NiO NPs(ethanol) against B. subtilis, was comparable to the ciprofloxacin.
1. Introduction
Nanoparticles find extensive biological and non-biological [Citation1,Citation2] applications due to their unique physical and chemical properties, distinct morphology, size, shape, crystalline nature, surface area-to-volume ratio, electro-optical, magneto-optical, chemical and mechanical properties [Citation3]. They can be synthesized by hydrothermal, microemulsion, electrospray, co-precipitation, laser ablation and sol–gel processes [Citation4,Citation5]. However, these approaches are quite expensive, low yielding and involve toxic chemicals which pose a threat to the environment [Citation6]. Now, the trend is shifting towards the green synthesis of NPs in the presence of plant extracts, microorganisms and enzymes [Citation7]. Green synthesis of NPs is attracting the attention of scientists and researchers due to its rapid, cost-effective, eco-friendly and sustainable approach [Citation8]. The plant extract acts as a capping agent through a coordination action by capturing the metal ions in the amylose helix on well-defined sites [Citation9]. The texture of plant leaves and petals acts as a biotemplate and thereby controls the size of the nanoparticles and hinders the agglomeration of particles [Citation10].
Ni(0) nanoparticles find applications as catalysts in hydrogen auto-transfer and transfer hydrogenation reactions, in low-temperature oxidation of carbon monoxide and propane, in diffusion bonding of stainless steel surfaces, for hydrogenation of nitrobenzene, and in supercapacitor electrode materials [Citation11,Citation12]. The surface characteristics of these materials prevent de-adsorption or detachment of the active materials to enhance the contact surface area of the conducting carbon materials which helps to increase the conductivity of the system. Therefore, the replacement of other carbonous materials from plants with these materials is preferable for supporting metal oxide as an electron conductor but also decreases the agglomerations of the nanomaterials during cycles [Citation13–15].
They can also be applied as eco-friendly adsorbents for the removal of dyes and pollutants from waste effluents of tanneries and textile industries and the determination of the chemical oxygen demand (COD) of water [Citation16]. They exhibit antifungal effects against causal agents of wilt in lettuce and tomato under in vitro and greenhouse conditions [Citation17]. More recently, nickel oxide nanoparticles (NiO NPs) have attracted even more attention due to their exceptional properties such as chemical stability, high coercive forces, magneto-crystalline anisotropy, large specific capacitance, excellent durability, electrochemical stability and antiferromagnetic properties. They find applications in catalysis biomedical, supercapacitors and dye-sensitized solar cells [Citation18]. They also demonstrate antioxidant, anticancer, cytotoxic, antifungal, antibacterial, enzyme inhibition and remediation properties and find a lot of catalytic and clinical applications [Citation8,Citation19]. They also find therapeutic applications as biomedicines due to their unique surface area, metal ion releasing/adsorbing ability and cytotoxic effects [Citation20]. Numerous chemical and physical methodologies have successfully been employed to synthesize nanoparticles. The synthesis of high surface area NiO NPs films by sol–gel, spray pyrolysis, electrodeposition, chemical bath deposition and spin coating stand out among the others. However, most pure NiO NP-based films have low adhesion and are challenging to synthesize as ultra-porous NiO NPs with diameters smaller than 20 nm. This results in their limited cycling stability [Citation21]. Green synthesis approaches are investigated as a potential approach to achieving such a goal. In comparison to the aforementioned conventional physical and chemical processes, green synthesis methodologies are energy-efficient, waste-free and ecologically benign.
In this context, to the best of our knowledge, the green synthesis of NiO NPs with Acacia Nilotica (A. nilotica) leaves is still not reported. Current studies were performed to produce NiO NPs in aqueous and ethanolic extracts of A. nilotica leaves. Some of the main advantages of green synthesis of NiO NPs are excellent yield, short duration, simplicity, environmental sustainability, green chemical reaction, and cost-effectiveness. Their structural features were compared by spectroscopic, microscopic and thermometric techniques including FTIR, Raman, XRD, SEM, TGA and DSC. The synthesized NiO NPs were subjected to electrochemical investigations by cyclic voltammetry and their charging/discharging potentials were determined. Finally, the synthesized NPs were tested for their antimicrobial potential and haemolytic activities.
2. Experimental
2.1. Chemicals and instrumentations
The analytical grade chemicals (nickel (II) nitrate hexahydrate, sodium hydroxide, dimethyl sulphoxide (DMSO) and ethanol) originating in Merck, Germany were used to preparing the NiO NPs. The structural analyses of NiO NPs were performed by a Bruker AXS, D8 Advance X-ray diffractometer (XRD). For morphological investigations and to calculate the size of NiO NPs, scanning electron microscopy (SEM) was performed using Hitachi S4800 (Japan). FTIR (Perkin Elmer, USA) spectra were recorded in the range of 4000–400cm−1. Raman spectra were recorded by Reinshaw in Vis Reflex. The compounds were subjected to TGA and DSC by SDT (Q600) thermal analyzer (TA Instruments, USA) under a nitrogen atmosphere at the heating rate of 20°C/min. The electrochemical properties and charging/discharging potential of the NiO NPs were evaluated by Galvanostat/Potentiostat (CS300, China).
