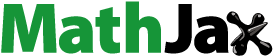
Abstract
The present Net-House Photobioreactor (NHPBR), which has a final capacity of 500 liters, was developed for the purpose of cultivating the Chlorella vulgaris by employing vinasse waste as a source of organic carbon and certain minerals in an effort to lower the cost of production. The growth unit consisted of one reservoir tank carrying 4 Plexi-glass ponds. For each plate, growth and biochemical composition were routinely evaluated to assess the precipitation and growth. Our data revealed no differences in the biomass and chemical constituents in the running algal slurry. However, differences were observed in the precipitated algal biomass for each plate, with the reservoir (CR) and the lower plate (P1) forming the greatest precipitation in terms of dry weight and mat thickness, respectively. The biochemical composition of the harvested biomass was 51.0% crude protein, 13.4% total carbohydrates, 12.1% lipids, and 7.2% ash.
1. Introduction
Biological CO2 mitigation coupled with biomass production through microalgae cultivation has attracted much attention in recent years as a strategic alternative that associates both environmental and economic interests [Citation1]. The rate of carbon uptake is limited by the metabolic activity of microalgae, which is in turn limited by photosynthetic efficiency. In theory, microalgae can use up to 9% of the incoming solar energy to produce 280 tons of dry biomass ha−1 year−1, while sequestering about 513 tons of CO2 [Citation2]. Hirata et al. [Citation3] cultivated Chlorella vulgaris in 3% CO2 for 8 days and achieved a carbon dioxide fixation of 865 mg CO2 L−1day−1. This represents around 1.7 times more carbon dioxide fixated than that estimated by Bilanovic et al. [Citation2], considering a liquid column depth of 15 cm. Thus, carbon dioxide fixation quantifies mainly in terms of biomass production [Citation4].
For microalgal applications in CO2 bio-fixation, and energy production, the tolerance to CO2, biomass production, and fatty acid content of microalgae are of great importance. Several microalgal species have been studied for CO2 sequestration, such as Chlorella kessleri, Scenedesmus obliquus [Citation5], Chlorella vulgaris [Citation6], Dunaliella tertiolecta, Botryococcus braunii, Spirulina platensis [Citation7], Chlorella sp. [Citation8], and Nannochloropsis oculata [Citation9]. Chiu et al. [Citation10] reported the complete inhibition of Chlorella sp. and Nannochloropsis oculata growth when CO2 concentration was above 5%, while De Morais and Costa [Citation5] observed enhancement of Chlorella kessleri, Scenedesmus obliquus, Spirulina sp. and Chlorella vulgaris growth using 6% CO2 enriched air. However, Scenedesmus obliquus grown in wastewater showed efficient growth and lipid accumulation of up to 10% CO2 [Citation11]. Thus, CO2 fixation by microalgae depends mainly on the cultivated species and the cultivation conditions.
Microalgae can fix carbon from different sources including CO2 from the atmosphere, from industrial exhaust gases, and in the form of soluble carbon in many waste streams [Citation12]. The utilization of industrial gaseous and/or liquid residues is receiving increasing attention for microalgae cultivation in view that the major barrier in the industrial cultivation of microalgae is the cost of the synthetic growth medium [Citation13]. Moreover, lipidic waste has been suggested as a carbon source for microalgae cultivation and energy recovery [Citation14].
Using of organic carbon from chemical resources was widely studied and discussed. Heterotrophic biomass production has also been successfully used to study algal metabolites [Citation15]. In this process, microalgae can be grown on organic carbon substrates such as glucose in stirred tank bioreactors or fermenters, where growth is independent of light energy, which allows much simple scale-up possibilities since smaller reactor surface to volume ratios can be used [Citation16]. These systems provide a high degree of growth control and also lower harvesting costs due to the higher cell densities [Citation17]. In addition, the set-up costs are minimal, although the system uses more energy than the autotrophic system because the latter includes the initial production of organic carbon sources via photosynthesis [Citation18].
The concept of hybrid systems might engage algae production more efficiently by increasing the bio-stimulation of organic and industrial carbon-rich waste. Therefore, the present study aimed to evaluate the mixotrophic growth of microalgae in a mixed cultivation system, namely the Net-House Photobioreactor (NHPBR). Vinasse waste was used as an organic carbon source, and metabolites including protein, carbohydrates, sugars, oil, chlorophyll, and carotenoids were analysed.
