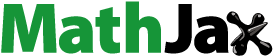
Abstract
The present paper reports on the effects of Aluminum-doping on the micro-structural and dielectric properties of La0.7Sr0.25Na0.05Mn0.95Al0.05O3 ceramics. The analyses of the X-ray diffraction data by the Rietveld refinement technique confirmed the formation of a rhombohedral phase in the Rc space group. The dielectric properties were analyzed by the complex impedance spectroscopic techniques in a wide temperature extent and a spectral range between 2 kHz and 1 MHz. The Nyquist diagram with a single semicircular arc is explained by the contribution of two conducting phases associated with the effects of grains and grain boundaries. The conductivity spectrum obeyed Jonscher’s power law and the conduction mechanism was attributed to the correlated barrier hopping mechanism. The dielectric parameters such as the dielectric constant (ε″) showed a compositional dependence, indicating the prevalence of inter-grain conductive contribution and microstructural features which can be interpreted by the Maxwell–Wagner process.
1. Introduction
The need for powerful nanotechnology devices in nowadays society has motivated researchers to find stable and more flexible structures having elevated performance and multiple applications [Citation1–10]. Indeed, according to the literature, lead-free perovskites could possibly be potential candidates for the storage of energy thanks to their multiferroic features and diverse applications such as dielectric capacitor systems and batteries [Citation11–13]. Additional to the commercially important aspect, these materials have many advantages, including simple manufacture, chemical stability, high resistance to degradation mechanisms, and tunable Curie temperature TC by changing the doping level [Citation14–16].
Recently, manganite materials have become one of the most interesting research topics due to their physical properties and their colossal magneto-resistance (CMR) [Citation17]. Many studies open up a wide range of chemical and microstructural designs in order to manipulate the magnetic and dielectric properties of these compounds [Citation18]. However, the combination of intrinsic and extrinsic factors induces a sophisticated framework to understand the physical properties of manganite crystals [Citation19]. Besides their important magnetic behaviour, some manganites have high dielectric permittivity values, which enhance their multiferroic properties and their industrial applications. These behaviours prove the contribution of microstructural design on the magneto-transport performance analyzed by Zener’s theory [Citation20].
It was believed that the physical properties of manganites are controlled by the structural distortion and the motion of charge carriers between two states of manganese, namely Mn3+ and Mn4+ ions. However, several theories argued that the strong spin-phonon coupling related directly to the dynamic Jahn–Teller (JT) type distortion of the MnO6 octahedra should be considered to explain the feature of the materials [Citation21, Citation22]. In fact, the structural distortion including octahedral tilting and/or cation displacement induces a spontaneous polarization that depends on the oxidation state and many parameters such as grain size and dopant nature [Citation23]. Along with the presence of many theoretical and experimental studies, the framework of Zener double exchange interaction model is successfully used to explain the magneto-transport behaviour in manganites. This theory is explained by the mobility of charge carriers with a simultaneous transfer of eg electrons between two mixed valences of Mn ions via Oxygen (2p orbital). Earlier studies have shown that Zener double exchange interaction analyses and charge carriers’ concentration can be influenced by different extrinsic factors such as microstructure design (grains and grain boundaries), defect related to oxygen distribution, and valence fluctuation of ions [Citation24]. However, several works have reported that a partial substitution at A site or/and Mn site are conventional techniques for improving the physicochemical properties and the magneto-transport features of manganites. In fact, doping elements induce a variation of the chemical pressure and the structural parameters (bond angle and distance) which alters the double exchange interaction strength and the intrinsic physical properties of the sample [Citation25]. The properties of manganite can be tuned also by an appropriate substitution with a monovalent ion such as (Li, Na, K) which causes the oxidation of Mn3+ to Mn4+ ion to give rise to the double exchange interactions Mn3+–O2−–Mn4+. These interactions are the origin of the coexistence of large magnetoresistance and magnetocaloric effect as reported by many research groups.
It should be noted that current analyses of the conductivity indicate that the hopping of charge carrier can be explained by the correlated barrier hopping (CBH) mechanism and confirm the presence of two electrical relaxation related to the grain and the grain boundaries. This heterogeneous micro-structure was analyzed also with impedance spectroscopy, which proves the important effect of the grain boundaries and indicates the non-Debye relaxation. Moreover, the high value of explained by the interfacial polarization effect and Koop’s theory points multiferroic proprieties of the sample.