2.2. Preparation of plant extracts
The sample plant (A. nilotica) was identified and authenticated by the Department of Biology, Lahore Garrison University, Lahore-Pakistan. Its leaves were collected from a peripheral area of the capital of Lahore (Punjab), Pakistan. The collected leaves of the sample plant were washed with distilled water and were then dried under shade. They were ground and homogenized into a fine powder by using a commercial grinder (TSK-049, West point France). The fine powder of A. nilotica was sieved with 80 mesh size and stored at 4°C for further use. The leave powder (20 g) of A. nilotica was added to 100 mL of distilled water and stirred in an orbital shaker (Gallenkamp, UK) for 24 h. The mixture was filtered through a Whatman filter paper No. 1 to obtain the aqueous extract (as the filtrate), which was stored at 4°C for subsequent use [Citation22]. The same procedure was applied for the preparation of ethanolic extract of leaves by using 20 g of leave powder and 100 mL of ethanol.
2.3. Green synthesis of NiO NPs
50 mL of Ni(NO3)2.6H2O, (5% w/v) was added to 100 mL aqueous extract of plant leaves in a 500 mL round bottom flask, and the contents were heated at 80°C with continuous stirring for 4 h to ensure a complete oxidation and reduction process. The formation of NiO pellets was confirmed by observing a change in colour. The mixture was left in the dark after a thorough stirring to observe a colour change and the formation of precipitates at the bottom of the flask. Then the centrifugation was performed at the rate of 1000 rpm for 30 min and the precipitates were collected from the bottom. After centrifugation, the clear supernatant was discarded while the pellets were collected. The obtained pellets were suspended in deionized H2O and were thoroughly washed 2–3 times to remove impurities. Next, the pellets were dried and calcined at 300°C for 3 h for effective crystallization. The calcined product was then crushed into a fine powder by using a mortar and pestle. The obtained product was of greyish-black colour and was stored in an air-tight container until further use or analysis [Citation23,Citation24]. The same procedure (as given above) was followed for the synthesis of nanoparticles from the ethanolic extract.
2.4. Antibacterial activities
2.4.1. Bacterial strains and antibacterial assay
To test the antibacterial potential of NiO NPs against four bacterial strains (two gram-positive, namely Staphylococcus aureus ATCC 25923, Bacillus subtilis ATCC 6051, and two gram-negative, namely Escherichia coli ATCC 25922, Pseudomonas aeruginosa ATCC 27853) a previously described disc diffusion method [Citation25–27] was employed with minor modifications. The above bacterial strains were purchased from Musaji Adam & Sons, Laboratory Suppliers, Karachi, Pakistan. The surface of nutrient agar media plates was swabbed slowly in three separate directions with an aliquot of 100 µL of inoculum that had been pre-adjusted (10 8 cells/mL) for seeding density. The plates’ surface was covered with sterile filter paper discs containing 5 µL (20 mg/mL DMSO) of NiO NPs solution. The use of DMSO-infused discs as a negative control was contrasted with the use of ciprofloxacin (5 µg) and streptomycin (10 µg) loaded discs as a positive control. The zone of inhibition (ZOI) was measured using a Vernier calliper (Starrett 799A-6/150, USA) following an incubation period of 24 h at 37°C.
2.5. Haemolytic activities
In the haemolysis assay, PBS (phosphate-buffered saline) was employed as a negative control and Triton X-100 (0.1%) was used as a positive control. Out of 10 mL of the taken blood sample, 4 mL was added to 8 mL of 0.2 M PBS at pH 7 and the mixture was then centrifuged for 10 min at the rate of 10,000 rpm. A further washing of the red blood cells (RBCs) containing the pellet was done with PBS at 10,000 rpm. The dilution of the obtained RBCs was done with 40 mL D-PBS. Both the negative and positive controls were made from this test sample. For incubation, the samples were kept for 4 h at room temperature and the samples were vortexed and centrifuged for 3 min at 10,000 rpm. After collecting the supernatant, a plate reader (Bio-Rad, USA) was used to measure the optical density (OD) value at 576 nm. The following equation was then applied to measure the percentage of haemolysis.
3. Results and discussions
3.1. Green synthesis approach
Plants offer a wide range of biochemical properties because of their biodiversity, and hence provide helpful sources for the synthesis of nanoparticles. A large number of phytochemicals, in the extracts of plant leaves, can easily be extracted which makes plant leaves extract an amazing source for the synthesis of metal oxide nanoparticles (MO NPs). The synthesis of NPs is facilitated because of the interesting feature of the plant leaf extract to act as a stabilizing and a reducing agent. The concentrations of biomedical reducing agents vary in the extracts of leaves of different types. Therefore, the compositions of leaf extracts give rise to a huge effect on the synthesis of nanoparticles. In the synthesis of nanoparticles, the involved essential phytochemicals are terpenoids, flavones, ketones, amides, aldehydes and carboxylic acids.