2. Materials and methods
2.1. Growth conditions
The green microalga Chlorella vulgaris was provided by the Algal Biotechnology Unit, National Research Centre, Cairo, Egypt and cultured mixotrophically using sugarcane waste (vinasse) and atmospheric carbon dioxide as a growth medium [Citation19]. Initially, the strain was laboratory grown to obtain sufficient inoculum [Citation20], which was further inoculated to the reactor. Based on the chemical analysis of waste, 2.0 g.l−1 of vinasse enriched in the (in situ) formulated growth medium to meet the neighbour content of BG-II [Citation21] using tap water for dilution. Vinasse addition was gradually added during the whole cultivation period (21 days after the full unit capacity) own to the growth rate to avoid the dark colour and osmosis effect of the vinasse waste. Sugars fraction and some biochemical constituents of waste are listed in . As shown in growth system is composed of a cement pond with a dimension of 1.2 × 4.0 × 1.0 m (W.L.D) as a reservoir tank filled with algal culture. The culture continuously rose to the upper plate (P1) through the electric pump (1.0 hp), which helped with continuous turbulence in the system. The exceeded culture volume in P1 was filled down by gravity to the lower plate (P2); then to plates P3 and P4. The culture was returned to the reservoir from P4. The technical specifications of the used unit are listed in . White lamps were provided for night illumination at light intensity (2 × 40 watts).
Table 1. Some biochemical properties of vinasse waste.
Table 2. Physical specification of the used cultivation system.
2.2. Growth measurements and biochemical analysis
2.2.1. Biomass profile
Several physical measurements were done to compare the different parts of the cultivation system on the algal biomass profile. Dry weight (g.l−1) was measured daily during the whole cultivation period by filtering a defined volume of algal slurry through a 0.54 µm membrane filter, followed by drying in a circulated oven (105 °C/30 min) and re-weighted and the difference in weights expresses the obtained biomass. In addition, algal mat thickness (mm) formed by algal sedimentation was measured in each plate, then manually harvested, weighted, and attributed to the surface area.
2.2.2. Biochemical analysis
Biochemical macromolecules of algal biomass and vinasse waste including proteins, carbohydrates, and oils were measured in the dry biomass. Pigmentation status (chlorophylls and carotenes) was extracted by 95% DMSO method, followed by absorbance measurement according to Hiscox and Israelstam [Citation22]. Based on the Micro Kjeldahl technique (Ma and Zauzag [Citation23]) including digestion, distillation (Buchii 320 nitrogen distillatory); and titration by 0.01N HCl, crude proteins were determined as total nitrogen (T.N) % × 6.25. Soluble protein (S.P) % was determined as soluble nitrogen (S.N) on the supernatant of algal extracted after homogenization with 15% trichloroacetic acid (TCA). Thus, soluble protein = S.N % × 6.25. True protein was calculated as T.N–S.N [Citation24]. As for amino acids, [Bailey, 1967] method was used after hydrolyzing with 10 ml of 6N HCl in a sealed tube [Citation25]. Total sugars were determined using the white phenol method [Citation26]; while the amount of reducing sugars was carried out by the dinitro salicylic acid (DNS) method using glucose as standard [Citation27]. Non-reduced sugars were calculated as T.S-R.S. Monosaccharides and hydroxymethyl furfural (HMF) in the hydrolysates was analysed using high performance liquid chromatography (HPLC, Agilent, USA). Comparison with analytical curves achieved the quantification using glucose, fructose and sucrose, standards. Oils were Soxhlet extracted by n-hexane: isopropanol mixture (3:2), and trans-methylated and then GC characterized [Citation28]. Macro and micronutrients were determined on the diluted ash solution. Ashing was performed using a furnace muffle at 500°C. After dilution with 2.0N HCl, the adjusted volume was filtered over ashless filter paper [Citation29]. Atomic absorption apparatus (PerkinElmer) was used for nutrient measurements. As for phosphorous, the vanadate method was used for yellow colour formation and measured at 400 nm using a JASCO spectrophotometer.
2.3. Statistical analysis
Experiments were carried out in three replicates, and the results are shown as the means ± standard deviation. One-way analysis of variance (ANOVA) was applied to statistically analyse the data at different probability levels (P) using Statistica version 8.0 (StatSoft Inc., Tulsa, OK, USA).
3. Results and discussion
3.1. Photobioreactor design affecting growth parameters
It is a worthy note that the specifications of the growth unit markedly affected the net algal biomass yield and consequently determine the production costs. Besides, capital costs should be put attention to massive production systems. The current photobioreactor could consider the most simplified type concerning cost, used area with high productivity potential. Comparing different types of production systems in the National Research Centre (NRC) including open plat, helical shape, zigzag shape and open ponds with the introduced photobioreactor (NHPBR) confirms this finding in . As presented in the net yield per used area is much higher compared with other existing units under the same conditions (NRC). Here, one square metre is required for producing 1.0 kg of dried biomass, while an open pond required 4.5 m2.