The perovskite manganese oxide La0.7Sr0.25Na0.05Mn0.95Al0.05O3(LSNMAl0.05) ceramic has interesting magnetic properties as reported by Zaidi et al. [Citation26] confirming, that it can be used in modern nanotechnologies such as magnetic refrigeration and magnetic recording process. In fact, the incorporation of Aluminum cation at B site creates a disorder in the microstructural properties, which affects the conductivity and dielectric parameters of La0.7Sr0.25Na0.05MnO3 compounds. This feature is not clearly understood. Furthermore, there are few papers and experimental results in the literature concerning this issue. These reasons motivated us to investigate the dielectric properties of the above-mentioned compound and search for a particular one with a multiferroic structure.
In fact, our main objective is to gain insight on the effect of temperature and spectral behaviour of dielectric properties and conduction mechanism using the impedance spectroscopy analyses which can provide a detailed information about the performance of the sample and its nanotechnologies application.
2. Experimental
The perovskite manganese oxide LSNMAl0.05 compounds were elaborated by the polymerization complex (PC) sol–gel route in air atmosphere without any inert gases using metal nitrates as starting materials. In this method La(NO3)3.6H2O, Sr(NO3)2.6H2O, NaNO3, Mn(NO3)2.4H2O and Al(NO3)3.9H2O raw material (with purity of 99.9%) were weighed in the desired proportions and dissolved with small amounts of water. However, an aqueous solution of appropriate stoichiometric quantities of highly purified metal nitrates was transformed into citrates by adjusting pH between 6.5 and 7. Under slow evaporation and adding ethylene glycol as polymerizing agent, the obtained sol was heated between 140 and 190 °C until obtained a black-brown wet gel. Subsequently, further progressively heating yields a dry fluffy porous mass, which was calcined at 600°C for 12 h. Finally, the desired mixture was pressed into pellets form (under 8 T/cm2) and sintered at 900°C for 15 h to attain a good crystal structure. The chemical composition and structural analyses of Al incorporation into the structure LSNMAl0.05 are reported also by Zaidi et al. [Citation26]. The detailed preparation procedure including mixing stoichiometric amounts of metal nitrate and heating procedure was presented [Citation26]. The sol–gel process is illustrated in the flow chart of Figure .
Microstructural behaviour such as morphological characters and grain size distribution of the final product were studied using scanning electron microscopy (SEM) at room temperature performed by JSM-6400 field emission working at acceleration voltage of 25 kV. Sample purity, crystalline phases, and crystallite size of LSNMAl0.05 compound were examined using powder X-ray diffraction (XRD) by employing the analytical XPERT-PRO diffract-meter with Cukα radiation (λCukα = 1.54 Å). The recorded data in the 2θ range of 10°–100° with step sizes of 0.017° and counting time of 18 s per step were fitted using Rietveld refinement and FULLPROF software.
Dielectric and conduction data recorded at different frequencies and temperatures were performed by the complex impedance analysis system [Citation27]. Before applying this technique, a thermal vapour deposition and circular mask were used to grow a thin aluminum film (a diameter of 6 mm and a thickness of 200 nm) on two sides of the pellets. After this step, the dual capacitance/conductance results were collected employing an impedance analyzer (Agilent 4294A) and a cold plate of a liquid nitrogen cooled cryostat.
3. Results and discussion
3.1. Structural and morphological properties
The XRD patterns and Rietveld simulated results of LSNMAl0.05 nano-powders are displayed in Figure (a). These patterns showed a sharp peak with high intensity, indicating a single-phase formation of a crystalline phase and high crystalline nature. In fact, the compound was crystallized with a rhombohedral structure (space group R-3c) characterized by the lattice parameters a = b = 5.486Å and c = 13.325Å producing a cell volume of 347.44 (Å3). Moreover, the scanning electron micrograph (SEM) allows to check the grain shapes and the grain boundary connection and its uniformity.
Figure 2. (a) Experimental and fitted data correspond to the open circle and solid lines, respectively, of LSNMAl0.05 XRD patterns. Vertical bars present the position of the Bragg reflections. The bottom of the figure shows the differences between the observed and the calculated intensities. (b) The Williamson-Hall curve of LSNMAl0.05. The insert exhibits a typical SEM image.

The inset of Figure (a) depicts the surface morphology of LSNMAl0.05 compound using the scanning electron micrograph (SEM) which allows for checking the grain shapes and the grain boundary connection and its uniformity. Obviously, well-defined grains with visible grain boundaries are observed which is in agreement with the impedance analysis. In fact, this SEM micrograph shows a highly dense surface and homogeneous microstructure attributed to the presence of a uniform distribution of regular-shaped grains with different size ranges. Moreover, it is evident that the grains are separated by clear grain boundaries with few scattered pores proving the existence of certain porosity. Usually, ceramics compounds are characterized by the contributions of semi-conductive grains and highly resistant grain boundaries in the conduction mechanism. The presence of thin insulating blocking layers at the internal grain boundaries explained by the Schottky barrier plays a crucial role in the dielectric behaviour of the compound and can be affected by the grain size and the barrier width [Citation28].