3.2. Mechanism of green synthesis of NiO NPs
Different plant metabolites such as sugars, polyphenols, terpenoids, phenolic acids, proteins and alkaloids are the prime role players in the bioreduction of metal ions to yield nanoparticles. Flavonoids are a large group of polyphenolic compounds that consist of different classes (flavonols, isoflavonoids, anthocyanins, chalcones, flavones and flavanones). These compounds can chelate and actively reduce metal ions into nanoparticles. The flavonoids contain different functional groups which are capable of forming nanoparticles. It has been postulated that the transformation of flavonoids from one tautomeric form (the enol-form) to the other tautomeric form (the keto-form) may discharge a reactive H-atom that can form nanoparticles after reducing the metal ions [Citation28,Citation29]. The O–H and N–H functional groups are responsible for the strong affinities towards metal ions. As a result, a large number of electron donor amine and hydroxyl functional groups act as potential reducing and capping agents to donate electrons to the nickel ions. Besides, several biomacromolecules can also act as surface protectors of NiO NPs through electrostatic and/or steric repulsions, which prevent the formation of aggregations [Citation30,Citation31]. In a nutshell, a combination strategy of leaf extract of A. nilotica is a simple and facile approach to preparing durable and efficient NiO NPs. This is clearly evident from the FTIR, Raman spectroscopies, XRD, SEM, TGA and DSC studies.
3.3. Structural properties
XRD analysis is used to provide information about the crystalline structure and size of particles. The XRD patterns of the synthesized NiO(aqueous) and NiO(ethanolic) NPs are displayed in . It was found that all the diffraction peaks are exactly matched with the standard JCPDS card number 47–1049 corresponding to NiO. The major peaks of synthesized NiO(aqueous) and NiO(ethanolic) NPs were observed at 2θ = 37.2°, 43.2°, 62.7° and 75.3° which correspond to the planes (111), (200), (220) and (311), respectively. Two additional peaks (represented by * in ) were also observed at 2θ = 45.1°, 51.6° in the XRD graph of NiO(ethanolic) NPs, which is likely due to the presence of Ni and Ni2O3 nanoparticles in the sample as is reflected from JCPDD card numbers 45-1027 and 14-0481, respectively. The Scherrer equation, which is given below, was used to calculate the average particle size of the NPs.
where D represents the crystalline size of the particles,
is the Scherrer constant (
= 0.9),
represents the wavelength of light employed for diffraction (
= 1.54 Å),
is the full-width at half-maximum (FWHM) of the diffraction peak and
is the angle of deflection.
The average crystallite sizes of the NiO(aqueous) and NiO(ethanolic) were 16 nm and 28 nm, respectively. So aqueous extract of A. nilotica leaves is more useful than its ethanolic extract for the production of small-sized NiO NPs and these findings are similar to those obtained from SEM analysis (section 3.3).
3.4. FTIR and Raman spectroscopy
FTIR spectra of green synthesized NiO NPs were measured in the range of 4000–500 cm−1 and the obtained data are displayed in . The spectra displayed the peaks for –OH, –CN, –C=O and Ni–O vibrations. However, the peaks of special importance are Ni–O vibrations which were displayed at 635 and 530 cm−1 for NiO NPs synthesized in aqueous and ethanolic extracts respectively; these values are in agreement with the reported studies [Citation32,Citation33].
Table 1. FTIR data of NiO NPs.
Raman spectra of green synthesized NiO NPs were recorded in the range of 0-2000cm−1. The Raman spectrum of NiO NPs(ethanolic) () displayed a strong and broad peak at 449.12 cm−1 due to the Ni–O stretching mode of vibration (1LO mode) and another peak at 1312 cm−1 due to two phonons (2LO mode). The Raman spectrum of NiO NPs(aqueous) () contained a strong broad peak at 460 cm−1 due to the Ni–O stretching mode of vibration (1LO mode). The absence of a magnon peak in the Raman spectra at 1500 cm−1 reveals the antiferromagnetic to the super magnetic transition of NiO NPs [Citation34]. However, the strong intensity in the Raman spectrum of NiO NPs(ethanolic) was observed, since the Raman scattering is an inelastic-scattering process and in ethanolic extraction, less water content was present which was explained by TGA analysis, so the probability of an occurrence of elastic scattering is higher compared with NiO NPs(aqueous).
3.5. Scanning electron microscopy
The surface morphological characteristics of the fabricated nanoparticles were examined by SEM (scanning electron microscopy). The SEM images (with a magnification of 5000) for NiO(ethanolic) and NiO(aqueous) are shown in (a,b), respectively. The images show that mono-dispersive and highly crystalline NiO nanoparticles are synthesized; some particles have a tetragonal appearance. (b) depicts that the particles are highly agglomerated and essentially are clusters of nanoparticles. The SEM image shows the nano-ranged size of polycrystalline particles. The presence of some larger nanoparticles is likely due to the tendency of NiO NPs to agglomerate due to their high surface energy and the high surface tension of the ultrafine nanoparticles. The fine particle size results in a large surface area that, in turn, enhances the nanoparticles’ catalytic activity. The average diameter of the cluster in the case of NiO NPs synthesized in aqueous solution observed from SEM analysis is 16 nm but the NPs synthesized in ethanol show good crystallinity, which can also be confirmed from the XRD graph. The average grain size observed in the SEM image of synthesized NiO NPs in ethanoic extract was 28 nm. Thus, it is confirmed from the SEM image that NiO NPs synthesized in ethanol extracts of A. nilotica leaves show a tetragonal crystal structure.
3.6. Thermogravimetric analysis
Thermogravimetric analysis of both the aqueous and ethanolic extracts was performed to know about the thermal stability and degradation pattern of synthesized nanoparticles. First, in the temperature range of 95–100°C, there is an initial weight loss rate of 8–10%, which is probably caused by the evaporation of the absorbed water (). The moisture content was lower in NiO(ethanolic) than NiO(aqueous). By increasing the temperature from 95°C to 600°C, no further loss in mass was observed; the results thus verify the stability of NPs. So it can easily be demonstrated from the thermogram that both types of NPs are thermally stable up to 600°C. As a result, TGA analysis demonstrates that a large number of bioactive phytochemicals, including phenolic and polysaccharides, are present in the marine cucumber extract and may coat the surface of the nanoparticles to serve as steric and/or electrostatic stabilizing agents [Citation35].