Table 3. Some technical and economical proprieties of different growth units (NRC-Egypt).
Despite productivity (g.l−1) and nutritional costs especially when all input factors are neutralized, production of the current photobioreactor seems to be the most efficient one. The open pond requires 105 m2 containing 25 m3 of culture volume with a final production of 25 kg of dried algal biomass, whilst NHPBR requires 5.76 m2 containing 5 m3 of culture volume and expectedly produces 5.0 kg of dried algal biomass. Concerning other production units (), the zigzag shape is the neighbour of the open pond. The price for 1 kg of algae ranges from $16.4 for open raceways ponds systems to $33 for closed photobioreactors systems and costs could be lowered to about $4.4 by increasing the sizes to 100 acres with today’s state-of-the-art [Citation30].
As shown in , the cultivation system consists of five different growth ponds. The first is the main reservoir, which was deep and made of concrete, while the other four ponds were shallow and made of fully transparent Plexi-glass which in turn increases the light-exposed volume and area. The deep reservoir effect was first identified by [Citation31], who claimed that the impact stems from the sufficient time for nutrient use within such a structure, which considerably influenced the growth pattern, pigmentation, sedimentation, and formation of the algal mat. Thus, dry weight expressed as g.l−1, pigments, mat thickness, and biochemical composition were determined separately in each pond. It was reported that open ponds are a cost-effective platform for algal production with a higher risk of bio-contamination than photobioreactors [Citation32]. However, Egbo et al. [Citation33] concluded that self-shading, photoinhibition, and high energy requirement for both lighting and mixing are still costly higher. Further challenges concerning photobioreactors are focused on the sustainability of the source(s) of nutrients for microalgae farming, availability of concentrated and uncontaminated carbon dioxide, and ideal means of decontamination. Concerning algae production in the Arab Region, open ponds seem to be more efficient due to high light intensity and temperature, causing high water loss due to evaporation followed by an increase in media salinity. Concerning the current work, increasing surface area to the net volume was performed.
3.2. Dry weight, mat thickness, and pigmentation
The dry weight showed the maximum value of 2.7 g.l−1 in the highly agitated reservoir pond, while tended to be lower in different plates from P1 to P4 . So, the minimal dry weight of 0.91 g.l−1 was recorded in the upper plate (P4). This growth inhibition compared with other plates might attribute to the direct exposure to sunlight with high light irradiation (420 .E), which results in photoinhibition. However, shading of the lower plates decreased this adverse effect, reaching the highest positive effect in the reservoir. Together with the shading effect, algal colony formation at a low turbulence rate in the reservoir resulted in the highest growth. In that context, El-Fouly et al. [Citation31] stated that modification of open plate growth unit increases the microalgal biomass production due to long dark time of algal growth cycle parallel with long light exposed area.
Table 4. Growth parameters of Chlorella vulgaris grown in Net-House photobioreactor (NHPBR) in relation to some unit specifications.
Mat thickness showed the same pattern where the lowest thickness was observed in P4 due to the high turbulence rate by pushing the algal culture from the water pump. Thick mat formation is desirable for easy hand-harvest of algal biomass. At the 9th day, dry weight was 2.88 g.l−1 with growth rate 0.24 g.d−1, comparing to 0.16 g.d−1 at the 14th day [Citation19].
Moderate concentrations of chlorophyll and carotenes were obtained with slight variations. These variations mainly depend on the environmental and technical aspects including light exposed area, turbulence, and culture depth. For this reason, high chlorophyll and carotene contents were observed in the reservoir unit due to low light intensity and low turbulence rate.
Organic carbon used in this case seems to enhance the algal growth due to the high initial carbon feeding beside the accompanied macro- and micro-nutrients. In this regard, El-Sayed [Citation34] found that citrate wastes at 50 ml.l−1 support growth media by high organic carbon and other organic acids which stimulate both vegetative growth and carotenoid accumulation. It is important to note that such wastes contain 0.47% of organic carbon and a C: N ratio of 0.73:1.0. As mentioned previously, in the absence of essential nutrients including nitrogen and phosphorous, increasing organic carbon concentrations also rise the salinity potential of the growth media [Citation19]. The changes in photosynthetic pigments under mixotrophic cultivation vary from one strain to another. For example, there was a significant loss of both chlorophyll a and b in Scenedesmus acutus [Citation35] and Platymonas subcordiformis [Citation36] under mixotrophic growth using acetate. Using organic carbon from chemical resources including organic acids or carbon-rich wastes was widely employed and discussed [Citation37]. Heterotrophic production has also been successfully used for algal biomass production [Citation38]; where growth is independent of light energy, providing much simpler scale-up possibilities since smaller reactor surface-to-volume ratios can be efficiently used [Citation16]. Many algal species are capable of using either the metabolism process (autotrophic or heterotrophic) for growth, meaning that they can perform photosynthesis or rely on organic materials [Citation39]. The ability of mixotrophs to process organic substrates means that cellular growth is not strictly dependent on light, therefore illumination is not a limiting factor for growth [Citation40], as either light or organic carbon substrates can support the growth. Examples of microalgae that display mixotrophic metabolism processes for growth are the cyanobacterium Spirulina platensis, and the green alga Chlamydomonas reinhardtii [Citation41]. Photosynthetic metabolism utilizes light for growth while aerobic respiration uses the organic carbon source [Citation42].
