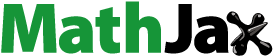
Abstract
Copper ferrite (CuFe2O4, CF) and silver-doped copper ferrite (Ag-CuFe2O4, ACF) were prepared via co-precipitation method. While nano-composite of ACF with rGO was prepared by ultra-sonication approach. Degradation of crystal violet (CV) and benzoic acid (BA) by CF, ACF, and ACF@rGO was conducted under sunlight. Because of tremendous stability and immense surface area of rGO, it shows good ability to transfer electrons during photocatalysis.Band gap for CF and ACF were tuned having values 1.36 and 1.67 eV. Ag-CuFe2O4@rGO has exhibited greater photo-catalytic activity than a bare and doped sample. 82.7% and 48.4% were degradation % of CV and BA by Ag-CuFe2O4@rGO respectively. Ag-CuFe2O4@rGO showed 1.3 times higher efficiency than bare CuFe2O4. ACF was sonicated with rGO and composite was formed which was not reported before and I was the novelty of this paper. The photodegradation ability and cyclic stability etc. was increased after the formation of composite.
1. Introduction
Quality of life has declined over time as a result of growing urbanisation and industry. The hazardous groundwater, fossil fuels, and air have increased as a result of conventional industrial waste disposal methods, which is having an adverse effect on human and animal health [Citation1,Citation2]. All of these drawbacks have led to the development of major environmental problems in the twenty-first century, including pollution and climate change, which pose substantial risks to ecosystems and human health [Citation3–8]. According to a recent World Health Organization report, 3.7 million people died in the twenty-first century as a result of environmental pollution, and 92% of the world's population resides in areas with severe air and water pollution [Citation9,Citation10]. Consequently, the elimination of hazardous waste in water and the atmosphere has emerged as a major national and international priority. Among industrial wastes, dyes and VOCs (volatile organic compounds) play a significant role in water and air pollution [Citation11–14]. AOPs (Advanced Oxidation Processes) have been utilized to remove organic contaminants from water and air [Citation15,Citation16]. Heterogeneous photo-catalysis is considered one of the top AOPs in this area due to its potential applications in the degradation of organic contaminants [Citation16–18]. Among the numerous AOPs, semiconductor-based photocatalysts are an efficient and low-cost technique for water pollution treatment and disinfection. By using photo-catalysis, organic molecules may be entirely reduced to H2O and CO2, with no secondary pollutants produced [Citation19–21]. Metal oxide-based semiconductor photocatalysts' distinctive physicochemical properties, nontoxicity, and exceptional photocatalytic activity have all been extensively utilised in the field of environmental cleanup [Citation22–24]. Titanium dioxide (TiO2) has gotten a lot of attention in recent years because of its low cost, nontoxicity, and chemical inertness [Citation25,Citation26]. The broad band-gap (3.2 eV) is restricted to UV light irradiation. Visible light covers about 43%, while ultraviolet light has about 4% of solar radiations [Citation27,Citation28]. As a result, the design and synthesis of effective visible-light-driven photo-catalysis is a critical study area [Citation29]. La2O3, TiO2, CdO, CeO2, CaO, and ZnO etc. are some metal oxides that are chosen as promising co-catalyst [Citation30–32].
Spinel-like ferrites which are nanostructured (MFe2O4; M = Co2+, Cu2+, Fe2+, Mn2+, Ni2+, Zn2+, and Mg2+) are regarded as a promising and efficient photocatalyst candidate material due to their low cost, ease of production, and distinctive magnetic characteristics. Spinel ferrites generate reactive superoxide radicals during photo-catalysis by absorbing photons from a light source and forming electron–hole pairs [Citation33–36]. For these reasons, spinel-type ferrites in the construction of magnetic photocatalyst nano-composites are quite advantageous [Citation37,Citation38]. As a consequence, combining CdTiO3 with magnetic spinel-type CuFe2O4 nanoparticles improves the effectiveness of photo-induced charge separation, resulting in strong photocatalytic activity. Furthermore, because of its magnetic characteristics, the composite may be magnetically isolated from the reaction mixture [Citation39].