More investigation about the micro-structural behaviour can be analyzed using the impact of the lattice strain on the crystallite size which is estimated by the broadening of the XRD peak and Williamson-Hall (W-H) method [Citation29]. This quantity is defined as the sum of the crystallite size broadening and the strain broadening
which depends on Bragg diffraction angle θhkl variation:
This method is performed to evaluate the impact of crystallite size and strain effect on the broadening using the convolution. However, the two effects are the main contribution of the line breadth. The first part is related to the crystallite size calculated by Debye-Scherrer’s formula as described below:
where
represents the factor of shape usually taken as
and
is the full width of Bragg diffraction peak [hkl] estimated at half maximum intensity. In this paper, just the noticeable peaks are considered.
The second part is related to the effect of the micro strains which is known as broadening of Bragg reflections. Its angular dependency is given by Wilson formula:
where
represents the root mean square value of the micro-strain in the material.
From a linear fit of the curve of versus
, the grain size and the lattice-strain value can be estimated using the y-intercept and the slope, respectively as illustrated in Figure (b).
All calculated results of the average crystal size using W-H model and Debye-Scherrer’s method (see Table ) imply that lattice strain effect should be considered in the grain size estimation of LSNMAl0.05.
Table.1. X-ray diffraction average crystal size of LSNMAl0.05.
In order to gain further insights, the inset of Figure (b) presents the SEM micrographs of LSNMAl0.05. Flat surfaces used to calculate the grain size (DSEM) by linear intercept technique. In fact, a simple comparison between the grain size values obtained by SEM and XRD (see Table ) proves that each grain observed by micrographs contains several crystallite forms.
3.2. Electrical properties
Figure exhibits the spectra of the electrical conductivity for several values of temperature ranging from 200 K to 400 K with a step of 20 K. Obviously, the temperature has a significant effect on the behaviour of dc-conductivity, which can be elucidated by the hopping model. This result can be explained by the semiconducting nature of the sample which is reinforced by the effect of the incorporation of Aluminum. In fact, the correlation between the conductivity and Al content can be explained by the rise of the polaron binding energies [Citation30]. Usually, the electronic activity in manganite is governed and controlled by the movements of charge carriers between Mn cation and (Mn3+/Mn4+) ratio. The increase of the Al-doping at Mn site produces a structural distortion that creates a disorder in charge carriers’ transfer through a partial substitution of some Mn-O-Mn by Mn-O–Al bonds. This mechanism will reduce the densities of 3d-electrons accountable for hopping conduction mechanism.
Figure 3. Frequency-dependent ac conductivity for LSNMAl0.05 for different values of the temperature
. The inset:
versus
.

At the mesoscopic scale and concerning the dielectric behaviour, the investigated sample may be regarded as heterogeneous. The surface morphology reveals semiconductor grains that are separated by insulating layers. This affects the conductivity and the permittivity of the sample. The disorder provoked by the temperature increase also affects the electrical properties [Citation31, Citation32].
To understand the conductivity process of our compound, we use two different hopping models to fit the experimental results of conductivity [Citation33]. These models are Mott variable-range hopping (VRH) model and the small polaron hopping (SPH) model [Citation34]. However, a systematic investigation provides that the VRH model can be the appropriate process to explain the conduction mechanism at low temperature range. In fact, Mott’s 3D-VRH model proves that the transport mechanism is characterized by binding energies and negligible hopping given as where T0 is the Mott temperature and
is a constant. However, the small polaron hopping (SPH) process is more appropriate to clarify the transport mechanism of charge carriers at high temperature region [Citation35]. Mathematically, the thermally activated SPH model is given by the expression
where Ea is the activation energy and kB is Boltzmann constant. The calculated activation energy is Ea = 0.14 eV (inset of Figure ). It suggests the role of the polaron formation and energy in the sample of LSNMAl0.05. This value is considered in the same order as other novel oxides including the manganites, which can be explained by the localization and delocalization of charge carriers [Citation36].