3.7. Differential scanning calorimetry
DSC is always taken between heat flow (w/g) and temperature. Both types of nanoparticles (NiOethanolic and NiOaqueous) were subjected to analysis by differential scanning calorimetry (DSC) and the obtained results are shown in (a,b), respectively. DSC measurements exhibit three distinct regions in this case. The initial region of weight loss is between 25°C and 200°C. As seen by the DSC graph, this region corresponds to the loss of physisorbed water, residual solvent and loosely attached hydroxyl groups in agreement with the prior explanations. Between 200°C and 270°C, the second weight loss area takes place, and a sizable endothermic heat flow is present. This is consistent with Ni(OH)2 breaking down to generate NiO. Therefore, within this range of the calcination temperature, substantial structural changes take place. A further loss of water through the conversion of Ni(OH)2 to NiO is thought to be the cause of the third region’s minimal weight loss, which occurs between 270°C and 600°C. However, a significant exothermic heat flow also occurs along with weight loss. This heat flow is thought to be caused by a structural relaxation in which pores are removed without significantly reducing weight.
3.8. Electrochemical investigations
Cyclic voltammetry (CV) and galvanostatic charging discharging (GCD) were performed to investigate the electrochemical behaviour of the synthesized NiO NPs (Figures and ). A three-electrode system was employed for the electrochemical studies. NiO deposited on nickel foam was used as a working electrode, Ag/AgCl as a reference electrode and a Pt wire as a counter electrode. The 6.0 M concentrated solution of KOH was used as an electrolyte. The CV curves ((a,b)) of NiO nanoparticles show good oxidation and reduction peaks at scan rates of 50, 60, 70, 80, 200, 200 mV/s in the potential range of −0.5 to +0.5 V. The reduction peaks during the cathodic scan and oxidation peaks during the anodic scan are asymmetrical because of the kinetic irreversibility of the oxidation and reduction reactions. These redox reactions are kinetically reversible due to the transfer of electrons and ions. The insertion of electrons or ions during the cathodic scan from +0.5 to −0.5 V reduces Ni3+ to Ni2+ in the presence of a KOH electrolyte. Under an applied electric potential, the ions and electrons migrate towards the NiO electrode through KOH. As the electrolyte does not conduct electrons and the charge-balancing electrons do not flow through the electrolyte so the electrons migrate through an external circuit and recombine with the ions present at the electrode surface. Thus, the electrochemical reactions take place on the NiO electrode. Likewise, the extraction of ions and electrons gives the oxidation of Ni2+ to Ni3+ in the electrolyte solution. This indicates that there is a quantifiable change in the redox reactions due to the addition/removal of hydroxide ions in the synthesized NiO film.
Figure 7. Galvanostatic charge–discharge graphs of NiO NPs (a) NiO NPs(ethanolic) and (b) NiO NPs(aqueous).

The cathodic and anodic currents in the CV curves increased with an increase in the scan rates. Such an increase in the cathodic and anodic currents confirms the reversible redox reaction of the NiO electrode. The value of a given potential is used to decide the completion of the electrolytic process of electrode materials. The Faradaic oxidation and reduction peaks slightly shifted with an increase in the scan rate from 50 to 200 mV/s. Such a slight shift in the peaks indicates a negligible polarization resistance of the electrode material. The CV curves obtained at even higher scan rates (200 mV/s) demonstrate excellent maintenance of their shapes and this confirms the ultrafast kinetics of the rate of the Faradaic process of the indicator electrode.
Next, we performed galvanostatic charge–discharge studies of synthesized NiO NPs at different current densities. The material shows good charge–discharge characteristics even at higher current densities. The galvanostatic charge–discharge was performed in the potential range from 0 to 0.35 V. (a,b) shows the obtained charge–discharge profiles. A good symmetry indicates good reversibility of the Faradaic redox process. The charging process in the potential range of 0–0.35 V shows a nonlinear curve, which indicates the occurrence of an electrochemical reaction. The nonlinear charge–discharge profile demonstrates the pseudo-capacitive character of the NiO material. The following equation was used to calculate the specific capacitance of synthesized NiO NPs.
where
is the constant current (A),
is the discharging time (s),
is the mass of the electrode (g) and
is the potential difference.
For the NiO electrode, the specific capacitance at low current density is much greater due to the fast diffusion of electrolyte ions into the working electrode. At higher current density, the diffusion of ions occurs just on the surface of the working electrode.
We measured the cyclic stability of the NiO electrode by repeating galvanostatic charge–discharge measurements up to 10,000 cycles at the current density of about 1 mA under the applied potential in an electrolyte solution. It was found that the retention capacity depends on the number of cycles. The specific capacitance calculated at 1 mA current density gives the maximum value of 983.3 mAh/g and 1405 mAh/g for NiO NPs(aqueous) and NiO NPs(ethanol), respectively. We observed that the synthesized NiO NPs in ethanol show a higher value (better results) of specific capacity, which is consistent with the previous results (Sections 3.1 and 3.3). The better results of NiO NPs(ethanol) as compared to NiO NPs(aqueous) are likely because of the smaller size and larger surface area of the former NPs. From the above discussion, it can be concluded that the synthesized electrode materials possess promising electrochemical stability with remarkable reversibility because of the redox reaction.