Growth is influenced by the media supplementation with glucose during light and dark cycles; hence, there is less biomass loss during the dark cycle [Citation40]. Among different organic carbon sources, glucose is the most widely used source of organic carbon and it is relatively inexpensive; however, acetate, citrate, and other organics have been suggested as well [Citation43]. However, it is recommended to use carbon-rich wastes to meet the beneficial use and minimize the production costs of microalgal biomass production. In that regard, many algal species are capable to grow autotrophically, heterotrophically, or mixotrophically based on the growth conditions [Citation44]. Concerning other nutrients, the CNPK ratio affected the growth of Chlorella vulgaris, however, the net effect slightly returns to the osmosis of the growth medium. It was observed that BG-II supports algal culture by CNPK nutrients on a ratio of 1.0C:35.3N:1.0P:1.29 K and wide differences between all treatments and the original media [Citation45].
3.3. Biochemical composition
In general, the different parts of the cultivation system showed no direct effect on the algal biochemical composition. It could be mentioned that such a structure allows proper growth and increases the net gain of biomass. The biochemical profile () was found to agree with previous results of proteins, carbohydrates, and oils of Chlorella vulgaris [Citation46].
Table 5. Biochemical constituents (%) of Chlorella vulgaris grown in Net House Photobioreactor.
The carbon-rich waste used in the current work is produced from alcohol production, specifically from spent-wash or distillery effluent. The raw spent-wash generated is acidic in nature, having dark brown colour with an unpleasant odour, high COD, and BOD (1,00,000 and 45,000 mg.l−1, respectively). It is commonly used for fertigation, cattle feed supplement, soil ameliorant, and vermicomposting production. It was found to be rich in organic carbon which accounted for more than 90 g.l−1 [Citation47].
3.3.1. Protein and amino acids of Chlorella biomass
On average, crude protein reached 51% of dry weight and was composed of 9.8% soluble protein and 41.2% true protein (). Increasing soluble protein content might be due to the late time of harvesting or cell decomposition. Soluble proteins refer to the increase of nitrogenous compounds including ammonia, nitrate, nitrite, free amino acids, and short chain peptides. Of these compounds, amino acids seem to be more beneficial in plant nutrition, poultry, ruminants, and human nutrition. Poultry receives nutrients through the consumption of natural feedstuffs, but some key essential amino acids (lysine, methionine, threonine, and tryptophan), vitamins, and minerals are often offered as synthetic supplements [Citation48]. Amino acids play a vital physiological role in the animal and human body which are assembled and metabolized to form proteins that are used to build different body tissues [Citation49]. Among the determined amino acids . glutamic acid was the dominant (7.08 mg.g−1), while the minimum was tryptophan and tyrosine (0.39 mg.g−1 for each). Glutamate is the precursor of different organelles in the living cells, where arginine is metabolized to ornithine, citrulline, and arginosuccinate [Citation50] with a high N:C ratio (4:6), and along with asparagine (2:4) acts as a major nitrogen storage compound in higher plants [Citation51]. Also, as a precursor of proline which is a cyclic amino acid accumulated in response to different abiotic stresses including drought, salinity, low and high temperatures, and heavy metals [Citation52]. Digestibility of amino acids is also affected by health and by alteration of the nutrient composition of diet [Citation53]. Diets having 90% arginine compared to lysine and high methionine were found to be beneficial in minimizing oxidative stress, modulating metabolic parameters, and influencing indicators of intestinal barrier integrity in turkeys with necrotic enteritis [Citation54].
Table 6. Amino acids from biomass of Chlorella vulgaris collected from NHPBR.
