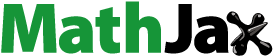
Abstract
In this study, the in vitro DNA-binding interactions of the food/drug additive, 5-hydroxymethylfurfural (HMF) and its major degradant, 5, 5'[oxy-bis(methylene)]bis-2-furfural (OBMF) were investigated. OBMF was synthesized and characterized using IR, NMR and mass spectrometry. Photometric titrations revealed OBMF induced more extensive perturbations in the 258 nm band of DNA and exhibited binding constants that were 5–12-folds higher than those of HMF. The greatest net changes in viscosity of DNA induced by HMF and OBMF were 10.5 and 8.9%, respectively which confirmed both compounds as minor groove binders. Docking revealed that OBMF and HMF bound to the guanine–cytosine regions of minor groove of DNA with global binding energies of −36.36 and −26.12 kcal/mol, respectively. DFT calculations revealed the higher electrophilicity of OBMF contributed to its increased interaction with the negatively charged DNA backbone. There is a need for stricter control of permissible levels of OBMF in food and drug products.
1. Introduction
5-hydroxymethyfurfural (HMF) is a product of the Maillard reaction involving the thermal-assisted conversion of disaccharides [Citation1]. Consequently, HMF is spontaneously generated during the heat processing of several sugar-containing foods such as dried fruits, confectionaries, pastries, bread, ice cream, coffee, etc. The levels of HMF in foods vary widely being dependent on the heat processing time and temperatures [Citation2]. In addition, HMF is deliberately employed as a food additive to impart characteristic flavour and encourage consumer acceptability. As such, widely varying ranges of 6.2–2000mg/kg in coffee, 3.2–703.1 mg/kg in honey and 9–9.5 mg/kg in caramel products have been documented [Citation1–3]. HMF has also been detected in heat-sterilized pharmaceutical parenteral solutions and Chinese herbal medicines [Citation4].
However, despite its ubiquity in many foods and pharmaceutical preparations, uncertainty still prevails with the safety of HMF especially as regards its potential genotoxic and/or mutagenic activity. While its cardio, immuno and hepato-protective activities have been documented, several reports have also suggested its potential toxicity [Citation5–7]. HMF has been reported to be nephrotoxic and an in vivo initiator of colon cancer in rats [Citation8]. In humans, HMF at high concentrations, has been demonstrated to be cytotoxic and irritable to mucous membranes [Citation1]. Studies have also established an association between HMF exposure from drug administration and side effects such as allergies, muscle damage and thrombophlebitis [Citation4,Citation9]. In other studies, the intact compound, though not toxic, was biotransformed to 5-sulphooxymethylfurfural which was genotoxic using in vitro mammalian cell assay models and capable of inducing conformational changes in DNA [Citation8,Citation10]. Accordingly, a recent spectrophotometric investigation of its binding with ct-DNA showed that HMF occupied the minor groove of DNA [Citation11]. The in vitro investigation of the binding of small molecules to DNA offers a simple but powerful tool to elucidate their mechanisms of interaction, improve the rational design of safer congeners and understand how binding could compromise the functions of DNA in controlling the preservation of inheritable genetic material and the biological synthesis of proteins [Citation12–16]. Although the cellular response and processing of DNA damage may or may not result in mutations, the induction of DNA damage following the binding of exogenous substances remains the first step in the process of agent-induced carcinogenesis [Citation17]. Consequently, human population studies have established an increased risk of cancer among individuals with higher-than-control levels of DNA damage events such as covalently bound ligand-DNA adducts and single- and double-DNA strand breaks [Citation18,Citation19].