Figure presents versus the frequency for a particular temperature. The dc-conductivity at low frequencies remained constant and showed a behaviour independent of the frequency which is explained by the weakness of the applied electric-field to perturb the hopping conduction process. However, this intrinsic feature can be attributed to the jump relaxation process associated with the long-range translational motion of charge transport [Citation37]. On the other hand, a dispersive region appears when increasing frequency. This proves that ac-conductivity governs the conduction mechanism associated with hopping frequency. In this regime, an exponential rise of dynamical conductivity would be the extension to the carrier motions which admit forward/backward hopping and short-range transitional motion due to the jump carrier conduction [Citation38]. It is noticed that the increase of Al-content in the polycrystalline structure induces a modification in charge carrier density and reflects the contribution of several models of hopping. In general, the conductivity curve can be analyzed and fitted using Johnscher’s formula [Citation39]:
where the coefficient A is a constant and n (0 < n < 1) is an exponent associated to the interaction degree of charge carriers and all intrinsic properties of materials such crystal lattice, temperature and frequency. The exponent n can be a solution to define the appropriate model and it describes the impact of polarizability related to the hopping energy barriers in the material as reported by Peláiz-Barranco et al. [Citation40]. Obviously, Figure illustrates the variation of conductivity with frequency and shows a quite linear dependence which provides unique conduction properties in LSNMAl0.05. This behaviour is attributed to the short-range hopping model, whereas the long-range hopping is suppressed [Citation41]. The exponent n is evaluated for all the investigated temperatures from a linear fit of conductivity data at high frequencies. The inset of Figure shows the evolution of the exponent n versus the temperature. It is clear that the parameter n decreases with rising temperature which proves that the conductivity mechanism in this solid is principally due to the hopping of carrier charge over a potential barrier process explained by the correlated barrier hopping (CBH) mechanism [Citation32, Citation42–44]. This model confirms that the conduction is manifested via bipolaron or single-polaron hopping mechanism through a Coulomb’s potential barrier. However, the minimum energy
of the charge carriers to move from one site to another is estimated from the slope of the Mott’s law equation described below as:
Figure 4. Variation of ac-conductivity versus frequency for a particular temperature (T = 280 K). A linear fit is used to obtain the exponent n characterizing the power law. The inset: the variation of the exponent n versus the temperature.

where, presents the Boltzmann constant. Using the linear fit of the (
) data as a function of temperature, the value of
may be assessed. We obtain
. It is clear that temperature reduces the effect of
which explains the increase of the free carriers’ density and the ac-conductivity with temperature.
The impedance spectroscopy is an important tool to investigate the dielectric relaxation and the impact of the microstructural properties on the electric response under an applied electric field. It can be used to distinguish the contribution of grain and grain boundaries effect of the compounds using the reactive/resistive parts of impedance from the current signals [Citation45].
Since the sample has simultaneously dielectric and conduction behaviours, the electrical impedance response is represented by a complex number. Its real part is designed by whereas its imaginary part is designed by
:
Figure represents the Nyquist (
∼-
) curve in the complex plane. The fitted data are simulated as a single semi-circle associated to several electrical processes related to the microstructure properties of the sample as well as the effects of the sample-electrode interface. The interface effects can be ignored in the present study. Indeed, usually, electrodes are readily recognizable in most oxide ion conductors with high capacitances, typical of double layer effects. Normally, nanomaterials are electrically heterogeneous consists of two main resistances attributed to the grain and grain boundary resistance, which together dominated the total resistance [Citation46,Citation47]. Each entire curve exhibited a depression behaviour which forbids the centralization of the semicircle on the x-axis.
Figure 5. Nyquist plots of LSNMAl0.05 at several temperatures. The insert exhibits the electrical equivalent circuit.

Such analytic visualization confirms a non-Debye relaxation of the polarization phenomena characterized by the distribution of relaxation times [Citation32]. In fact, many factors such as grain boundaries, atomic defect distribution, and grain orientation induce a non-ideal relaxation [Citation48].
The analysis of impedance response provides a usually powerful method to understand the relaxation process related to the correlation between the charge transport and the microstructural properties (grain and grain boundaries). Usually, the electrical conduction in ceramics is governed by the resistance/capacitance parameters of the grain boundaries [Citation32]. It is expected that the response of the grain is suppressed at high temperature. The intercept of the semi-circle arc on the Z’ axis defines the resistance of the grain (Rg) and grain boundary (Rgb) at low and high frequency ranges, respectively.
An electrical equivalent circuit and Z-view software were applied to fit the experimental measurements and to extract the microstructural parameters (grain/grain boundaries). Normally, nanomaterials are electrically heterogeneous. Therefore, the electrical equivalent circuit consists of two main resistances attributed to the grain and grain boundary resistance, which together dominated the total resistance. In fact, the best fits are obtained when we use an equivalent circuit that consists of a series combination of grains and grains boundary elements.