3.3.2. Carbohydrates
Carbohydrates represented 13.4% of dry weight and included 71.6% non-reducing sugars and 28.4% reduced sugars (). Chlorella has been reported to have relatively high carbohydrate and lipid contents [Citation55]. In addition, different species of Chlorella are common microalgae used to treat wastewater because of their high tolerance of soluble organic compounds and ease of metabolites alteration by application of physical stress such as radiation [Citation56]. The effect of organic carbon on carbohydrate content depends on the carbon source, its concentration, and the grown algal species. For instance, the addition of 10.0 g.l−1 glucose increases the lipid content of Chlorella vulgaris ESP-31 [Citation57], while the lipid content in Chlorella sp. was not affected by 2.0 g.l−1 glucose [Citation58]. This suggests that utilization of monosaccharides leads to fast microalgal growth. However, with the higher concentration of monosaccharides transported from the medium into algal cells, the faster synthesis of cellular carbohydrates occurred, thereby reducing the cellular protein content [Citation59].
3.3.3. Lipids and fatty acids
Unlike other oil crops, microalgae grow rapidly and among them, Chlorella species are exceedingly rich in oil. Microalgae with high oil productivities are desired for producing essential oils, unsaturated fatty acids, and biodiesel [Citation60,Citation61]. The oil content of Chlorella vulgaris was 12.1% (), which was reported to increase under nutrient stress [Citation62,Citation63]. In addition, the application of organic carbon was reported to enhance lipid content. For instance, the lipid content of Chlorella sorokiniana grown mixotrophically at 5 g.l−1 glucose was higher than that of autotrophic cells [Citation64].
In addition to the impact on oil content, cultivation conditions significantly influence the fatty acid profile. In the present study, the composition of Fatty Acid Methyl Esters (FAMEs) of Chlorella vulgaris is shown in . The most potent fatty acids were identified as C16:0 (palmitic acid methyl ester, 12.16%); C18.0 (Stearic acid methyl ester, 3.64%); C18.1 (oleic acid methyl ester, 32.81%); C18:2 (linoleic acid methyl ester, 12.14); C20:4 (arachidonic acid methyl ester, 4.12) and C22:6 (docosahexaenoic acid methyl ester, 4.09).
Table 7. Fatty acids methyl ester of Chlorella vulgaris grown in net house photobioreactor NHPBR.
The content of the saturated fatty acids (19.75%) in Chlorella oil was lower than the unsaturated fatty acids (68.55%) and the ratio between saturated and unsaturated fatty acids was approximately 0.2:1. Nutritionally, essential fatty acids, such as omega-3 fatty acids, serve as important cellular functions. They are a necessary part of the diet because the human body has no biochemical pathway to produce these molecules [Citation65]. In addition to nutritional value, the recent focus on algal oil took wide lines for renewable energy production including biodiesel [Citation66,Citation67]; bioalchohol [Citation68,Citation69]; and biogas [Citation70]. In this concern, long chain and saturated fatty acids are beneficial, in which a biorefinery approach can be applied to use fatty acids for both energy and nutrition [Citation71].
3.3.4. Ash content
Ash content of the grown microalga was markedly affected and raised to be about 13.0% on a dry weight basis (). In general, algal ash content monitored the mineral nutritional status, especially with a mass production scale. Such an increase in ash content could be attributed to the high absorption potential. Among the macronutrients (); phosphorous calcium and potassium were found to be the abundant elements and reached (%) 2.8, 2.39 and 1.95; respectively. As for the micronutrients, iron is the most one. The data were in contrast with those previously obtained, where 9.18% of ash was recorded with outdoor grown Chlorella vulgaris using commercial fertilizer compounds [Citation39].
Table 8. Ash fraction of Chlorella vulgaris grown in net house photobioreactor NHPBR.
In addition, ash content also varied from one species to another based on genetic behaviour, where 30.43% was obtained in Amphora coffeaeformis [Citation72]. Furthermore, the growth stage of the sole alga is responsible for ash content since Spirulina platensis represented 10.4 and 12.08% for vegetated and stressed grown cells [Citation73].
4. Conclusions
Vertical expansion of algal production allows high production potential and reduces the overall costs in terms of land, labour, electricity, and infrastructure. The current growth photobioreactor showed interesting results regarding microalgal biomass production with desired biochemical composition. Further studies are recommended to evaluate microalgal CO2 sequestration potential using the NHPBR.
Acknowledgments
This research work has been carried out as a part of activities of the Algal Biotechnology Unit, National Research Centre (Prof. Dr. Abo El-Khair B. El-Sayed). The authors wish to express grateful thanks to staff members of the Algal Biotechnology Unit.
Disclosure statement
No potential conflict of interest was reported by the author(s).
References
- Almutairi AW. Full utilization of marine microalgal hydrothermal liquefaction liquid products through a closed-loop route: towards enhanced bio-oil production and zero-waste approach. 3 Biotech. 2022;12(9):209.
- Bilanovic D, Andargatchew A, Kroeger T, et al. Freshwater and marine microalgae sequestering of CO2 at different C and N concentrations – response surface methodology analysis. Energy Convers Manage. 2009;50(2):262–267.