It is remarkable to note that HMF is only one of several by-products of the Maillard reaction generated during the heat processing of foods and parenteral solutions [Citation2]. Derivatives of HMF including furfural, N-acetyl-lysine-glucose and 5, 5’[oxy-bis(methylene)]bis-2-furfural (OBMF) are co-generated under the same conditions [Citation20]. In particular, the concentrations of HMF and OBMF exist in a dynamic equilibrium during the Maillard reaction and thus, OBMF is often employed as a visual indicator of the synthetic conversion of disaccharides to HMF [Citation2,Citation21]. In addition, HMF is highly unstable undergoing extensive degradation during the prolonged storage of foods and parenteral solutions to yield OBMF among a host of other degradative products. These degradative products, (whose relative levels might vary with batch-to-batch inconsistency in the heat treatment and storage conditions of products) are not often quantified or controlled and are usually overlooked in toxicity profiling of HMF [Citation8,Citation22]. For example, OBMF has been found at levels greater than that of HMF in parenteral solutions and traditional Chinese medicines [Citation4]. Unfortunately, other than an investigation of its immuno-modulatory effects, there is a dearth of studies on the potential effect of OBMF on human health or its contribution to the toxicity liabilities of HMF [Citation4]. We are of the opinion that a better understanding of the toxicity profile of HMF and some of the attendant inconsistencies in its safety assessment might be gained by investigating its major degradant, OBMF. In this particular study, we have focused on the ct-DNA interaction of OBMF and hypothesize that the similarity of its chemical functionalities (with HMF, as shown in Figure ) and the higher number of hydrogen bond donor/acceptor atoms in its side chains might mimic and possibly potentiate its interaction with DNA than HMF.
Figure 1. Chemical structures of (a) 5-hydroxymethylfurfural and (b) 5, 5’[oxy-bis(methylene)]bis-2-furfural.
![Figure 1. Chemical structures of (a) 5-hydroxymethylfurfural and (b) 5, 5’[oxy-bis(methylene)]bis-2-furfural.](/cms/asset/f1d82f86-1303-4639-be0e-9f0067790ed3/tusc_a_2183705_f0001_oc.jpg)
The aim of this study was, therefore, to characterize the binding of OBMF and HMF with ct-DNA using UV–visible spectroscopy, DNA viscosity studies, DFT calculations and molecular docking approaches. A comparison of the binding strengths and mechanisms employed by OBMF and HMF was made as well as the structural considerations explaining the observed trends.
2. Experimental
2.1. Materials and methods
All reagents were sourced from Sigma-Aldrich except 5-hydroxymethyfurfural and activated alumina which were purchased from AK Scientific and BDH UK, respectively. Stock solutions of ct-DNA, HMF and OBMF were prepared in a 10 mM Tris-HCl buffer solution (pH 7.4).
For the DNA solutions, the ratio of the absorbance at 260 and 280 nm was 2.01, indicating that DNA was sufficiently free from protein. A working concentration of 30 µM DNA was prepared from properly diluted samples using a molar absorption coefficient of 6600 M−1 cm−1 at 260 nm.
2.2. Instrumentation
Synthesis was monitored with TLC on pre-coated silica gel GF254 plates using water: ethanol: ethyl acetate (3:6:1) and propan-1-ol: ethyl acetate: water (6:2:2) as mobile phases. The percentage purity of the product was determined using HPLC (Agilent Technologies 1290 Infinity) hyphenated to a mass spectrometer (Agilent Infinity G6125B). Chromatographic conditions included gradient elution on a C-18 column using a mobile phase of 0.1% formic acid in water: 0.1% acetic acid in methanol (70:30). The IR spectrum of the product was acquired using the KBr method with a Buck Scientific M530 IR spectrophotometer. The NMR data of the product were obtained at room temperature using a Nanalysis 60PRO Multinuclear NMR spectrometer and the chemical shifts in ppm were recorded with respect to the residual protons of DMSO-d6 solvent. Absorption spectra of ctDNA-ligand titrations were recorded using a UV–visible spectrophotometer with a 1 cm path length (Spectroquant Pharo 300).
2.3. Synthesis and spectroscopic characterization of OBMF
OBMF was synthesized via the thermal dehydration of HMF with slight modifications [Citation23]. Dry DMSO was prepared by drying analar grade DMSO over freshly activated alumina for 24 h. A reaction mixture comprising 11:1 molar ratios of 5-hydroxymethylfurfural (5.45 g, 43.21 mmol) and dry DMSO (0.29 mL, 3.92 mmol) was then refluxed at 155°C for 90 min. The reaction mixture was cooled in an ice bath after which 50 mL of distilled water was added and the mixture refluxed for an additional 30 min. The reaction mixture was thereafter filtered while it was hot and the filtrate was allowed to slowly cool to room temperature. The yellow product was filtered from the solution and dried in a desiccator.