The inset of Figure presents the equivalent circuit used in the Nyquist fitting, which is assembled by a series of two parallel RC branches [RgCg + RgbCgb]. It denotes the electrical contributions of semi-conducting grains RgCg and the insulating grain boundary RgbCgb at high and low frequencies, respectively.
All the results related to the different parameters evaluated at several temperatures are depicted in Table . It is evident that Rgb values are more significantly important than that of Rg. This reveals the high insulation character of the grain boundaries. From this table, we can notice that the resistance value decreases with increasing temperature. This trend is interpreted by the decrease of the bandwidth magnitude and the double-exchange interaction which is in good agreement with several works on perovskite materials [Citation49]. In fact, the high resistance of the compound can be related to the small grain size and the important amount of the grain boundary as well as the low porosity of the sample as observed by SEM.
Table 2. Fitting parameters of LSNMAl0.05 sample using Zview software.
Figure presents the frequency dependence of the imaginary part of the dielectric constant at different temperatures. At low frequency,
curve revealed an important dispersion which decays when the frequency increases until reaching a very small value. This behaviour is similar to that observed in many ceramic systems and confirms the usual dispersion behaviour [Citation50]. Furthermore, the predominant grain boundary resistance Rgb, the dispersion feature of the dielectric constant at low frequencies as well as the high value of
are related to the interfacial polarization effect and Koop’s theory [Citation51]. According to this mechanism known also as the Maxwell–Wagner (MW) effect, the colossal dielectric properties in perovskites are due to the interfacial polarization mechanism created by the difference of conductivity between semiconducting grains and insulating grain boundaries.
Through hopping, the micro-structural inhomogeneity increased the density of charge carriers at the grain boundaries and generated the polarization. This curve reveals also a decrease of dielectric permittivity with increasing frequency, which opposes the charge carriers’ direction to reach the grain boundaries and consequently the polarization declines [Citation52]. The thermal activation process is an important parameter which allows to the change of the electrical transport of the semiconducting ceramics by increasing temperature. This latter improves the density of entrapped charge carriers at the internal phase boundaries which induces an increase of the polarization and dielectric constant .
It is clear that our sample showed a noticeably large dielectric constant compared to that reported in several studies on pristine LaMnO3 material which can be interpreted by the interfacial polarization mechanism arising from electrically heterogeneous grain and grain boundary regions [Citation53,Citation54]. In fact, different reports in the literature show that the substitution of Mn by a transition metal or La-site by divalent cation induces a structural disorder that can be responsible for a high dielectric constant and increase the dielectric properties of LaMnO3 [Citation55]. Usually, the substitution can increase the impact of grain-boundary regions which form an insulating shell on semiconducting grains, and decrease the mobility of the charge carrier’s polaron/electron.
The above-discussed results concerning the dielectric properties reveal that the sample has a high value of the imaginary part of the dielectric constant. This makes it a potential candidate for microelectronic devices, energy industry applications such as thin-film devices (MEMS/Gb-DRAM), multilayer ceramic capacitors, and high-density energy storage.
4. Conclusion
The La0.7Sr0.25Na0.05Mn0.95Al0.05O3 manganite was synthesized using sol–gel technique. The structural properties showed that our compound has a single phase without any impurities in rhombohedral distorted structure characterized by the space group R-3c. Further, the Williamson-Hall method was used to estimate the size of crystallite. It confirmed the impact of the strain effect on the structure. The variation of the electrical conductivity versus the frequency has been measured for several values of the temperature ranging from 220 K to 400 K. Several models have been used to explain the behaviour of the dc-conductivity and the ac-conductivity. VRH and SPH model are the appropriate mechanism used to explain the dc-conductivity behaviour in low and high temperatures respectively. Meanwhile, the ac-conductivity analyses using Jonscher’s universal power law suggests that the conduction process follows the correlated barrier hopping (CBH) model. The complex impedance has been investigated. The heterogeneous micro-structure was analyzed also by the Nyquist curve, which indicates the non-Debye nature of the relaxation phenomena associated to grains and grain boundaries effect. In fact, the relaxation mechanism is activated by the polaron hopping. The Nyquist diagram has been advantageously exploited to extract the resistance and the capacity that characterizes the equivalent circuit. The contribution of the grains and grain boundaries have been revealed. Moreover, the impedance results proved the presence of two relaxations associated to grains and grain boundaries in the conductivity process. The relaxation mechanism is activated by the polaron hopping and the activation energy obtained by the small polaron hopping model is 0.14 eV. The variation of the dielectric permittivity has been analyzed versus the frequency and the temperature. The dependence of dielectric dispersion frequency could be explained by the mechanism of electron–hole hoping as well as Koop’s theory which is responsible for polarization. Our investigation into the dielectric properties showed a high value of the imaginary part of the dielectric constant, which proves that our sample can be used for energy industry applications such as thin-film devices (MEMS/Gb-DRAM), multilayer ceramic capacitors and high-density energy storage.