CuFe2O4 has received a lot of interest among ferrites because of its many potentials uses in electronics, sensors, drug administration, magnetic memory, and high-frequency devices [Citation40]. CuFe2O4 also has the benefits of being inexpensive, having great photochemical stability, and having outstanding magnetic characteristics and photo-response [Citation41,Citation42]. CuFe2O4 is a potential photocatalytic and photo-electrochemical catalyst. Furthermore, because of its high reactivity in accelerating the elimination of organic compounds by hydrogen peroxide and peroxymonosulfate, it is an excellent alternative for heterogeneous Fenton oxidation. Copper ferrite (CuFe2O4) has been widely studied and used in many applications due to its appealing, electronic, thermal, magnetic, and catalytic properties [Citation43]. In particular, it has been used in a variety of catalytic applications, including thermal catalysis, photocatalytic pollutant degradation, and photocatalytic hydrogen production [Citation41,Citation44–48].
Silver (Ag) as a doped material has gained attractive attention. It improves the photocatalytic activity of semiconductor photocatalysts and lowers the recombination rate of holes and electrons pair [Citation49–51]. By changing or by lowering the band gap energy silver doped material makes a photocatalyst more active in visible light [Citation52]. It also plays a vital role in wastewater treatment. Further, graphene as a promising 2D material has many special properties such as tremendous mechanical, electrical, and thermal properties and has a high surface area. To restore its aromatic structure, GO is reduced to rGO, and rGO with doped material (composite) has a maximum capacity to lower the recombination rate and has an efficient capability to transfer the charge carrier specie [Citation53–56].
In this research, we synthesized CF and ACF via the co-precipitation method, and the ultra-sonication route was for the fabrication of ACF@rGO. The degradation of coloured and colourless organic effluents (crystal violet and benzoic acid) under sunlight by photocatalysts (CF, ACF, and ACF@rGO) was figured out and the photocatalysis mechanism was examined. I have selected this work because it degrades the pollutants from the environment, which causes the pollution. Furthermore, it also used for waste water treatment and most important thing is that it an ecofriendly. Nano composite of silver copper ferrite was made with rGO; it is a novelty of this work. It was not reported before. When composite formed its cyclic stability and photodegradation ability enhances.
2. Experimental section
2.1. Chemicals used
All the required chemicals of analytical grade were bought from Sigma Aldrich Company. CuFe2O4, Ag-CuFe2O4, Ag-CuFe2O4@rGO, GO, and rGO were synthesized through the following precursors. Iron nitrate nonahydrate (Fe (NO3)3.9H2O, ≥98%), copper nitrate (Cu (NO3)2, ≥99.9%), hydrogen peroxide (H2O2, 35%), ammonia (NH3, ≥99.98%), Sulphuric acid (H2SO4, 98%), Silver nitrate (AgNO3, 99.9%), Graphene powder (99%) and Potassium nitrate (KNO3, >99%).
2.2. Synthesis of CuFe2O4, and Ag-CuFe2O4
0.01 mol solution of copper nitrate and 0.02 mol iron nitrate were prepared with distilled water in 100 mL. Both the solutions were mixed and placed under strong magnetic stirring for 30 min. By adding ammonia, the pH of the solution was raised and stirring was continued further for 30 min, and then stirring was stopped. The suspension was heated for 2 h at 95–100°C as mentioned in Figure (a). The prepared solution was placed for cooling. Later, the CF nanoparticles were washed again and again with distilled water to maintain a pH of 7. The sample was dried in an oven and the dry material was crushed and then calcinated for 1 h at 600°C [Citation36]. A suitable amount of silver nitrate (2% Ag doping, 0.02 g in 50 ml of distilled water) was prepared and then added to a solution of copper ferrite. The same procedure was followed further for the synthesis of copper ferrite. The product was silver-doped copper ferrite.
Figure 1. (a) Scheme of fabrication of CuFe2O4, and Ag-CuFe2O4. (b) Scheme of fabrication of Ag-CuFe2O4@rGO.

As silver is not stable in the air it can easily react with atmospheric oxygen and other pollutant in the air as a result silver oxide formed. During synthesis process, in an air tight bag it was stored before use, laboratory was clean and dry, silver was handled by wearing gloves and all the instruments were also dried before adding silver in them.
2.3. Synthesis of reduced graphene oxide (rGO)
Reduced graphene oxide was formed when 30 mL of GO was added with distilled water and it was sonicated for 3 h. 0.225 ml of hydrazine and 1.5 mL of ammonia were added to a sonicated sample. Then stirred the mixture for 2 h. in paraffin oil by and maintained the temperature at 90°C. The rGO was obtained, was in black and finally, it was dried [Citation37].