Colour: yellow product; mp: 111–113°C, Yield: 32.7%; IR (KBr) cm−1 2690.47–2782.47 (aldehyde H), 1600.0 (C = O), 1289.60 (C–O); 1H NMR (DMSO-d6 60 MHz)
9.54 (s, 2H CHO), 7.45 (d, J = 3.6 Hz, 2H), 6.70 (d, J = 3.6 Hz, 2H), 4.58 (s, 4H); 13C NMR (DMSO-d6 60 MHz)
178.78 (C-1 and C-12), 157.72 (C-5 and C-8), 152.81 (C-2 and C-11), 123.99 (C-3 and C-10), 112.66 (C-4 and C-9), 64.19 (C-6 and C-7); Negative-ion ESI: m/z molecular ion peak not found, Calculated M 234.2.
2.4. Spectrophotometric titrations of ligands with ct-DNA
Photometric titration was carried out by keeping the concentration of ct-DNA constant and adding a fixed volume of the different working solutions of the ligand to obtain values of 0, 0.25, 0.5, 0.75, 1.0, 1.5 and 2.0. The reaction mixtures were incubated at 25°C for 30 min after which the UV–visible spectra between 190 and –800 nm were obtained. For each data acquisition, baseline correction was made with the appropriate ligand concentration.
2.5. DNA viscosity studies
The DNA concentration was kept constant at 7.5 × 10−5 M, while the concentrations of the ligands were varied to obtain final ratios of 0, 0.05, 0.1, 0.2, 0.4 and 0.5. All measurements were carried out in duplicates using a digital micro-viscometer maintained at 25°C. The mean values of replicate determinations were treated as a plot of the relative viscosity
as against
ratios, where
and
refer to the viscosities of DNA in the absence and presence of ligands, respectively [Citation24].
2.6. Density function theory calculations
The geometrical parameters of HMF and OBMF in the ground state were optimized using the DFT-B3LYP hybrid functional and the 6-311G**(d,p) basis set [Citation25]. The vibrational frequencies of the optimized geometries were also evaluated using the same methods to confirm that the stationary state was achieved. All calculations were done with the GAMESS program and visualized with MacMolPt molecular visualizer [Citation26].
2.7. Docking study
The crystallographic structure of ds-DNA (PDB ID: 1BNA) was downloaded from the RCSB protein data bank [Citation27]. The PDB files of HMF, OBMF and DNA were subjected to the PatchDock server for docking followed by refinement with the FireDock to obtain the ten best refined complex solutions with respect to the global minima binding energy [Citation28–30]. Post-docking analysis of the conformations and stability of the best complex structures were done using the Biovia Discovery Studio Visualizer 2020 software.
3. Results and discussion
3.1. Chemistry
OBMF was successfully synthesized by the thermal dehydration of HMF. The general scheme of the reaction is depicted in Scheme 1. Thin layer chromatography analysis using water: ethanol: ethyl acetate (3:6:1) and propan-1-ol: ethyl acetate: water (6:2:2) as mobile phases revealed Rf values of 0.73 and 0.70, respectively for HMF, while Rf values of 0.65 and 0.62, respectively, were recorded for OBMF. The liquid chromatographic analysis of the yellow product revealed a percent content of 95.72%w/w.
The synthesis of ethers via the thermal-assisted removal of a molecule of water from the corresponding alcohols has been employed for aromatic and allylic ethers [Citation31,Citation32]. The process typically proceeds via a resonance-stabilized carbocation with yields of less than 50%. The melting point and NMR data of the product were identical to those described in the literature [Citation4].