Acknowledgement
The authors would like to thank Deanship of Scientific Research at Majmaah University for supporting this work under Project Number No. R-2021-306.
Disclosure statement
No potential conflict of interest was reported by the author(s).
Additional information
Funding
References
- Momin A, Zubair M, Islam MF, et al. Enhance magnetoelectric coupling in xLi0.1Ni0.2Mn0.6Fe2.1O4–(1−x) BiFeO3 multiferroic composites. J Mater Sci: Mater Electron. 2019;30(4):13033–13046.
- Liu Q, Xiao Z, Xie H, et al. Multifunctional layer-perovskite oxide La2-xCexCuO4 for solid oxide fuel cell applications. Int J Hydrogen Energy. 2021;46(15):9818–9825.
- Djellabi R, Ordonez MF, Conte F, et al. A review of advances in multifunctional XTiO3 perovskite-type oxides as piezo-photocatalysts for environmental remediation and energy production. J Hazard Mater. 2022;421:126792.
- Purohit V, Choudhary R, Sahu M. Structural and electrical properties of lead-free perovskite: Bi (Sr 0.25 Ti 0.25 Fe 0.5) O 3. J Inorg Organomet Polym Mater. 2020;30:3026–3035.
- Al-Douri A T, Ibraheam A, Gdoura R, et al. Morphological and optical investigations of the NiZnFe2O3 quaternary alloy nanostructures for potential application in optoelectronics. J Taibah Univ Sci. 2021;15:275–281.
- Heiba Z K, Mohamed M B, Altowairqi Y, et al. Effect of alloying on the structure and electric properties of ZnFe2O4/ZnMn2O4 heterostructure nanocomposites. J Taibah Univ Sci. 2021;15:999–1012.
- Ghosh J, Sengupta A, Halder P, et al. Single polaron hopping in Fe doped glassy semiconductors: structure–electrical transport relationship. J Appl Phys. 2022;132(20):205108.
- Halder P, Bhattacharya S. Debye to non-debye type relaxation in MoO3 doped glassy semiconductors: A portrait on microstructure and electrical transport properties. Phys B. 2023;648:414374.
- Sengupta A, Chamuah A, Ram R, et al. Formation of Li10Zn4O9, Li2MoO3, and ZnSeO3 nanophases: roles in electrical conductivity and electrochemical stability in lithium ion conductors and their crystalline counterparts. ECS J Solid State Sci Technol. 2022;11(11):113008.
- Benali A, Benali E M, Melo BM, et al. Significant improvement of dielectric, magnetic, and gas-sensing properties of the (La0. 8Ca0. 2) 0.6 Bi0. 4FeO3 nanomaterial: particles size effects. J Mater Sci: Mater Electron. 2023;34(1):45.
- Rayssi C, Jebli M, Dhahri J, et al. Experimental-structural study, Raman spectroscopy, UV-visible, and impedance characterizations of Ba0. 97La0. 02Ti0. 9Nb0. 08O3 polycrystalline sample. J Mol Struct. 2022;1249:131539.
- Aman S, Ahmad N, Farid H MT, et al. The electrical, dielectric and magnetic effect of strontium-substituted spinel ferrites for high-frequency applications. J Taibah Univ Sci. 2021;15:721–729.
- Jebli M, Hamdaoui N, Smiri B, et al. Raman spectra, photoluminescence, and low-frequency dielectric properties of Ba 0.97 La 0.02 Ti 1− x Nb 4x/5 O 3 (x = 0.00, 0.05) ceramics at room temperature. J Mater Sci: Mater Electron. 2020;31:15296–15307.
- Li Y, Li J, Chen Q, et al. Enhanced temperature coefficient of resistance and magnetoresistance of Co-doped La0. 67Ca0. 33MnO3 polycrystalline ceramics. Ceram Int. 2022;48:407–414.
- Rayssi C, Rhouma F, Dhahri J, et al. Structural, electric and dielectric properties of Ca 0.85 Er 0.1 Ti 1− x Co 4x/3 O 3 (0≤x≤ 0.1). Appl Phys A. 2017;123:778.
- Zaidi M, Dhahri J, Zeydi I, et al. Large magnetocaloric effect and critical behavior in La 0.7 Ba 0.2 Ca 0.1 Mn 1− x Al x O 3. RSC Adv. 2017;7:43590–9.