- Hirata K, Phunchindawan M, Tukamoto J, et al. Cryopreservation of microalgae using encapsulation-dehydration. Cryo Lett. 1996;17:321–328.
- Jacob-Lopes E, Cacia Ferreira Lacerda LM, Franco TT. Biomass production and carbon dioxide fixation by Aphanothece microscopica Nägeli in a bubble column photobioreactor. Biochem Eng J. 2008;40(1):27–34.
- de Morais MG, Costa JAV. Carbon dioxide fixation by Chlorella kessleri, C. vulgaris, Scenedesmus obliquus and Spirulina sp. cultivated in flasks and vertical tubular photobioreactors. Biotechnol Lett. 2007;29(9):1349–1352.
- Mountourakis F, Papazi A, Kotzabasis K. The microalga Chlorella vulgaris as a natural bioenergetic system for effective CO2 mitigation—new perspectives against global warming. Symmetry (Basel). 2021;13(6):997), doi:10.3390/sym13060997.
- Sydney E, Da Silva T, Tokarski A, et al. Screening of microalgae with potential for biodiesel production and nutrient removal from treated domestic sewage. Appl Energy. 2011;88(10):3291–3294.
- Chiu S-Y, Kao C-Y, Chen C-H, et al. Reduction of CO2 by a high-density culture of Chlorella sp. in a semicontinuous photobioreactor. Bioresour Technol. 2008;99(9):3389–3396.
- Krishnan V, Uemura Y, Suzana Y, et al. Aspects of carbon dioxide mitigation by Nannochloropsis oculata cultured in a photobioreactor. Appl Mech Mater. 2014;625:12.
- Chiu S-Y, Kao C-Y, Tsai M-T, et al. Lipid accumulation and CO2 utilization of Nannochloropsis oculata in response to CO2 aeration. Bioresour Technol. 2009;100(2):833–838.
- Han SF, Jin W, Tu R, et al. Optimization of aeration for biodiesel production by scenedesmus obliquus grown in municipal wastewater. Bioprocess Biosyst Eng. 2016;39(7):1073–1079.
- Kamyab H, Md Din MF, Lee CT, et al. Lipid production by microalgae Chlorella pyrenoidosa cultivated in palm oil mill effluent (POME) using hybrid photo bioreactor (HPBR). Desalin Water Treat. 2015;55(13):3737–3749.
- Zhang S, Liu Z. Advances in the biological fixation of carbon dioxide by microalgae. J Chem Technol Biotechnol. 2021;96(6):1475–1495.
- Almutairi AW, Al-Hasawi ZM, Abomohra AE-F. Valorization of lipidic food waste for enhanced biodiesel recovery through two-step conversion: a novel microalgae-integrated approach. Bioresour Technol. 2021;342:125966.
- Silva T, Moniz P, Silva C, et al. The role of heterotrophic microalgae in waste conversion to biofuels and bioproducts. Processes. 2021;9(7):1090.
- Eriksen NT. Production of phycocyanin—a pigment with applications in biology, biotechnology, foods and medicine. Appl Microbiol Biotechnol. 2008;80(1):1–14.
- Chen G-Q, Chen F. Growing phototrophic cells without light. Biotechnol Lett. 2006;28(9):607–616.
- Chisti Y. Biodiesel from microalgae beats bioethanol. Trends Biotechnol. 2008 Mar;26(3):126–131.
- El S, Battah M, Wehedy. Utilization efficiency of artificial carbon dioxide and corn steam liquor by chlorella vulgaris. Biolife Journal. 2015.
- El-Sayed A, El-Sheekh M. Outdoor cultivation of Spirulina platensis for mass production. Notulae Scientia Biologicae. 2018;10:38.
- Stanier RY, Kunisawa R, Mandel M, et al. Purification and properties of unicellular blue-green algae (order chroococcales). Bacteriol Rev. 1971;35(2):171–205.
- Hiscox JD, Israelstam GF. A method for the extraction of chlorophyll from leaf tissue without maceration. Can J Bot. 1979;57(12):1332–1334.
- Ma T, Zuazaga G. Micro-Kjeldahl determination of nitrogen. A new indicator and an improved rapid method. Indus Eng Chem Anal Ed. 1942;14(3):280–282.
- Rosni S, Ahmad F, Awang A, et al. Crude proteins, total soluble proteins, total phenolic contents and SDS-PAGE profile of fifteen varieties of seaweed from Semporna, Sabah, Malaysia. Int Food Res J. 2015;22:1483–1493.
- Cornwell DG. Techniques in protein chemistry (Bailey, J. Leggett). J Chem Educ. 1964;41(6):352.