3.2. UV–visible spectroscopic titrations of ligands with ct-DNA
As a result of the low symmetry and several heteroatom lone pairing of its nucleobases, the UV–visible spectrum of DNA is influenced largely by the absorbance transitions of its nucleobases which gives a predominant broad band at around 258 nm [Citation33]. The position and absorptivity of this band are reflective of the AT/GC base content and the secondary structure of DNA. Thus, measurements of changes in this band make UV–visible spectroscopy a very useful tool in the study of the mechanisms and stability of ligand-DNA interactions [Citation12]. The changes in the electronic absorption spectra of ct-DNA with increasing mole ratios of the investigated ligands were, therefore, monitored and are depicted in Figure . The 258 nm band of DNA showed hypochromic changes with increasing mole ratios of HMF. This was accompanied by a slight blue shift of the band. In contrast, increasing mole ratios of OBMF led to an initial increase in absorptivity of the 258 nm band
) followed by a progressive decrease in absorptivity at higher concentrations of OBMF. More pronounced changes including a blue shift
of the 258 nm band and a disappearance of the 202 nm band could also be observed with higher concentrations of OBMF.
Figure 2. Electronic absorption spectra of ct-DNA with increasing mole ratios of (a) HMF and (b) OBMF.

Ligands interact non-covalently with DNA using two predominant modes including intercalation in which planar heteroatomic molecules slide in between stacked bases and minor groove binding in which aromatic compounds employ hydrophobic interactions, electrostatic and hydrogen bonding [Citation34,Citation35]. A third mode of interaction that involves the electrostatic binding of mono-cationic chemical species is very much less prominent and weak without intercalation or groove binding [Citation13,Citation15]. The intercalative mechanism is accompanied by an overlap of the π orbitals of the intercalating molecule and DNA base pairs leading to extensive conjugation and a shift of the 258 nm band of DNA to higher wavelengths [Citation12]. In addition, the transition probabilities of the coupling orbitals are reduced causing a decrease in absorptivity. Thus, concomitant bathochromic and hypochromic changes in the 258 nm band of DNA are indicative of an intercalative mechanism of interaction by a ligand [Citation35,Citation36]. Consequently, the observed hypsochromic shift in the 258 nm band of DNA following the addition of increasing concentrations of HMF and OBMF suggested that the binding of both ligands is not by the intercalative mechanism. This is further supported by the observation that the absorptivity of the 258 nm band of DNA was higher, with respect to , at all the test concentrations of OBMF. The exact mode of interaction between the ligands and DNA will be further confirmed by thermodynamic studies, DNA viscosity measurements and molecular modelling.
The binding constants of HMF and OBMF to DNA were determined by a double reciprocal method based on Equation (1) which is the most suitable for the determination of energies of the anticipated order [Citation12].
(1)
(1) where
and A are the absorbance values of DNA in the absence and presence of different concentrations of the ligands, respectively.
is the final absorbance of the ligand–protein complex.
The double reciprocal plots for the binding of the two compounds with DNA are depicted in Figure .
The binding constants were determined from the ratio of the intercept to the slope of the plots as depicted in Table . The results showed that when compared with HMF, the degradant OBMF exhibited a 5–12-fold increase in binding affinity for DNA at the two temperatures investigated, indicating increased stability of the OBMF-DNA complex in comparison with the HMF-DNA system.
Table 1. Binding and thermodynamic energy changes associated with the binding of HMF and OBMF with DNA.
The thermodynamic parameters associated with the binding were determined using a modified Van’t Hoff equation for two temperature levels. The overall free energy change was calculated with Equation (2), while the enthalpic and entropic contributions were estimated using Equations (3) and (4), respectively.
(2)
(2)
(3)
(3)
(4)
(4)
The thermodynamic energy changes associated with the formation of HMF-DNA and OBMF-DNA complexes are also shown in Table .
The negative free energy changes associated with the formation of the two complexes indicate that the binding is spontaneous, while the reduction of the binding constants with increasing temperatures is indicative of an exothermic process. The thermodynamic consideration of the contribution of enthalpy and entropy to the overall free energy changes can be used to decipher the predominant mode of interaction between ligands and macromolecules. According to previous studies, combined positive enthalpy and entropy changes delineate hydrophobic interactions, while a combination of negative enthalpy and entropy changes indicate hydrogen bonding and Van der Waals interactions [Citation37]. The negative ΔH and ΔS values associated with the binding of HMF and OBMF with ct-DNA thus showed that the formation and stabilization of the resultant protein complexes are driven by reversible intermolecular forces of interaction including hydrogen bonding and Van der Waals forces.