A better result of specific capacitance can be calculated by using GCD curves. Thus, calculations were done for both synthesized NiO NPs(ethanolic) and NiO NPs(aqueous) by using GCD graphs provided in . The specific capacitance of NiO NPs(ethanolic) calculated at current densities 1, 2, 3, 4 and 5 mA is 770, 122, 98, 95 and 94.3 mAh/g, respectively. The specific capacitance of synthesized NiO NPs(aqueous) at current densities 1, 2, 3, 4 and 5 mA was also calculated as 914, 685, 580, 528 and 515 fAh/g, respectively. These results indicate that the specific capacitance of NPs synthesized in ethanol demonstrates better results.
3.9. Antibacterial activity by biofilm inhibition assay
NiO NPs(ethanol) showed better results than NiO NPs(aqueous) in their antibacterial efficacies. The inhibition effect (the zone of inhibition = 18.30 ± 0.58 mm) of NiO NPs(ethanol) against B. subtilis ATCC 6051 was considerable and comparable to that of standard drug ciprofloxacin. The NiO NPs(ethanol) showed a greater inhibition effect than that of streptomycin against B. subtilis ATCC 6051. Streptomycin was used as a positive control and yielded an inhibition zone of 11.1 ± 0.21 mm. NiO NPs(aqueous) also produced a better inhibition effect than that of streptomycin () against the B. subtilis ATCC 6051 and P. aeruginosa ATCC 27853 with zones of inhibition equal to 13.7 ± 0.58 mm and 10.5 ± 0.50 mm, respectively.
Table 2. The zone of inhibition (mm, Mean ± SD) of fruit peel extract and ciprofloxacin and streptomycin.
Antibacterial results may differ due to the use of different solvents for herbal extraction, plant parts, method, microorganisms in the environment, the region where the plants grow up and the timer of harvesting of the plants. The ratios of phytochemical components, such as polyphenols and flavonoids in the plant extract, directly influence the antimicrobial properties of the plant.
An electrostatic interaction between nano-scaled materials and bacterial strains gives rise to the generation of reactive oxygen species. Reportedly, these reactive oxygen species are responsible for the death of bacterial cells (). There are two possibilities for the reactions of nanomaterials with the bacterial strains. In the first possible reaction, a strong interaction between Ni2+ ions and the negatively charged parts of the bacterial cells results in the collapse of the bacterial cells. In the second possible reaction, an electronic excitation from the valence to the conduction band occurs upon irradiation of the NiO surface with light. Further electronic reaction with O2 produces O−2 radicals which result in the production of H2O2. A reaction of h + with water produces ·OH. These produced species (O−2· and ·OH) play a prime role in breaking down the lipid or protein molecules present on the outer surface of the bacterial cells [Citation36,Citation37]. So, the antibacterial potential of nanoparticles can be attributed to Ni2+ ions that come from the NiO nanoparticles. The membrane’s permeability increases due to the release of ions to support oxidative stress. This oxidative stress causes the death of the cells. Similar arguments have been made in some previously reported studies, where the antibacterial properties of some other nanoparticles have been studied in detail [Citation38,Citation39].
The increasing resistance of microbes to drugs has made the search for new antimicrobial drugs an extremely important area of research. A current approach is to screen medicinal plants for novel antimicrobial principles.
3.10. Haemolytic activity
Synthesized NiO NPs were subjected to haemolytic activity evaluations to analyze their toxic haemolytic effects on human beings. Such kinds of activities are used to ensure that the tested products are safe and can be used as medicinal drugs in future [Citation40]. Triton-X100 and phosphate buffer were used as positive and negative controls, respectively. The obtained data are shown in . The synthesized NPs have shown negligible activities against red blood cell lysis than Triton-X100 and have shown almost no toxic haemolytic effects.
Table 3. Haemolytic activities of NiO NPs.
3.11. Comparison of results with previous studies
The synthetic raw materials and important properties of the investigated NiO NPs were also compared with already reported similar studies and the comparison has been displayed in . The literature reports that the size of Ni and NiO nanoparticles lies at 3.4–60 nm and 1.42–55 nm, respectively when the NPs are synthesized with different plant extracts (). These studies suggest face-centred cubic or spherical structures in most cases and polyhedral, irregular or agglomerated forms in a few cases. In the current studies, the sizes of NiO NPs synthesized with aqueous and ethanolic extracts of A. nilotica leaves were 16 and 28 nm, respectively.Green chemistry technology is in an early phase of development and expansion. It is foreseeable that this technology is going to be used widely throughout the world in future. From the industrial and commercialization point of view, the implementation of the basics of green chemistry in the expansion of new materials is seriously under consideration. There exists a strong connection between the chemical structure and the functioning of these materials. A better understanding of the key information of preparation methods of the nanomaterials can lead to design principles for efficient synthesis of these materials. The bioengineering of molecules, cells and organs of the above-mentioned plants is carried out to meet the demanding needs of novel nanomaterials. Green nanotechnology can greatly reduce the negative impact on the environment by lowering pollution and producing an enterprising effect on the design of nanomaterials or products. As indicated in , nanotechnology can remediate existing environment-related problems.