For the preparation of reduced graphene oxide hydrazine and ammonia are two essential chemicals that are highly used. Oxygen containing functional groups can be removed from GO by the help of powerful reducing agent that is hydrazine. Conductivity and mechanical properties of GO can be improved by a reducing agent (ammonia). rGO can be obtained by these chemicals in the reducing process and these chemicals make it suitable for vast applications.
2.4. Synthesis of Ag-CuFe2O4@rGO
To prepare a nano-composite of silver-doped copper ferrite with reduced graphene oxide ultra-sonication route was used. 0.24 g of Ag-CuFe2O4 was added to 40 mL of distilled water while 0.06 g of rGO was also added to 40 mL of distilled water. Both solutions were placed for sonication for 1 h at 60°C. Afterward, to get a homogenized mixture both the solutions were dissolved and sonicated for 2 h as shown in Figure (b). At last, it was placed for drying at 80°C.
3. Characterization
For the identification of the functional group of fabricated samples FT-IR (Fourier transform spectroscopy) was carried out through the Shimadzu IR Affinity-1S spectrometer. By using a dual beam UV-Visible spectrophotometer carry-60 the absorption spectra and efficiency of the photocatalyst were identified. The structural confirmation of fabricated samples was analysed by using x-ray diffraction via Lab XRD-6100 (Cu-kα source and λ = 1.54A°). SEM was used for the analysis of surface topology via FEI Inspect S50 SEM. At room temperature, all the characterizations were done.
4. Photocatalytic activity
The photocatalytic efficiency of prepared photocatalysts (CF, ACF, and ACF@rGO) was investigated by using a coloured organic dye (crystal violet) and colourless organic effluent (benzoic acid) under sunlight. 0.015 g of fabricated catalyst was added in 40 mL of stock solution of 8 ppm of crystal violet and 50 mL of 10 ppm of benzoic acid. For 1 h, all the beakers containing a catalyst and solution of coloured dye and colourless organic compound were placed in the dark for stirring. 6 mL of the solution was extracted from the reaction after 1 h for further processing. The beakers were placed for 160 min in sunlight for further degradation. After every 20 min. the 6 mL of solution was taken out and centrifuged. By UV-Visible spectrophotometer the absorption spectra of each catalyst were collected.
Following equations were used for the calculation of % degradation of dyes [Citation57]
(1)
(1) A0 is the absorbance value at 0 min and the absorbance value at t min is At.
The fabricated sample rate constant was found by using eq.2.
(2)
(2)
5. Result and discussion
5.1. Analysis of functional group
The range of FT-IR spectra is from 400–4000 cm−1. Fourier transform infrared spectroscopy was used for the recognition of functional groups. From 400–600 cm−1 presence of copper and ferrite was depicted. While the band around about 443.7 cm−1 ascribed the presence of metal cations in octahedral sites and the band at about 600 cm−1 assigned the metal cations in tetrahedral sites. 3437 cm−1 showed the presence of water molecules this band is due to the stretching of H2O and O-H molecules. While the band around 1630 cm−1 referred to the bending of the water molecule. The bands observed at around 1070, 1216 and 1418 cm−1show that co-precipitated samples were attributed to and
[Citation36]. As the FT-IR spectrum of all the photocatalyst samples is similar but in graph c there was a peak of carbon dioxide at 2358 cm−1 it indicates that the copper ferrite structure was uniform by incorporating Ag and rGO nanosheets. All the plotted data of CF, ACF, and ACF@rGO are presented in Figure (a–c).
5.2. Optical analysis
With the help of a UV-Visible spectrophotometer the absorption spectra of all the samples were drawn. By using absorption spectra, the optical analysis was calculated for fabricated nano-materials and their composite. It is from 200 to 800 nm and shown in Figure (a–c). The spectra were observed at 354 and 432 nm for CF and ACF, whereas at 274 nm the sharp absorption peak at π- π*was observed due to aromatic C = C, and due to the C = O bond at 397 nm the n- π* absorption peak was analysed.
