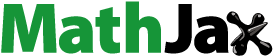
Abstract
The electrolysis of water for substantial production of hydrogen and oxygen with very high purity is considered as a potentially practical technology. In the present study, we have fabricated a graphene-oxide hybrid zinc-selenide electrocatalyst and characterized it using different techniques. XRD analysis confirms the phase purity and crystal structure of the electrocatalyst. Furthermore, the elemental arrangement and morphology of the electrocatalyst were examined by electron dispersive X-ray spectroscopy (EDX) and scanning electron microscopy (SEM). To check the activity of electrocatalysts through electrochemical cyclic voltammetry (CV), linear cyclic voltammetry (LSV), and impedance analysis have represented that catalyst activation occurs at lower applied potential because of the decrease of electro-negativity of the anion. The ZnSe also displayed efficient OER catalytic performance in a basic medium, making it a very energetic bifunctional electro-catalyst for water splitting with a cell voltage of 1.38 V and an overpotential of 155 mV at 10 mA/cm2.
1. Introduction
Recently science and technology have played a vital role in raising the living standard of the people. The advancement in science and technology during the last few years brought a revolution in technical progress, influencing every part of life [Citation1–4]. In order to maintain this revolution, sustainable energy resources are the basic need of today’s world. Intense and fast scientific research to cop is the only solution to this problem of depleting energy resources [Citation5]. At present, various types of energy resources are being utilized in the present world. Fossil fuel is the primary source of present progress, but it is a non-renewable energy resource [Citation6–8].
The world’s energy crisis has worsened due to rapid mining and fossil fuel use to keep people alive [Citation9,Citation10]. If we want clean energy sources with a longer shelf life, we need to design a system that can create and consume energy sustainably [Citation11]. Because it is simple to convert a high energy density that emits low CO2 and is beneficial for the environment, hydrogen energy is an excellent choice [Citation12,Citation13]. Therefore, this alternative energy source has the greatest choice for displacing fossil fuels [Citation14–16]. Currently, hydrogen energy can be produced in large quantities using steam created from fossil fuels. Hydrogen can be produced through the electrochemical splitting of water, a low-cost energy and environment-friendly technique [Citation17,Citation18]. The electrochemical process for splitting water comprises the hydrogen evolution reaction (HER) and the oxygen evolution reaction (OER). The slowest OER which is the four-electron transfer approach because it is tough [Citation19,Citation20]. It is stared as the most significant component of the electrolytic water reaction and requires a significantly larger overpotential than HER [Citation21,Citation22]. A catalyst can accelerate chemical processes, which also accelerates the OER. [Citation23,Citation24]. At the time, noble metal catalysts like RuO2 and IrO2 were the most difficult ones available in the market [Citation25,Citation26]. However, because they are expensive and unreliable, it is difficult to implement/utilize them for large-scale production. Due to this, it is still essential to find OER catalysts that are less expensive, longer lasting, and have higher electro-catalytic properties [Citation27,Citation28].
Hence transition metals and their oxides, phosphates, nitrides, sulphides, and selenides, which are abundant on earth and have many of the same properties as precious metals, have recently become more well-liked [Citation29,Citation30]. Ni, Co, and Fe-based catalysts performed well in both HER and OER due to the ability to modify their 3D electronic structures, the presence of spin electrons, and the variety of their crystal structures [Citation31]. Feng et al. successfully fabricated Co9S8 nano-spheres operating OER in Alkaline conditions. With just 285 mV over potential, they could achieve a 10 mA/cm2 current density [Citation32]. Yang et al. used synchronous chemical vapour deposition to produce a NiS2@NiSe2/NF with rich edges. At the point in the OER process where the rate is determined, the contact between the NiS2 and NiSe2 phases lowered the barrier of energy reaction and enhanced the rate of electron allocation [Citation33]. Li et al. produced CoMn double hydroxide nanospheres with holes. Nanospheres can be used for OER and water splitting because of their large electrochemical surface area and capacity to move Co and Mn ions. It has been shown that this objective can be met by modifying the electrical structure of materials to make them more active [Citation34]. Zhao et al. developed a complex Mn-modified CoSe2 nano-sheet and successfully proved its efficacy. This made it possible to change the atom’s grouping and electrical structure. To make this material more active, carbon nano-tubes, heteroatoms, and other active components are commonly added [Citation35]. Xia and his coworkers used a sequential combination technique on graphene-loaded carbon fabric to produce cobalt selenide nano-particles. Layers, a sizable specific surface area, and compatibility with CoSe2 make CoSe2@VG/CC ideal for OER and Na+ flux [Citation36]. The electron spin orbits in Co 3d and Se 4p overlap, causing this to happen. Because it includes Co2+ and Co3+, the nonstoichiometric compound CoSe2 exhibits strong electrostatic interactions with OH- groups [Citation37]. Mn-Co0.85Se/NiSe2/NF exhibits excellent OER performance. The synthesized catalyst showed alkaline stability of at least 20 hours at 20 mA/cm2 current density, a dominant 33.26 mV dec-1 Tafel slope, and a low 175 mV overpotential [Citation38].
Chalcogenides with comparable stoichiometry electrodeposited on a nickel foam electrode displayed the lowest overpotential at 10 mA/cm2 current density [Citation39]. This observation supports the idea that catalytic activity increases as the covalency around the transition metal increases. Numerous studies on hybrids that combine graphene with various materials have been conducted, and their photo-electrochemical, electrochemical, and catalytic performance has been noticeably improved [Citation40,Citation41]. This is due to the increased active surface areas and efficient electron transport provided by graphene. The results above strongly imply that adding e- donor functional groups may greatly improve the electrochemical action and provide a creatively constructed pathway for effective electrocatalysts for water electrolysis that have a prodigious capacity to replace Pt-based electro-catalysts [Citation42,Citation43].