Table 4. The reported green synthesis of NiO NPs.
Green nanotechnology is an ideal solution to lower the riskiness of nanotechnology by removing the negative impacts of the synthesis and applications of nanomaterials. The real applications of these materials require their production on an industrial scale. A large number of these materials are available in the market and are employed to prepare personal care products, clothing and cosmetics. The industrial and production sectors, including medicine and drug delivery, will experience a boost in the development of modern products. On the basis of several implementations to human beings and global development, these disruptive technologies can be commercialized. However, a critical interest is needed for health evaluations and the environmental impacts of these materials. It is a well-known fact that the health hazards of nanoparticles are comprehended slowly but they need to be addressed on an urgent basis because of their practically uncontrollable synthesis and utilization. It is quite alarming that nano-based entities are being produced and included in consumer products at a rapid rate and, therefore, the development of overnight mechanisms is an urgent need of the hour. Safety and health regulations should negotiate regulatory testing cost load very carefully, which could ultimately play an important role in giving preferences to the detrimental effects linked to nanomaterials.
4. Conclusions
Nickel oxide nanoparticles (NiO NPs) were synthesized by a green method using nickel nitrate (Ni(NO3)2.6H2O) and sodium hydroxide (NaOH) in the presence of Acacia nilotica leaf extracts (aqueous and ethanolic). The nanostructures were characterized by X-ray diffraction (XRD) studies, Fourier-transformed infrared (FTIR) and Raman spectroscopies, scanning electron microscopy (SEM), thermogravimetric analysis (TGA) and differential scanning calorimetry (DSC). The NPs were evaluated for their antibacterial potential and haemolytic activities and their electrochemical properties were also investigated. The FTIR analysis verified the existence of Ni–O vibrations at 635 and 530 cm−1 for NPs synthesized in aqueous and ethanolic extracts, respectively. Raman peaks at 449.12 and 460 cm−1 for NiO(aqueous) and NiO(ethanolic), respectively show the Ni–O stretching modes of vibration. The X-ray diffraction patterns revealed that the NiO nanoparticles have a face-centred cubic (FCC) structure which confirmed the presence of a high degree of crystalline nature. The average crystalline size of NiO(ethanolic) was significantly smaller (45 nm) than that (601 nm) of NiO(aqueous). The SEM images verified the spherical shapes of NiO NPs. Thermograms of the NPs show their good stability up to 600°C and loss of 7% moisture up to 95°C. Cyclic voltammetry (CV) measurements were carried at different scan rates in a potential window from −0.5 to 0.5 V. CV measurements have shown prominent oxidation and reduction peaks for NiO(ethanolic) at 0.19 and 0.31 V and NiO(aqueous) at 0.21 and 0.36 V, respectively. It was concluded that the operating potential for the device consisting of nickel oxide electrodes was −0.5–0.5 V. The synthesized material may find future applications as electrode material in supercapacitors. Antibacterial activity evaluations against Staphylococcus aureus and Proteus mirabilis demonstrated that NiO(ethanolic) shows higher potential against the activity of S. aureus than all the other cases. No toxic haemolytic effects were displayed by the synthesized NiO NPs.
Informed consent statement
This study was not performed on humans.
Acknowledgements
Shabbir Hussain: Supervision, Methodology, Formal analysis, Muazzam Ali Muazzam: Review, Analysis, Mahmood Ahmed: Review & editing, Writing – original draft, Muhammad Ahmad: Review, Validation, Zeeshan Mustafa: Review, Shahzad Murtaza: Investigation, Formal analysis, Jigar Ali: Validation, Muhammad Ibrar: Formal analysis, Review, Muhammad Shahid: Review, Muhammad Imran: Formal analysis, Review.
Disclosure statement
No potential conflict of interest was reported by the author(s).
Data availability statement
This study did not report any data.
References
- Nosheen S, Irfan M, Abidi SH, et al. A review: development of magnetic nano vectors for biomedical applications. GSC Adv Res Rev. 2021;8:85–110.
- Mueez A, Hussain S, Ahmad M, et al. Green synthesis of nanosilver particles from plants extract. Int J Agric Env Biores. 2022;7:96–122.
- Hussain S, Amjad M. A review on gold nanoparticles (GNPs) and their advancement in cancer therapy. Int J Nanomater Nanotechnol Nanomed. 2021;7:19–25.
- Rahman MA, Radhakrishnan R, Gopalakrishnan R. Structural, optical, magnetic and antibacterial properties of Nd doped NiO nanoparticles prepared by co-precipitation method. J Alloys Compd. 2018;742:421–429.
- Jia F, Zhang L, Shang X, et al. Non-aqueous Sol–Gel approach towards the controllable synthesis of nickel nanospheres, nanowires, and nanoflowers. Adv Mater. 2008;20:1050–1054.
- Nagaraj B, Krishnamurthy N, Liny P, et al. Biosynthesis of gold nanoparticles of Ixora coccinea flower extract & their antimicrobial activities. Int J Pharma Bio Sci. 2011;2:557–565.
- Deshpande K, Mukasyan A, Varma A. Direct synthesis of iron oxide nanopowders by the combustion approach: reaction mechanism and properties. Chem Mater. 2004;16:4896–4904.