3.3. DNA viscosity studies
In the absence of NMR or x-ray crystallography, hydrodynamic studies such as the measurement of DNA viscosity with increasing concentrations of bound ligand have been identified as one of the most accurate methods for determining the binding mode of a ligand [Citation12]. The intercalative mechanism requires a separation of the stacked DNA base pairs for subsequent insertion of the bound ligand, thereby leading to a lengthening of the DNA helix and an increase in its viscosity. Similarly, partial non-classical intercalating ligands will cause bending or distortion in the DNA helix, thereby reducing its length and viscosity. In contrast, DNA minor groove binders employ weak reversible forces of interaction and consequently, cause little or no changes in the relative viscosity of DNA [Citation34,Citation38].
As shown in Figure , the relative viscosity of DNA did not show any appreciable changes with increasing concentrations of HMF or OBMF.
The greatest net change in the viscosity of DNA induced by HMF and OBMF was 10.5 and 8.9%, respectively. This result was similar to a net change of about 15% recorded with levetiracetam, another DNA minor groove binder, at the same mole ratio [Citation12]. In contrast, increases of 50–67% in the viscosity of DNA are common with classical intercalating mechanisms [Citation34]. The results, therefore, confirmed that HMF and OBMF bind to the minor groove of DNA. Although an earlier study equally identified minor groove binding as the mode of interaction of HMF with DNA [Citation11], our study represents the first on the binding characteristics of OBMF with DNA.
3.4. Analysis of conceptual DFT parameters
The computed optimized geometries and contour plots of the electron density distribution of HOMO and LUMO for HMF and OBMF, respectively, at B3LYP/6-311G (d,p) level are depicted in Figure . The energy levels of the HOMO, LUMO and global reactivity descriptors for HMF and OBMF are also shown in Table .
Figure 5. Contour plots of the electron density distribution of HOMO and LUMO of (a) HMF and (b) OBMF.

Table 2. The electronic properties and chemical reactivity of ligands calculated at B3LYP/6-311G (d,p) level.
The energy calculations showed small energy gaps and feasible energy transitions from HOMO to LUMO in both ligands. However, the positive chemical potential value of HMF is due to its poor stability and spontaneous degradation in the presence of moisture and light [Citation39]. In contrast, OBMF showed higher kinetic stability and is thus capable of more persistent intermolecular interactions with DNA. In addition, the decreased tendency of OBMF to donate electrons (evidenced by its higher ionization potential) and its higher global electrophilicity index could contribute to its more extensive interaction with the negatively charged ribose phosphate chain of DNA [Citation40].
3.5. Molecular docking studies
Molecular docking of HMF and OBMF with ds-DNA using PatchDock returned 56 and 218 protein complex solutions, respectively. Following optimization and refinement using FireDock, the ranking of the ten best solution complexes, based on global energy values and the contributory roles of Van der Waals forces and atomic contact energy, are depicted in Table for HMF and Table for OBMF.
Table 3. Docking energies of HMF with DNA by FireDock.
Table 4. Docking energies of OBMF with DNA by FireDock.
As shown in Figures and , molecular docking confirmed that both HMF and OBMF were bound to the minor groove of ct-DNA, respectively. The results showed that HMF interacted with DNA mainly via Van der Waals, pi-pi stacked and hydrogen bond interactions occurring between HMF and G and C bases (Figure (a)). In particular, the HMF-DNA complex was stabilized by two hydrogen bond interactions occurring between the furan oxygen of HMF and the hydrogen atoms of the primary amino group of two guanine bases on chain B (DG16 and DG10).
Figure 6. HMF-ctDNA interaction showing (a) docking pose in minor grooves (b) surrounding nucleobases (c) intermolecular forces.

Figure 7. OBMF-ctDNA interaction showing (a) docking pose in minor grooves (b) surrounding nucleobases (c) intermolecular forces.

Scheme 1. Thermal dehydration of 5-hydroxymethylfurfural yields 5, 5’[oxy-bis(methylene)]bis-2-furfural.