- DuBois M, Gilles KA, Hamilton JK, et al. Colorimetric method for determination of sugars and related substances. Anal Chem. 1956;28(3):350–356.
- Miller GL. Use of dinitrosalicylic acid reagent for determination of reducing sugar. Anal Chem. 1959;31(3):426–428.
- Liu J, Huang J, Sun Z, et al. Differential lipid and fatty acid profiles of photoautotrophic and heterotrophic chlorella zofingiensis: assessment of algal oils for biodiesel production. Bioresour Technol. 2011;102:106–110.
- Chapman HD, Pratt PF. Methods of analysis for soils, plants and waters. Soil Sci. 1962;93(1.
- Davis R, Aden A, Pienkos PT. Techno-economic analysis of autotrophic microalgae for fuel production. Appl Energy. 2011;88(10):3524–3531.
- El-Fouly MM, Abdalla FE, El-Sayed AB. Modified open plate system for open door production of algal biomass. Egyptian Journal of Phycology. 2001;2(1):1–7.
- Yun J-H, Smith VH, Pate RC. Managing nutrients and system operations for biofuel production from freshwater macroalgae. Algal Res. 2015;11:13–21.
- Egbo M, Okoani A, Okoh I. Photobioreactors for microalgae cultivation–an overview. Int J Sci Eng Res. 2018;9:65–74.
- El-Sayed A. Carotenoids accumulation in the green alga Scenedesmus sp. incubated with industrial citrate waste and different induction stresses. Nat Sci. 2010;8(10):34–40.
- Ogawa T, Aiba S. Bioenergetic analysis of mixotrophic growth in Chlorella vulgaris and Scenedesmus acutus. Biotechnol Bioeng. 1981;23(5):1121–1132.
- Xie J, Zhang Y, Li Y, et al. Mixotrophic cultivation of Platymonas subcordiformis. J Appl Phycol. 2001;13(4):343–347.
- Brennan L, Owende P. Biofuels from microalgae—a review of technologies for production, processing, and extractions of biofuels and co-products. Renew Sust Energ Rev. 2010;14(2):557–577.
- Miao X, Wu Q. Biodiesel production from heterotrophic microalgal oil. Bioresour Technol. 2006;97(6):841–846.
- Cupo A, Landi S, Morra S, et al. Autotrophic vs. heterotrophic cultivation of the marine diatom cyclotella cryptica for EPA production. Mar Drugs. 2021;19(7):355.
- Andrade MR, Costa JA. Mixotrophic cultivation of microalga Spirulina platensis using molasses as organic substrate. Aquaculture. 2007;264(1-4):130–134.
- Chen F. High cell density culture of microalgae in heterotrophic growth. Trends Biotechnol. 1996;14(11):421–426.
- Zhang X-W, Zhang Y-M, Chen F. Application of mathematical models to the determination optimal glucose concentration and light intensity for mixotrophic culture of Spirulina platensis. Process Biochem. 1999;34(5):477–481.
- Bashir KMI, Mansoor S, Kim N-R, et al. Effect of organic carbon sources and environmental factors on cell growth and lipid content of Pavlova lutheri. Ann Microbiol. 2019;69(4):353–368.
- Li L, Huang J, Almutairi AW, et al. Integrated approach for enhanced bio-oil recovery from disposed face masks through co-hydrothermal liquefaction with Spirulina platensis grown in wastewater. Biomass Conversion and Biorefinery. 2021;09:25.
- Figler A, Márton K, B-Béres V, et al. Effects of nutrient content and nitrogen to phosphorous ratio on the growth, nutrient removal and desalination properties of the green alga Coelastrum morus on a laboratory scale. Energies. 2021;14(8):2112.
- El-Sayed A. Physiological studies on some fresh water green algae: PhD Thesis. Faculty of Agriculture, Cairo University; 1999.
- Pugazh G, Thiyagu R, Sivarajan P. Physico-chemical characterization of raw and diluted effluent from distillery industry. 2016;3:2055–2059.
- Ravindran V. Poultry feed availability and nutrition in developing countries. Poultry Dev Rev. 2013;01:60–63.
- Debnath BC, Biswas P, Roy B. The effects of supplemental threonine on performance, carcass characteristics, immune response and gut health of broilers in subtropics during pre-starter and starter period. J Anim Physiol Anim Nutr. 2019;103(1):29–40.
- Slocum RD. Genes, enzymes and regulation of arginine biosynthesis in plants. Plant Physiol Biochem. 2005;43(8):729–745.
- Lea PJ, Sodek L, Parry MA, et al. Asparagine in plants. Ann Appl Biol. 2007;150(1):1–26.