5.3. X-Ray Diffraction analysis
For the identification of the spinal structure and crystalline nature of a prepared material X-ray diffraction was used. Herein Figure (a–c) XRD pattern of copper ferrite, silver doped copper ferrite, and their composite were shown. Several intense diffraction 2θ angles were observed in Figure (a) at 32.05°, 45.9°, 61.87°, and 64.3°are attributed to planes (103), (303), (521), and (440) respectively (Ref No. 01-072-1174). But some peaks of CuO were also present in Figure (a) at 27.6° (220), 35.4° (311), 38.4° (200), and 57.04° (511) [Citation58]. In Figure (b) AgCF XRD pattern also possessed the same peaks as that of pristine copper ferrite. Both owned the same tetragonal structure, which means that the structure of copper ferrite did not manipulate by a dopant like silver. The extra peak at 26.6° was detected in the pattern of ACF@rGO which indicated the characteristic peak of rGO. Phase purity and high crystal structure were shown by sharp peaks with high intensity. It is shown in Figure (c). By using a Mathematical equation the specific surface area was detected [Citation59].
(3)
(3) The specific surface area of Copper ferrite was 152 gm−2.
5.4. SEM analysis
Surface morphology can be detected by employing scanning electron microscopy. Figure (a) shows the SEM profile of CF which showed that it had nano-particle morphology and exhibits the agglomeration of particles. Figure (b) represents the SEM image of ACF it showed that it also had also nano-particles which are almost similar to the bare one.
The nanoparticle size for copper ferrite was observed as 12 nm. While Figure (c) represented the surface of ACF@rGO which showed that there were rGO sheets between nanoparticles.
5.5. Band-gap analysis
The reaction of the photo-catalyst towards the photo-degradation of coloured and colourless compounds can be explained better with the help of a band gap. It is a vital technique and is needed for the transfer of e- from VB to CB of semiconductor material. 1.23 eV to 3.26 eV is the band gap range for semiconductors. By Tauc plot the band-gap energy of prepared material is calculated [Citation60].
(4)
(4) Whereas in the above equation, α is the absorption spectra, ν represents light energy, h is the Planck’s constant, and band gap energy is shown by Eg. Tauc plot is drawn between hυ (x-axis) and (αhυ)n y-axis. Copper ferrite showed band gap energy of 1.36 eV. While the band gap energy of silver-doped copper ferrite is 1.67 eV [Citation61]. The doped sample shows wider band gap value than a pristine sample. It showed that larger Eg of ACF (doped) material makes it more efficient for electron and hole transfer. The bandgap of silver-doped copper ferrite shows that in the visible region, light absorbance enhances and it reveals that a doped sample can be used as a photocatalyst efficiently.
Therefore, the band-gap energy depends upon the following factors such as size, shape, and dimensions. Bandgap plots are shown in Figure (a,b).
6. Photocatalysis
Crystal violet as a coloured pollutant and benzoic acid as a colourless effluent was used to check out the photocatalytic ability of the prepared catalyst. The % degradation of effluents was figured out by using a UV-Visible spectrophotometer. With time the absorption of organic effluents declines. Firstly, the reaction was placed in the dark for 1 h after that the reaction was placed in sunlight for further completion of the reaction.
6.1. Photo-degradation of Crystal violet (CV)
8 ppm solution was prepared in pure water to scrutinize the photocatalytic capability of synthesized material for photo-degradation of CV. Afterward 6 mL of the solution was extracted after every 20 min and then centrifuged. Absorption spectra of CF, ACF, and ACF@rGO are present in Figure (a–c) [Citation57]. Percentage degradation of CV by CuFe2O4, Ag-CuFe2O4, and Ag-CuFe2O4@ rGO is 66.7%, 75.3% and 82.7%. As Ag-CuFe2O4@ rGO has a greater % degradation value than doped and undoped.
Figure 7. Degradation profile of crystal violet in the presence of visible light by (a) CF, (b) ACF, and (c) ACF@rGO.

Kinetics plots are exhibited in Figure . Rate constant of CV by prepared photocatalyst CuFe2O4, Ag-CuFe2O4, Ag-CuFe2O4@ rGO are 0.0059, 0.0077, 0.0092 min−1. The rate constant of composite is higher. It means that ACF@rGO (composite) has shown tremendous results.
Figure 8. (a) % degradation Vs time plot of CV degradation by CF, ACF, and ACF@rGO. (b) At/A0 vs time plot of C.V (c) Kinetics of fabricated photocatalysts.

The rate constant and percent degradation of crystal violet by prepared photocatalysts comparison is presented in Table and Figure (a,b). Further a comparison is also added to show how our work is different from the reported literature in Table .
Table 1. Kinetics parameters of CF, ACF, and ACF@rGO nanocomposite.
Table 2. Comparison of % removal of crystal violet (CV) by different photocatalysts.