- Sana SS, Singh RP, Sharma M, et al. Biogenesis and application of nickel nanoparticles: a review. Curr Pharm Biotechnol. 2021;22:808–822.
- Laokul P, Amornkitbamrung V, Seraphin S, et al. Characterization and magnetic properties of nanocrystalline CuFe2O4, NiFe2O4, ZnFe2O4 powders prepared by the aloe vera extract solution. Curr Appl Phys. 2011;11:101–108.
- Kar A, Ray AK. Synthesis of nano-spherical nickel by templating hibiscus flower petals. J Nanosci Nanotechnol. 2014;2:17–20.
- Morozov YG, Belousova O, Kuznetsov M. Preparation of nickel nanoparticles for catalytic applications. Inorg Mater. 2011;47:36–40.
- Wu X, Xing W, Zhang L, et al. Nickel nanoparticles prepared by hydrazine hydrate reduction and their application in supercapacitor. Powder Technol. 2012;224:162–167.
- Kumar R, Singh RK, Alaferdov AV, et al. Rapid and controllable synthesis of Fe3O4 octahedral nanocrystals embedded-reduced graphene oxide using microwave irradiation for high performance lithium-ion batteries. Electrochim Acta. 2018;281:78–87.
- Devi N, Sahoo S, Kumar R, et al. A review of the microwave-assisted synthesis of carbon nanomaterials, metal oxides/hydroxides and their composites for energy storage applications. Nanoscale. 2021;13:11679–11711.
- Sahoo S, Kumar R, Joanni E, et al. Advances in pseudocapacitive and battery-like electrode materials for high performance supercapacitors. J Mater Chem A. 2022;10:13190–13240.
- Pandian CJ, Palanivel R, Dhananasekaran S. Green synthesis of nickel nanoparticles using Ocimum sanctum and their application in dye and pollutant adsorption. Chin J Chem Eng. 2015;23:1307–1315.
- Ahmed AI, Yadav DR, Lee YS. Applications of nickel nanoparticles for control of Fusarium wilt on lettuce and tomato. Int J Innov Res Sci Eng Technol. 2016;5:7378–7385.
- Jaji N-D, Lee HL, Hussin MH, et al. Advanced nickel nanoparticles technology: from synthesis to applications. Nanotechnol Rev. 2020;9:1456–1480.
- Moavi J, Buazar F, Sayahi MH. Algal magnetic nickel oxide nanocatalyst in accelerated synthesis of pyridopyrimidine derivatives. Sci Rep. 2021;11:1–14.
- Rossi LM, Quach AD, Rosenzweig Z. Glucose oxidase–magnetite nanoparticle bioconjugate for glucose sensing. Anal Bioanal Chem. 2004;380:606–613.
- Thema F, Manikandan E, Gurib-Fakim A, et al. Single phase Bunsenite NiO nanoparticles green synthesis by Agathosma betulina natural extract. J Alloys Compd. 2016;657:655–661.
- Bhaskar S, Sinha A, Banach M, et al. Cytokine storm in COVID-19 – immunopathological mechanisms, clinical considerations, and therapeutic approaches: the REPROGRAM consortium position paper. Front Immunol. 2020;11:1648.
- Vasudeo K, Pramod K. Biosynthesis of nickel nanoparticles using leaf extract of coriander. Biotechnol Ind J. 2016;12:1–6.
- Lingaraju K, Naika HR, Nagabhushana H, et al. Biosynthesis of nickel oxide nanoparticles from Euphorbia heterophylla (L.) and their biological application. Arab J Chem. 2020;13:4712–4719.
- Kazmi STB, Naz I, Zahra SS, et al. Phytochemical analysis and comprehensive evaluation of pharmacological potential of Artemisia brevifolia Wall. ex DC. Saudi Pharm J. 2022;30:793–814.
- Mohammed IA, Ahmed M, Ikram R, et al. Synthesis of 1, 3-benzoxazines based on 2, 4, 4-trimethyl-7, 2’, 4'-trihydroxy Flavan: antibacterial, anti-inflammatory, cyclooxygenase-2 inhibition and molecular modelling studies. Lett Drug Des Discovery. 2019;16:58–65.
- Ahmed M, Qadir MA, Shafiq MI, et al. Synthesis, characterization, biological activities and molecular modeling of Schiff bases of benzene sulfonamides bearing curcumin scaffold. Arab J Chem. 2019;12:41–53.
- Khalafi T, Buazar F, Ghanemi K. Phycosynthesis and enhanced photocatalytic activity of zinc oxide nanoparticles toward organosulfur pollutants. Sci Rep. 2019;9:1–10.
- Buazar F. Impact of biocompatible nanosilica on green stabilization of subgrade soil. Sci Rep. 2019;9:1–9.
- Rezazadeh NH, Buazar F, Matroodi S. Synergistic effects of combinatorial chitosan and polyphenol biomolecules on enhanced antibacterial activity of biofunctionalized silver nanoparticles. Sci Rep. 2020;10:1–13.
- Safat S, Buazar F, Albukhaty S, et al. Enhanced sunlight photocatalytic activity and biosafety of marine-driven synthesized cerium oxide nanoparticles. Sci Rep. 2021;11:1–11.
- Rahdar A, Aliahmad M, Azizi Y. Nio nanoparticles: synthesis and characterization. J Nanostruct. 2015;5:145–151.