- Sudakshina B, Supin K, Vasundhara M. Effects of Nd-deficiency in Nd0. 67Ba0. 33MnO3 manganites on structural, magnetic and electrical transport properties. J Magn Magn Mater. 2022;542:168595.
- Apandi N, Ibrahim N, Awang Z, et al. Effect of Fe substituted on the monovalent La0. 85Ag0. 15Mn1-xFexO3 doped manganites: their electromagnetic and microwave properties. Mater Sci Eng: B. 2022;276:115562.
- Moualhi Y, Rahmouni H, Khirouni K. Dynamics of charge carriers in doped manganite based on conductivity measurements and theoretical models. Phys B. 2021;616:413129.
- Zener C. Interaction between the d-shells in the transition metals. II. Ferromagnetic compounds of manganese with perovskite structure. Phys Rev. 1951;82:403.
- Millis A. Cooperative Jahn-Teller effect and electron-phonon coupling in La 1− x A x MnO3. Phys Rev B. 1996;53:8434.
- Kossi S E, Mnefgui S, Dhahri J, et al. Critical behavior and its correlation with magnetocaloric effect in La0. 7Sr0. 25Na0. 05Mn (1− x) TixO3 (0≤x≤ 0.1) manganite oxide. Ceram Int. 2015;41:8331–8340.
- Ouni I, Ben Khlifa H, M’nassri R, et al. Transport properties and dielectric response of Pr0. 8Na0. 2-xKxMnO3 (x = 0, 0.05, 0.1, 0.15 and 0.2) ceramics synthesized by sol–gel method. Appl Phys A. 2021;127:1–12.
- Hamdi R, Khelifi J, Walha I, et al. Structural and dielectric properties of La 0.5 Pr 0.2 Ba 0.3 Mn 1− x Ti x O 3 (x = 0.0 and 0.1) manganite. J Low Temp Phys. 2021;203:158–179.
- Vadgama V, Gadani K, Udeshi B, et al. Electronic phase derived impedance spectroscopic behavior of La0. 5Nd0. 2A0. 3MnO3 manganites. J Alloys Compd. 2021;885:160930.
- Zaidi N, Elabassi M, Selmi M, et al. Structural characterization and magnetic interactions of La 0.7 Sr 0.25 Na 0.05 Mn 1-x Al x O 3. J Supercond Novel Magn. 2020;33:2257–2267.
- Kossi S E, Rhouma F, Dhahri J, et al. Structural and electric properties of La0. 7Sr0. 25Na0. 05Mn0. 9Ti0. 1O3 ceramics. Phys B. 2014;440:118–123.
- Zang G, Zhang J, Zheng P, et al. Grain boundary effect on the dielectric properties of CaCu3Ti4O12 ceramics. J Phys D: Appl Phys. 2005;38(11):1824.
- Islam S AU, Ikram M. Structural stability improvement, Williamson Hall analysis and band-gap tailoring through A-site Sr doping in rare earth based double perovskite La 2 NiMnO 6. Rare Met. 2019;38:805–813.
- Molak A, Szeremeta A, Zubko M, et al. Influence of hydrostatic pressure on electrical relaxation in non-homogeneous bismuth manganite-lead titanate ceramics. J Alloys Compd. 2021;854:157219.
- Bourguiba M, Raddaoui Z, Smiri B, et al. Effect of Ti substitution on the structural, optical spectroscopy, dielectric and optical conductivity properties of La0. 67Ba0. 25Ca0. 08Mn1-xTixO3 (x = 0 or 0.05) manganite ceramic. J Inorg Organomet Polym Mater. 2022;32(10):3889–3901.
- Raddaoui Z, El Kossi S, Brahem R, et al. Hopping conduction mechanism and impedance spectroscopy analyses of La0.70Sr0.25Na0.05Mn0.70Ti0.30O3 ceramic. J Mater Sci: Mater Electron. 2021;32(12):16113–16125.
- Han J, Yu X, Jin S, et al. Nd1-xSrxMnO3 (x = 0.3): A perovskite manganite ceramic that exhibits PTC and NTC double properties. Ceram Int. 2023;49(3):4386–4392.
- Kossi SE, Rayssi C, Dhahri AH, et al. High dielectric constant and relaxor behavior in La0. 7Sr0. 25Na0. 05Mn0. 8Ti0. 2O3 manganite. J Alloys Compd. 2018;767: 456–463.
- Altintas S P. Structural and magneto-transport features of cobalt-substituted mixed-valence manganites. J Mater Sci: Mater Electron. 2021;32:7176–7186.
- Franchini C, Reticcioli M, Setvin M, et al. Polarons in materials. Nat Rev Mater. 2021;6:580–586.