- Sharma SS, Dietz K-J. The significance of amino acids and amino acid-derived molecules in plant responses and adaptation to heavy metal stress. J Exp Bot. 2006;57(4):711–726.
- Chrystal PV, Moss AF, Khoddami A, et al. Effects of reduced crude protein levels, dietary electrolyte balance, and energy density on the performance of broiler chickens offered maize-based diets with evaluations of starch, protein, and amino acid metabolism. Poult Sci. 2020;99(3):1421–1431.
- Ognik K, Konieczka P, Mikulski D, et al. The effect of different dietary ratios of lysine and arginine in diets with high or low methionine levels on oxidative and epigenetic DNA damage, the gene expression of tight junction proteins and selected metabolic parameters in Clostridium perfringens-challenged turkeys. Vet Res. 2020;51(1):1–14.
- Ho S-H, Huang S-W, Chen C-Y, et al. Characterization and optimization of carbohydrate production from an indigenous microalga chlorella vulgaris FSP-E. Bioresour Technol. 2013;135:157–165.
- Metting Jr. FB, Biodiversity and application of microalgae. J Ind Microbiol Biotechnol. 1996;17(5-6):477–489.
- Yeh K-L, Chang J-S. Effects of cultivation conditions and media composition on cell growth and lipid productivity of indigenous microalga Chlorella vulgaris ESP-31. Bioresour Technol. 2012;105:120–127.
- Cheirsilp B, Torpee S. Enhanced growth and lipid production of microalgae under mixotrophic culture condition: effect of light intensity, glucose concentration and fed-batch cultivation. Bioresour Technol. 2012;110:510–516.
- Zhang W, Zhang P, Sun H, et al. Effects of various organic carbon sources on the growth and biochemical composition of chlorella pyrenoidosa. Bioresour Technol. 2014;173:52–58.
- Rizwan M, Lee JH, Gani R. Superstructure optimization of biodiesel production from microalgal biomass. IFAC Proceedings. 2013;46:111–116.
- Almutairi AW. Evaluation of halophilic microalgae isolated from Rabigh Red Sea coastal area for biodiesel production: screening and biochemical studies. Saudi J Biol Sci. 2022;29(8):103339.
- Sheehan J, Dunahay T, Benemann J, et al. Look back at the U.S. Department of Energy's aquatic species program: biodiesel from algae; Close-Out Report.; National Renewable Energy Lab., Golden, CO. (US); 1998. p. Medium: ED; Size: 325 pages.
- Almutairi AW. Effects of nitrogen and phosphorus limitations on fatty acid methyl esters and fuel properties of Dunaliella salina. Env Sci Pollut Res. 2020;27(26):32296–32303.
- Wan M, Liu P, Xia J, et al. The effect of mixotrophy on microalgal growth, lipid content, and expression levels of three pathway genes in Chlorella sorokiniana. Appl Microbiol Biotechnol. 2011;91(3):835–844.
- White B. Dietary fatty acids. Am Fam Physician. 2009;80(4):345–350.
- Gomaa M, Refaat M, Salim T, et al. Identification of green alga Chlorella vulgaris isolated from freshwater and improvement biodiesel productivity via UV irradiation. Microbiol Biotechn Lett. 2019;47:381–389.
- Almutairi AW, El-Sayed AE-KB, Reda MM. Evaluation of high salinity adaptation for lipid bio-accumulation in the green microalga Chlorella vulgaris. Saudi J Biol Sci. 2021;28(7):3981–3988.
- El-Sayed A, Nashwa A, El-Fakharany M. Bioethanol production from Egyptian alga Enteromorpha sp. Middle East Journal of Applied Sciences. 2017;1:216–225.
- Fetyan NA, El-Sayed AE-KB, Ibrahim FM, et al. Bioethanol production from defatted biomass of nannochloropsis oculata microalgae grown under mixotrophic conditions. Environ Sci Pollut Res. 2022;29(2):2588–2597.
- Zabed HM, Akter S, Yun J, et al. Biogas from microalgae: technologies, challenges and opportunities. Renewable Sustainable Energy Rev. 2020;117:109503.
- Abedi E, Sahari MA. Long-chain polyunsaturated fatty acid sources and evaluation of their nutritional and functional properties. Food Sci Nutr. 2014;2(5):443–463.
- El-Sayed AEB, Aboulthana WM, El-Feky AM, et al. Bio and phyto-chemical effect of Amphora coffeaeformis extract against hepatic injury induced by paracetamol in rats. Mol Biol Rep. 2018 Dec;45(6):2007–2023.
- Hassan N, Mohamed Z, El-Sayed A. Production and evaluation of pasta supplemented with Spirulina platensis biomass. Adv Food Sci. 2015;37(4):153–162.