![Scheme 1. Thermal dehydration of 5-hydroxymethylfurfural yields 5, 5’[oxy-bis(methylene)]bis-2-furfural.](/cms/asset/1897b2be-52bd-4b15-abbf-fb295e160d50/tusc_a_2183705_f0008_oc.jpg)
In contrast, hydrophobic interactions including pi-pi T-shaped, pi-donor bonds between OBMF and G and C bases were the most prominent intermolecular forces driving its binding to DNA (Figure ). Probable hydrogen bonding could also exist between the methylene group of the OBMF side chain and the cytosine base (DC15 of chain B).
Thus, molecular docking simulations revealed that HMF and OBMF were bound to the minor groove of DNA. This is in agreement with thermodynamic and DNA viscosity studies which indicated that binding was not by intercalating mechanisms. Both HMF and OBMF interacted with DNA via a combination of Van der Waals forces, hydrogen bonding and hydrophobic interactions including pi-pi stacked, pi-pi T-shaped and pi-donor bonds. This is consistent with minor groove binders which usually form DNA complexes that are stabilized by hydrophobic interactions and Van der Waals forces [Citation41].
Docking studies also showed that the global binding energy value of OBMF with DNA was higher than that of HMF. This implies OBMF can induce more persistent alterations to native DNA as the OBMF-DNA complex is better stabilized than HMF-DNA. The differences in the binding affinities of HMF and OBMF can be explained by the consideration of their chemistry on the binding mechanisms. Minor groove binders are mostly aromatic compounds connected by side chains that permit freedom of movement and torsion [Citation29]. The flexibility of the aromatic structures allows for an easy fit into the minor groove of DNA. It is, therefore, anticipated that OBMF with its higher C/H ratio than HMF showed a more extensive network and range of hydrophobic interactions with DNA resulting in a higher global energy value. In addition, the free rotation along the sigma bonds of the ether bridge between the two furan rings in OBMF increases its number of possible conformations and consequently, its ability to adopt a curved shape that is complimentary to the DNA minor groove. Expectedly, following the refinement of the side-chain conformations and rigid-body orientations of the protein complex solutions with FireDock, 218 transformations were possible with OBMF as against only 56 with HMF [Citation30].
4. Conclusion
Studies have reported a widespread occurrence of HMF and its degradant OBMF in heat-processed sugar-containing foods and parenteral solutions. However, while the genotoxicity of HMF has been differently investigated, the safety assessment of OBMF remains neglected. A comparison of the DNA-binding interactions of HMF and OBMF using UV–visible spectroscopic, viscometric, DFT and docking studies was carried out. Based on calculated binding constants, docking energies and global chemical reactivity descriptors, OBMF exhibited a stronger binding affinity for DNA, formed better-stabilized DNA complexes and could induce more persistent alterations to native DNA. This implies that consumers of HMF-containing products might be at increased risk because of the cumulative toxicity effects of the co-present OBMF. There is, therefore, a need for stricter regulatory control of permissible levels of OBMF in food and drug products.
Acknowledgements
The authors gratefully acknowledge the assistance of the technical staff of the Multidisciplinary laboratory of the University.
Disclosure statement
No potential conflict of interest was reported by the author(s).
References
- Severin I, Dumont C, Jondeau-Cabaton A, et al. Genotoxic activities of the food contaminant 5-hydroxymethylfurfural using different in vitro bioassays. Toxicol Lett. 2010;192:189–194.
- Morales FJ. Hydroxymethylfurfural (HMF) and related compounds. In: Stadler R, Lineback DR, editors. Process-induced food toxicants: occurrence, formation, mitigation and health risks. New Jersey: John Wiley and Sons, Inc; 2009. p. 135–179.
- Abraham K, Gürtler R, Berg K, et al. Toxicology and risk assessment of 5-Hydroxymethylfurfural in food. Mol Nutr Food Res. 2011;55:667–678.
- Lin N, Liu T, Lin L, et al. Comparison of in vivo immunomodulatory effects of 5-hydroxymethylfurfural and 5, 5′-oxydimethylenebis (2-furfural). Regul Toxicol Pharmacol. 2016;81:500–511.