- Aydi S, Chérif W, Khammassi F, et al. Microstructural properties, dielectric behaviour, conduction mechanism, impedance, and electrical modulus of La0. 65Ca0. 25Sr0. 1MnO3 manganite. Appl Phys A. 2021;127:1–12.
- Hizi W, Rahmouni H, Gassoumi M, et al. Transport properties of La 0.9 Sr 0.1 MnO3 manganite. The Eur Phys J Plus. 2020;135:1–13.
- Khan A A, Fayaz M U, Khan M N, et al. Investigation of charge transport mechanism in semiconducting La 0.5 Ca 0.5 Mn 0.5 Fe 0.5 O 3 manganite prepared by sol–gel method. J Mater Sci: Mater Electron. 2018;29:13577–13587.
- Pelaiz-Barranco A, Gutierrez-Amador M, Huanosta A, et al. Phase transitions in ferrimagnetic and ferroelectric ceramics by ac measurements. Appl Phys Lett. 1998;73:2039–2041.
- Kharrat A BJ, Bourouina M, Moutia N, et al. Gd doping effect on impedance spectroscopy properties of sol-gel prepared Pr0. 5-xGdxSr0. 5MnO3 (0≤x≤ 0.3) perovskites. J Alloys Compd. 2018;741:723–733.
- Raddaoui Z, El Kossi S, Brahem R, et al. Hopping conduction mechanism and impedance spectroscopy analyses of La0. 70Sr0. 25Na0. 05Mn0. 70Ti0. 30O3 ceramic. J Mater Sci: Mater Electron. 2021;32(12):16113–16125.
- Bourguiba M, Raddaoui Z, Dimassi W, et al. Evaluation of the microstructure, optical properties and hopping conduction mechanism of rare earth doped Ba 0.85 Ca 0.12 RE 0.03 Ti 0.90 Zr 0.04 Nb 0.042 O 3 ceramics (RE = Ce3+ and Pr3+). RSC Adv. 2022;12(17):10598–10607.
- Raddaoui Z, Brahem R, Bajahzar A, et al. Structural, double Jonscher response and non-Debye-type relaxor behavior of Ba0. 75Sr0. 25Ti0. 9Zn0. 2O3 ceramic. J Mater Sci: Mater Electron. 2021;32(18):23333–23348.
- Moussi R, Bougoffa A, Trabelsi A, et al. Effect of Sr-substitution on structure, dielectric relaxation and conduction phenomenon of BaTiO3 perovskite material. J Mater Sci: Mater Electron. 2021;32:11453–11466.
- Abram EJ, Sinclair DC, West AR. A strategy for analysis and modelling of impedance spectroscopy data of electroceramics: doped lanthanum gallate. J Electroceram. 2003;10(3):165–177.
- Alotaibi M, Almutairi F, West AR. Resistive-switching in yttria-stabilized hafnia ceramics. J Am Ceram Soc. 2022;106(2):822–828.
- Mansour S O, Louati B, Guidara K. AC conductivity and dielectric behavior of low-temperature phase of α-AgCuPO 4. Ionics. 2016;22:1135–1143.
- Panda B, Routray K L, Karmakar S, et al. Investigation on the optical, electrical, dielectric, and magnetic properties of (1− x) La 0.7 Ca 0.3 MnO 3/xCoFe 2 O 4 nanocomposites. J Mater Sci: Mater Electron. 2019;30:10094–10108.
- Moualhi Y, M’nassri R, Nofal MM, et al. Influence of Fe doping on physical properties of charge ordered praseodymium–calcium–manganite material. Eur Phys J Plus. 2020;135(10):1–23.
- Shukla J, Mishra A. Study of structural, dielectric, and modulus behaviour of barium modified YMnO3 manganite. Mater Today Proc. 2021;46:2189–2192.
- Belik AA, Zhang L, Terada N, et al. A-site-ordered quadruple perovskite manganite CeMn7O12 with trivalent cations. J Solid State Chem. 2020;283:121161.
- Mahmood A, Warsi MF, Ashiq MN, et al. Improvements in electrical and dielectric properties of substituted multiferroic LaMnO3 based nanostructures synthesized by co-precipitation method. Mater Res Bull. 2012;47:4197–4202.
- Manna S, Dutta K, De SK. High dielectric permittivity observed in Na and Al doped NiO. J. Phys. D: Appl. Phys. 2008;41:155416.
- Triyono D, Laysandra H, Liu HL, et al. Structural, optical, and dielectric properties of LaFe1−xMnxO3 (x = 0.00, 0.05, 0.10, 0.15, and 0.20) perovskites. J Mater Sci: Mater Electron. 2019;30:18584–18598.