- Zhang Y, Mahdavi B, Mohammadhosseini M, et al. Green synthesis of NiO nanoparticles using Calendula officinalis extract: chemical charactrization, antioxidant, cytotoxicity, and anti-esophageal carcinoma properties. Arab J Chem. 2021;14:103105.
- Reddy BR, Harish G, Reddy CS, et al. Synthesis and characterization of Cu doped NiO nanoparticles. Int J Mod Eng Res. 2014;4:62–66.
- Sepahvand M, Buazar F, Sayahi MH. Novel marine-based gold nanocatalyst in solvent-free synthesis of polyhydroquinoline derivatives: green and sustainable protocol. Appl Organomet Chem. 2020;34:e6000.
- Mustajab M, Ikram M, Haider A, et al. Promising performance of polyvinylpyrrolidone-doped bismuth oxyiodide quantum dots for antibacterial and catalytic applications. Appl Nanosci. 2022;12:2621–2633.
- Ikram M, Haider A, Imran M, et al. Facile synthesis of starch and tellurium doped SrO nanocomposite for catalytic and antibacterial potential: in silico molecular docking studies. Int J Biol Macromol. 2022;221:496–507.
- Haider A, Ijaz M, Ali S, et al. Green synthesized phytochemically (Zingiber officinale and Allium sativum) reduced nickel oxide nanoparticles confirmed bactericidal and catalytic potential. Nanoscale Res Lett. 2020;15:1–11.
- Ikram M, Bashir Z, Haider A, et al. Bactericidal action and molecular docking studies of catalytic Cu-doped NiO composited with cellulose nanocrystals. Int J Biol Macromol. 2022;195:440–448.
- Hussain S, Ali S, Shahzadi S, et al. Heterobimetallic complexes containing Sn(IV) and Pd(II) with 4-(2-hydroxyethyl)piperazine-1-carbodithioic acid: synthesis, characterization and biological activities. Cogent Chem. 2015;1:1029038.
- Mariam AA, Kashif M, Arokiyaraj S, et al. Bio-synthesis of NiO and Ni nanoparticles and their characterization. Dig J Nanomater Biostruct. 2014;9:1007–1019.
- Bibi I, Kamal S, Ahmed A, et al. Nickel nanoparticle synthesis using Camellia Sinensis as reducing and capping agent: growth mechanism and photo-catalytic activity evaluation. Int J Biol Macromol. 2017;103:783–790.
- Pakzad K, Alinezhad H, Nasrollahzadeh M. Green synthesis of Ni@ Fe3O4 and CuO nanoparticles using Euphorbia maculata extract as photocatalysts for the degradation of organic pollutants under UV-irradiation. Ceram Int. 2019;45:17173–17182.
- Huang Y, Zhu C, Xie R, et al. Green synthesis of nickel nanoparticles using Fumaria officinalis as a novel chemotherapeutic drug for the treatment of ovarian cancer. J Exp Nanosci. 2021;16:368–381.
- Din MI, Nabi AG, Rani A, et al. Single step green synthesis of stable nickel and nickel oxide nanoparticles from Calotropis gigantea: catalytic and antimicrobial potentials. Environ Nanotechnol Monit Manag. 2018;9:29–36.
- Kuchekar S, Dhage P, Gaikwad V, et al. Biosynthesis and characterization of nickel nanoparticle using Ocimum sanctum (Tulsi) leaf extract. Chem Sci Trans. 2018;7:696–702.
- Heydari S, Shirmohammadi Aliakbarkhani Z, Hosseinpour Zaryabi M. Photocatalytic degradation of safranin dye from aqueous solution using nickel nanoparticles synthesized by plant leaves. Int J Nanosci Nanotechnol. 2020;16:153–165.
- Sadaa AM, Al Abdullah ZT. Green synthesis of nickel nanoparticles and their application of removal of aliphatic hydrocarbons from crude oil. Iraqi J Sci. 2021:62;4333–4341.
- Chen H, Wang J, Huang D, et al. Plant-mediated synthesis of size-controllable Ni nanoparticles with alfalfa extract. Mater Lett. 2014;122:166–169.
- Angajala G, Ramya R, Subashini R. In-vitro anti-inflammatory and mosquito larvicidal efficacy of nickel nanoparticles phytofabricated from aqueous leaf extracts of Aegle marmelos Correa. Acta Trop. 2014;135:19–26.
- Olajire A, Mohammed A. Green synthesis of nickel oxide nanoparticles and studies of their photocatalytic activity in degradation of polyethylene films. Adv Powder Technol. 2020;31:211–218.
- Din MI, Tariq M, Hussain Z, et al. Single step green synthesis of nickel and nickel oxide nanoparticles from Hordeum vulgare for photocatalytic degradation of methylene blue dye. Inorg Nano-Metal Chem. 2020;50:292–297.
- Habtemariam AB, Oumer M. Plant extract mediated synthesis of nickel oxide nanoparticles. Mater Int. 2020;2:205–209.
- Karunakaran G, Jagathambal M, Van Minh N, et al. Green synthesis of NiFe2O4 spinel-structured nanoparticles using Hydrangea paniculata flower extract with excellent magnetic property. JOM. 2018;70:1337–1343.
- Habtemariam AB, Bekele E. Facile synthesis of nickel oxide nanoparticles using Rhamnus prinoides leaf extract and evaluation of its antibacterial activities. Regen Eng Transl Med. 2022:8;482–488.