- Wölkart G, Schrammel A, Koyani CN, et al. Cardioprotective effects of 5-hydroxymethylfurfural mediated by inhibition of L-type Ca2+ currents. Br J Pharmacol. 2017;174:3640–3653.
- Matzi V, Lindenmann J, Muench A, et al. The impact of preoperative micronutrient supplementation in lung surgery. A prospective randomized trial of oral supplementation of combined α-ketoglutaric acid and 5-hydroxymethylfurfural. Eur J Cardio-Thoracic Surg. 2007;32:776–782.
- Li W, Qu XN, Han Y, et al. Ameliorative effects of 5-hydroxymethyl-2-furfural (5-HMF) from Schisandra chinensis on alcoholic liver oxidative injury in mice. Int J Mol Sci. 2015;16:2446–2457.
- Arribas-Lorenzo G, Morales FJ. Estimation of dietary intake of 5-hydroxymethylfurfural and related substances from coffee to Spanish population. Food Chem Toxicol. 2010;48:644–649.
- Shapla UM, Solayman M, Alam N, et al. 5-Hydroxymethylfurfural (HMF) levels in honey and other food products: effects on bees and human health. Chem Cent J. 2018;12:35.
- Durling LJK, Busk L, Hellman BE. Evaluation of the DNA damaging effect of the heat-induced food toxicant 5-hydroxymethylfurfural (HMF) in various cell lines with different activities of sulfotransferases. Food Chem Toxicol. 2009;47:880–884.
- Zhou Z, Hu X, Zhang G, et al. Exploring the binding interaction of Maillard reaction by-product 5-hydroxymethyl-2-furaldehyde with calf thymus DNA. J Sci Food Agric. 2019;99:3192–3202.
- Shahabadi N, Hadidi S. Spectroscopic studies on the interaction of calf thymus DNA with the drug levetiracetam. Spectrochim Acta Part A Mol Biomol Spectrosc. 2012;96:278–283.
- Luo YJ, Wang BL, Kou SB, et al. Assessment on the binding characteristics of dasatinib, a tyrosine kinase inhibitor to calf thymus DNA: insights from multi-spectroscopic methodologies and molecular docking as well as DFT calculation. J Biomol Struct Dyn. 2020;38:4210–4220.
- Shi JH, Zhou KL, Lou YY, et al. Multi-spectroscopic and molecular docking studies on the interaction of darunavir, a HIV protease inhibitor with calf thymus DNA. Spectrochim Acta – Part A Mol Biomol Spectrosc. 2018;193:14–22.
- Shi JH, Liu TT, Jiang M, et al. Characterization of interaction of calf thymus DNA with gefitinib: spectroscopic methods and molecular docking. Photobiol B Biol. 2015;147:47–55.
- Shi JH, Chen J, Wang J, et al. Binding interaction between sorafenib and calf thymus DNA: spectroscopic methodology, viscosity measurement and molecular docking. Spectrochim Acta – Part A Mol Biomol Spectrosc. 2015;136:443–450.
- DeMarini DM. The role of genotoxicity in carcinogenesis. In: Baan R, Stewart B, Straif K, editors. Tumour site concordance and mechanisms of carcinogenesis. Lyon: International Agency for Research on Cancer; 2019. p. 107–116.
- Wu HC, Kehm R, Santella RM, et al. DNA repair phenotype and cancer risk: a systematic review and meta-analysis of 55 case–control studies. Sci Rep. 2022;12:3405.
- Katerji M, Duerksen-Hughes PJ. DNA damage in cancer development: special implications in viral oncogenesis. Am J Cancer Res. 2021;11(11):3956–3979.
- Li C, Wang H, Juárez M, et al. Structural characterization of amadori rearrangement product of glucosylated Nα-acetyl-lysine by nuclear magnetic resonance spectroscopy. Int J Spectrosc. 2014;2014:1–6. 789356. doi:10.1155/2014/789356
- Kuster BFM. 5-Hydroxymethylfurfural (HMF). A review focussing on its manufacture. Starch-Stärke. 1990;42:314–321.
- Lewkowski J. Synthesis, chemistry and applications of 5-hydroxymethyl-furfural and its derivatives. Arkivoc. 2001;1:17–54.