6.2. Photo-degradation of Benzoic acid (BA)
10 ppm of BA was mixed in distilled H2O. In the same way, 6 mL of solution was withdrawn and then centrifuged and absorption spectra were measured. Benzoic acid as a colourless organic effluent was used to analyse the photocatalytic effectiveness of the prepared photocatalyst. The absorption spectra of photo-catalysts decline with time as shown in Figure (a–c). The % degradation of benzoic acid by CF, ACF, and ACF@rGO is 29.5%, 39.7%, and 48.4%. Composite has shown again better results. The rate constant and percent degradation of benzoic acid by prepared photocatalysts comparison is presented in Table .
Table 3. Kinetic parameters of CF, ACF, and ACF@rGO.
In Figure (a) Bar graphs of % degradation and Figure (b,c) kinetics plots are shown. It has the rate constant for benzoic acid by CuFe2O4, Ag-CuFe2O4, and Ag-CuFe2O4@ rGO are 0.0021, 0.0026, and 0.0037 min−1. Also, Comparison graphs are added for % degaradation and rate constant (Figure ).
6.3. Stability and magnetic ability
The reproducibility of the fabricated nanocomposite was very significant. The cyclic experiment was performed three times and shown in Figure . Tremendous stability and reusability of Ag-CuFe2O4@rGO are illustrated.
Moreover, by a magnetic stirrer, the magnetic separation of the prepared nanocomposite has been observed. It was an easy way to isolate and recycle the photocatalyst and represented in Figure .
7. Photocatalytic mechanism
A supposed mechanism is recommended for the photo-degradation of crystal violet by the fabricated catalyst. Under sunlight, electrons get excited and transfer from the valence band to the conduction band of the photo-catalyst. In the valence band holes are created. For an excellent photocatalyst, the recombination rate of electrons and holes pair must be low. Electrons from the conduction band of ACF move towards the rGO layer and are trapped by it, as a result, the recombination layer decreases. Whereas it will enhance the photoactivity (Figure ).
Afterward, oxygen is reduced to in the conduction band due to electrons [Citation51]. Oxidation of H2O into OH radicles took place in the valence band because of the presence of holes. The organic dye is degraded to CO2 and H2O. The mechanism is explained from Equations (5)–(11)
(5)
(5)
(6)
(6)
(7)
(7)
(8)
(8)
(9)
(9)
(10)
(10)
(11)
(11)
8. Conclusion
ACF@rGO can degrade textile dye (coloured and colourless organic effluents) effectively. By the co-precipitation method, the synthesis of the pristine and doped sample was done successfully. Nano-composite with rGO followed the ultra-sonication route. Characterization of the fabricated sample was done via different techniques. FT-IR was used to figure out the functional groups of synthesized samples. There are few chances for rejoining of hole and electron after doping. The rate constant of crystal violet and benzoic acid by Ag-CuFe2O4@rGO were 0.0092 and 0.0037 min−1. The degradation % of crystal violet by CF, ACF, and ACF@ rGO was 66.7%, 75.3%, and 82.7%. 29.5%, 39.7% and 48.4% were % degradation of benzoic acid by CuFe2O4, Ag-CuFe2O4 and Ag-CuFe2O4@rGO. To build a healthy and safe environment Ag-CuFe2O4@rGO has the potential to solve the issues that are connected with environmental pollution and it behaves as a promising material.
Acknowledgements
The authors express their gratitude to Princess Nourah bint Abdulrahman University Researchers Supporting Project number (PNURSP2023R42), Princess Nourah bint Abdulrahman University, Riyadh, Saudi Arabia.
Disclosure statement
No potential conflict of interest was reported by the author(s).
References
- Adil M, Rahman A, Zulifqar S, et al. Facile synthesis of binary metal substituted copper oxide as a solar light driven photocatalyst and antibacterial substitute. Advance Powder Technology 2021;32:940–950.
- Bashir S, Jamil A, Amin R, et al. Hydrothermally synthesized Gd-doped BiSbO4 nanoparticles and their graphene-based composite: A novel photocatalytic material. J Solid State Chem. 2022;312:123217.
- Ali H, Khan E. Environmental chemistry in the twenty-first century. Environ Chem Lett. 2017;15:329–346.
- Shakhashiri BZ, Bell JA. Climate change and our responsibilities as chemists. Arab J Chem. 2014;7:5–9.