- Larousse C, Rigal L, Gaset A. Synthesis of 5,5′-oxydimethylenebis (2-furfural) by thermal dehydration of 5-hydroxymethyl-2-furfural in the presence of dimethylsulfoxide. J Chem Technol Biotechnol. 1992;53:111–116.
- Reddy PR, Rajeshwar S, Satyanarayana B. Synthesis, characterization of new copper (ii) Schiff base and 1,10 phenanthroline complexes and study of their bioproperties. J Photochem Photobiol B Biol. 2016;160:217–224.
- Sharma D, Ojha H, Pathak M, et al. Spectroscopic and molecular modelling studies of binding mechanism of metformin with bovine serum albumin. J Mol Struct. 2016;1118:267–274.
- Bode B, Gordon M. Macmolplt: a graphical user interface for GAMESS. J Mol Graph Model. 1998;16:133–138.
- Wani TA, Alsaif N, Bakheit AH, et al. Interaction of an abiraterone with calf thymus DNA: investigation with spectroscopic technique and modelling studies. Bioorg Chem. 2020;100:103957.
- Schneidman-Duhovny D, Inbar Y, Nussinov R, et al. PatchDock and SymmDock: servers for rigid and symmetric docking. Nucleic Acid Res. 2005;33:W363–W367.
- Teoh TC, Salmah I, Tang JM. Molecular dynamics and docking of biphenyl: a potential attachment inhibitor for HIV-1 gp120 glycoprotein. Trop J Pharm Res. 2014;13:339–346.
- Mashiach E, Schneidman-Duhovny D, Andrusier N, et al. FireDock: a web server for fast interaction refinement in molecular docking. Nucleic Acids Res. 2008;36:W229–W232.
- Hashim A, Poulose V, Thiemann T. One pot o-alkylation/Wittig olefination of hydroxybenzaldehydes in DMSO. Chem Proc. 2021;3:99.
- Kazemia M, Noori Z, Kohzadi H, et al. A mild and efficient procedure for the synthesis of ethers from various alkyl halides. Chem . Commun. 2013;1:43–50.
- Farrell N, Kellett SA, Farrell NP, et al. Molecular methods for assessment of non-covalent metallodrug–DNA interactions. Chem Soc Rev. 2019;48:971.
- Ashok A, Banerjee S, Anand N, et al. Spectroscopic and viscometric determination of DNA-binding modes of some bioactive dibenzodioxins and phenazines. Biochem Biophys Reports. 2019;18:100629.
- Long EC, Barton JK. On demonstrating DNA intercalation. Acc Chem Res. 1990;23:271–273.
- Łączkowski KZ, Anusiak J, Switalska M, et al. Synthesis, molecular docking, ctDNA interaction, DFT calculation and evaluation of antiproliferative and anti-Toxoplasma gondii activities of 2,4-diaminotriazine-thiazole derivatives. Med Chem Res. 2018;27:1131–1148.
- Adegoke OA, Ghosh M, Jana A, et al. Studies of the interactions of 4-carboxyl-2,6-dinitrophenylazohydroxynaphthalenes with CT-DNA in aqueous medium. J Mol Liq. 2012;174:17–25.
- Mehran S, Rasmi Y, Karamdel HR, et al. Study of the Binding Interaction between Wortmannin and Calf Thymus DNA : Multispectroscopic and Molecular Docking Studies. Evidence-Based Compimentary Altern Med. 2019;2019:4936351.
- Deghady A, Hussein R, Alhamzani A, et al. Density functional theory and molecular docking investigations of the chemical and antibacterial activities for 1-(4-Hydroxyphenyl)-3-phenylprop-2-en-1-one. Molecules. 2021;26:3631.
- Alsaedi S, Babgi BA, Abdellatif MH, et al. Effect of net charge on DNA-binding, protein-binding and anticancer properties of copper (I) phosphine-diimine complexes. J Inorg Organomet Polym Mater. 2021;31:3943–3952.
- Mišković K, Bujak M, Baus Lončar M, et al. Antineoplastic DNA-binding compounds: intercalating and minor groove binding drugs. Arh Hig Rada Toksilkol. 2013;64:593–602.