- Bashir B., Khalid M. U., Aadil M. et al. CuxNi1-xO nanostructures and their nanocomposites with reduced graphene oxide: Synthesis, characterization, and photocatalytic applications. Ceramics International. 2021;47(3):3603–36013.
- Adil M, Zulfiqar S., Warsi M. F. et al. Mesoporous and macroporous Ag-doped Co3O4 nanosheets and their superior photo-catalytic properties under solar light irradiation. Ceramics International. 2021;47(7):9806–9817.
- Wang J, Wu W, Rong F. Development and tri-objective exergoeconomic-environmental optimization of a novel molten carbonate fuel cell-based system for power, hydrogen and freshwater tri-generation. Int J Hydrogen Energy. 2021;46:20715–20731.
- Etminan M, Nabiyouni G, Ghanbari D. Preparation of tin ferrite–tin oxide by hydrothermal, precipitation and auto-combustion: photo-catalyst and magnetic nanocomposites for degradation of toxic azo-dyes. J Mater Sci: Mater Electron. 2018;29:1766–1776.
- Munir S., Rasheed A., Zulfiqar S. et al. Synthesis, characterization and photocatalytic parameters investigation of a new CuFe2O4/Bi2O3 nanocomposite: Ceramics International. 2020;46(18):29182–29190.
- Adil M, Mahmood M., Warsi M. F. et al. Fabrication of MnO2 nanowires and their nanohybrid with flat conductive matrix for the treatment of industrial effluents. Flat Chem. 2021;30:100316.
- Kousar T., Aadil M., Zulfiqar S. et al. Wet-chemical synthesis of nanostructured Ce-doped mixed metal ferrites for the effective removal of azo dyes from industrial discharges. Ceramics International. 2022;48(18):11858–11868.
- Cardon D. Natural dyes, sources, tradition, technology and science, 2007, 268.
- Kamal MS, Razzak SA, Hossain MM. Catalytic oxidation of volatile organic compounds (VOCs) – a review. Atmos Environ 2016;140:117–134.
- Riaz S, Ashraf M, Hussain T, et al. Functional finishing and coloration of textiles with nanomaterials. Color Technol. 2018;134:327–346.
- Yehia FZ, Kandile NG, Badawi AM, et al. Glutamic acid modified Fenton system for degradation of BTEX contamination. Clean–Soil Air Water (Basel). 2012;40:692–697.
- Cheng R, Fan X, Wang M, et al. Facile construction of CuFe2O4/gC 3 N 4 photocatalyst for enhanced visible-light hydrogen evolution. RSC Adv. 2016;6:18990–18995.
- Farhadi S, Siadatnasab F. Sonocatalytic degradation of organic pollutants by CdS nanoparticles hydrothermally prepared from cadmium (II) diethanoldithiocarbamate. Desalin Water Treat. 2017;66:299–308.
- Sillanpää ME, Kurniawan TA, Lo W-h. Degradation of chelating agents in aqueous solution using advanced oxidation process (AOP). Chemosphere. 2011;83:1443–1460.
- Sepahvand S, Farhadi S. Fullerene-modified magnetic silver phosphate (Ag3PO4/Fe3O4/C60) nanocomposites: Hydrothermal synthesis, characterization and study of photocatalytic, catalytic and antibacterial activities. RSC Adv. 2018;8:10124–10140.
- Chen X, Shen S, Guo L, et al. Semiconductor-based photocatalytic hydrogen generation. Chem Rev 2010;110:6503–6570.
- Marszewski M, Cao S, Yu J, et al. Semiconductor-based photocatalytic CO2 conversion. Mater Horiz. 2015;2:261–278.
- Pang YL, Lim S, Ong HC, et al. Research progress on iron oxide-based magnetic materials: synthesis techniques and photocatalytic applications. Ceram Int. 2016;42:9–34.
- Liu Xueqin., Iocozzia J., Wang Y. et al. Noble metal–metal oxide nanohybrids with tailored nanostructures for efficient solar energy conversion, photocatalysis and environmental remediation. Energy & Environmental science . 2017 2):402–434.
- Karthikeyan C, Arunachalam P, Ramachandran K, et al. Recent advances in semiconductor metal oxides with enhanced methods for solar photocatalytic applications. J Alloys Compd. 2020;828:154281.
- Thompson TL, Yates JT. Surface science studies of the photoactivation of TiO2 new photochemical processes. Chem Rev 2006;106:4428–4453.
- Guo Q, Zhou C, Ma Z, et al. Fundamentals of TiO2 photocatalysis: concepts, mechanisms, and challenges. Adv Mater. 2019;31:1901997.
- Morikawa T, Asahi R, Ohwaki T, et al. Band-gap narrowing of titanium dioxide by nitrogen doping. Jpn J Appl Phys. 2001;40:L561.
- Kalathil S, Khan MM, Ansari SA, et al. Band gap narrowing of titanium dioxide (TiO2) nanocrystals by electrochemically active biofilms and their visible light activity. Nanoscale. 2013;5:6323–6326.
- Lakhera SK, Watts A, Hafeez HY, et al. Interparticle double charge transfer mechanism of heterojunction α-Fe2O3/Cu2O mixed oxide catalysts and its visible light photocatalytic activity. Catal Today. 2018;300:58–70.
- Ikram M, Haider A, Bibi ST, et al. Synthesis of Al/starch co-doped in CaO nanoparticles for enhanced catalytic and antimicrobial activities: experimental and DFT approaches. RSC Adv. 2022;12:32142–32155.
- Guo H, Zhang W, Sun Y, et al. Double disks shaped ZnO microstructures synthesized by one-step CTAB assisted hydrothermal methods. Ceram Int. 2015;41:10461–10466.
- Wahab R, Khan F, Singh R, et al. Utilization of photocatalytic ZnO nanoparticles for deactivation of safranine dye and their applications for statistical analysis. Physica E. 2015;69:101–108.
- Shetty K, Renuka L, Nagaswarupa H, et al. A comparative study on CuFe2O4, ZnFe2O4 and NiFe2O4: morphology, impedance and photocatalytic studies. Mater Today Proc. 2017;4:11806–11815.
- Mathew DS, Juang R-S. An overview of the structure and magnetism of spinel ferrite nanoparticles and their synthesis in microemulsions. Chem Eng J. 2007;129:51–65.
- da Silva FG, Depeyrot J, Campos A, et al. Structural and magnetic properties of spinel ferrite nanoparticles. J Nanosci Nanotechnol. 2019;19:4888–4902.
- Kiani A, Nabiyouni G, Masoumi S, et al. A novel magnetic MgFe2O4–MgTiO3 perovskite nanocomposite: rapid photo-degradation of toxic dyes under visible irradiation. Compos B: Eng. 2019;175:107080.
- Kefeni KK, Msagati TA, Nkambule TT, et al. Spinel ferrite nanoparticles and nanocomposites for biomedical applications and their toxicity. Mater Sci Eng C. 2020;107:110314.
- Vestal CR, Zhang ZJ. Effects of surface coordination chemistry on the magnetic properties of MnFe2O4 spinel ferrite nanoparticles. J Am Chem Soc 2003;125:9828–9833.
- Jahanara K, Farhadi S. A magnetically separable plate-like cadmium titanate–copper ferrite nanocomposite with enhanced visible-light photocatalytic degradation performance for organic contaminants. RSC Adv. 2019;9:15615–15628.
- Ghobadi M. Based on copper ferrite nanoparticles (CuFe2O4 NPs): catalysis in synthesis of heterocycles. J Synthetic Chem. 2022;1:84–96.
- Masoumi S, Nabiyouni G, Ghanbari D. Photo-degradation of azo dyes: photo catalyst and magnetic investigation of CuFe2O4–TiO2 nanoparticles and nanocomposites. J Mater Sci: Mater Electron. 2016;27:9962–9975.
- Zhu Z, Li X, Zhao Q, et al. Photocatalytic performances and activities of Ag-doped CuFe2O4 nanoparticles. Mater Res Bull. 2013;48:2927–2932.
- Chowdhury A, Balu S, Venkatesvaran H, et al. Facile construction of CuFe2O4/pg-C3N4 pn heterojunction with boosted photocatalytic activity and sustainability for organic degradation reactions under visible-light. Surf Interfaces. 2022;34:102329.
- Masunga N, Mmelesi OK, Kefeni KK, et al. Recent advances in copper ferrite nanoparticles and nanocomposites synthesis, magnetic properties and application in water treatment: review. J Environ Chem Eng. 2019;7:103179.
- Kodasma R, Palas B, Ersöz G, et al. Photocatalytic activity of copper ferrite graphene oxide particles for an efficient catalytic degradation of Reactive Black 5 in water. Ceram Int. 2020;46:6284–6292.
- Jing P, Li J, Pan L, et al. Efficient photocatalytic degradation of acid fuchsin in aqueous solution using separate porous tetragonal-CuFe2O4 nanotubes. J Hazard Mater 2015;284:163–170.
- Fu Y, Chen Q, He M, et al. Copper ferrite-graphene hybrid: a multifunctional heteroarchitecture for photocatalysis and energy storage. Ind Eng Chem Res. 2012;51:11700–11709.
- Ghanbari D, Sharifi S, Naraghi A, et al. Photo-degradation of azo-dyes by applicable magnetic zeolite Y–Silver–CoFe2O4 nanocomposites. J Mater Sci: Mater Electron. 2016;27:5315–5323.
- Ahmad I. Comparative study of metal (Al, Mg, Ni, Cu and Ag) doped ZnO/g-C3N4 composites: efficient photocatalysts for the degradation of organic pollutants. Sep Purif Technol. 2020;251:117372.
- Yang X, Cui H, Li Y, et al. Fabrication of Ag3PO4-graphene composites with highly efficient and stable visible light photocatalytic performance. ACS Catal. 2013;3:363–369.
- Surendra B. Green engineered synthesis of Ag-doped CuFe2O4: characterization, cyclic voltammetry and photocatalytic studies. J Sci: Adv Mater Dev. 2018;3:44–50.
- Ahmad M, Ahmad I, Ahmed E, et al. Facile and inexpensive synthesis of Ag doped ZnO/CNTs composite: study on the efficient photocatalytic activity and photocatalytic mechanism. J Mol Liq. 2020;311:113326.
- Mensah B, Konadu DS, Nsaful F, et al. A systematic study of the effect of graphene oxide and reduced graphene oxide on the thermal degradation behavior of acrylonitrile-butadiene rubber in air and nitrogen media. Sci Afr. 2023;19:e01501.
- Paul S, Mondal S, Mitra I, et al. Effects of graphene oxide and reduced graphene oxide on the energy storage capacity of a short-chain dyad. A comparative study with the pristine dyad. J Mol Struct. 2023;1274:134548.
- Xu J, Shu R, Wan Z, et al. Construction of three-dimensional hierarchical porous nitrogen-doped reduced graphene oxide/hollow cobalt ferrite composite aerogels toward highly efficient electromagnetic wave absorption. J Mater Sci Technol. 2023;132:193–200.
- Hao R, Li D, Zhang J, et al. Green synthesis of iron nanoparticles using green tea and its removal of hexavalent chromium. Nanomaterials. 2021;11:650.
- El-Bahy SM, Arshad J, Munir S, et al. Improved photocatalytic performance of a new silver doped BiSbO4 photocatalyst. Ceram Int. 2022;48:23914–23920.
- Naghikhani R, Nabiyouni G, Ghanbari D. Simple and green synthesis of CuFe2O4–CuO nanocomposite using some natural extracts: photo-degradation and magnetic study of nanoparticles. J Mater Sci: Mater Electron. 2018;29:4689–4703.
- Arshad J, Alzahrani FMA, Munir S, et al.. Integration of 2D graphene oxide sheets with MgFe2O4/ZnO heterojunction for improved photocatalytic degradation of organic dyes and benzoic acid. Ceramic International. 2023;49:18988–19002.
- Munir S, Baig MM, Zulfiqar S, et al. Anisotropic thermal diffusivity and conductivity in SiC/SiC tubes studied by infrared imaging and X-ray computed tomography. Ceram Int. 2022;48:21717–21727.
- Karim KMR, Tarek M, Sarkar SM, et al. Photoelectrocatalytic reduction of CO2 to methanol over CuFe2O4@PANI photocathode. Int J Hydrogen Energy. 2021;46:24709–24720.
- Habib MA, Muslim M, Shahadat MT, et al. Photocatalytic decolorization of crystal violet in aqueous nano-ZnO suspension under visible light irradiation. J Nanostructure Chem. 2013;3:1–10.
- Tavakoli-Azar T, Mahjoub AR, Sadjadi MS, et al. Improving the photocatalytic performance of a perovskite ZnTiO3 through ZnTiO3@S nanocomposites for degradation of crystal violet and Rhodamine B pollutants under sunlight. Inorg Chem Commun. 2020;119:108091.
- Tadjarodi A, Imani M, Kerdari H, et al. Preparation of CdO rhombus-like nanostructure and its photocatalytic degradation of azo dyes from aqueous solution. Nanomater Nanotechnol. 2014;4:16–16.