Here, we described a straightforward hydrothermal method for creating composites made of zinc selenide and graphene oxide (ZnSe/GO). GO may be effectively reduced during production, and ZnSe nanocrystals were also produced and distributed over the graphene sheets. The electrochemical experiment’s output shows a 155 mV overpotential with a 97 mV/dec Tafel slope value at a 10 mA/cm2 current density. This discovery opens up new paths for an investigation into OER catalytic activity and emphasizes the importance of cheap, accessible, and highly effective catalysts. The potential creation technique was also disclosed, and this study of ZnSe/GO may serve as an excellent paradigm for future design and research into graphene-chalcogenide composites.
2. Experimental section
2.1. Materials
The chemicals used to synthesize electrochemical electrodes were of analytical grade and were used as received without additional purification. Nickel foam and selenium powder (Se) were purchased from Sigma Aldrich. Zinc chloride (84%, ZnCl2), potassium hydroxide (53%, KOH), and hydrazine hydrates (50%, N2H4) were bought from Fisher Scientific. Sulphuric acid (67%, H2SO4), phosphoric acid (H3PO4), potassium per magnate (96%, KMnO4), hydrogen peroxide (74%, H2O2), hydrochloric acid (80%, HCl), and graphite powder (GP) were purchased from Merck. The deionized water (DI) is used as a universal solvent to prepare solutions.
2.2. Hydrothermal synthesis of ZnSe
For the synthesis of ZnSe, 0.56 g (0.1 M) of KOH and 0.14 g (0.1 M) of ZnCl2 were dissolved in deionized water. Then 0.349 g Se powder in the above solution was added, followed by the addition of 2 ml of hydrazine monohydrates in the above solution and stirred for one hour on a magnetic hot plate at a suitable temperature. The mixture was put into a stainless-steel autoclave sealed in a 50 ml Teflon tube. In the heating oven, the autoclave was kept at 200°C for 3 hours and at room temperature, the autoclave was cooled. The precipitate from this course was collected, washed three times with DI, and then treated with ethanol. The resulting precipitate was dehydrated for 8 hours at 70°C in the oven. The sample was ground before being put away for more examination and characterization.
2.3. Synthesis of graphene oxide (GO) by improved hammers method
In this technique, 27.0 ml of H2SO4 and 3.0 ml of H3PO4 by volume ratio 9:1 was mixed and stirred on a magnetic stirrer for several minutes at a suitable temperature and then add 0.225 g graphite powder in the mixing solution under vigorous stirring. The mixture was then placed in an ice bath below 20°C and slowly added 1.32 g potassium per magnate. This solution was stirred for 7 h till the solution became grey. H2O2 (0.675 ml) was dropped slowly and stirred for 25 minutes to remove the excess potassium permanganate.
The residue was cleaned again with HCl and DI water 3 times after the supernatant was decanted. The precipitates of graphene oxide were dehydrated in an oven for 24 hours at 90°C. The precipitates were finely ground and saved for further investigation.
2.4. Preparation of ZnSe/GO nanocomposite
For the synthesis of the ZnSe/GO nano-composite, 0.58 g of already synthesized ZnSe and 0.63 g of GO were added in 30 mL of deionized water, and the resultant solution was gently stirred for 15 minutes and then placed into a 50 mL autoclave and heated for 8 hours at 160°C. The resultant product was centrifuged, washed with ethanol (C2H5OH), and then placed in an oven to desiccate overnight at 110°C.
2.4. Preparation of electrode
The electrode for electrochemical analysis was prepared by tacking nickel foam 1×1 cm piece, which is sonicated for 20 minutes in a mixture of deionized water and alcohol. The paste was fabricated by grinding the electrode material (0.5 mg) in 1 microliter of deionized water and then placed on the nickel foam, which was then used as the working electrode. The synthesized electrode was placed in an oven for 30 minutes for drying.
2.5. Physical characterization
For determining the product structure and crystal phases, an X-ray powder diffractometer (XRD, Philips, X'pert, system employing CuKα radiation) was employed [Citation44]. The Debye-Scherer equation was employed to find the size of crystallite from the XRD patterns:
(1)
(1)
Using scanning electron microscopy, the sample’s morphology was analyzed (FESEM, ZEISS, SIGMA VP-500). For compositional analysis, the materials were examined using energy-dispersive spectroscopy (EDX; FESEM, TE-SCAN). Utilizing N2 adsorption-desorption at liquid nitrogen temperature, textural parameters such as specific surface area (SSA) and pore size were analyzed.
2.6. Electrochemical catalytic activity
Utilizing a typical three-electrode cell with a potentiostat, chronoamperometry was used to determine the stability of the catalyst, and linear sweep voltammetry (LSV) and cyclic voltammetry (CV) were utilized to measure the OER and HER catalytic activity. The reference, counter, and working electrodes, correspondingly, were Ag/AgCl, Pt rods, and electrodeposited ZnSe/GO sheets on NF. All potentials attained against Ag/AgCl were transformed to the reversible hydrogen electrode (RHE):
(2)
(2)
The measured film deposition area was used to calculate the electrochemical results for all electro-deposition studies. Electrochemical double-layer capacitance was used at various scan rates to determine the catalyst’s ECSA (electrochemically active surface area). Cdl was used to measure the ESCA values in the non-faradic region at different scanning rates. The electrochemical active surface area was calculated with the given equation:
(3)
(3)
(4)
(4)
The specific capacitance (Csp) used to compute the ECSA of the catalyst in an alkaline solution. Based on already published nickel-based OER catalysts, we estimated the value of Csp is 0.04 mF cm2 in the purposes of this work, and Cdl is the double-layer capacitance. The Tafel slope, which may be stated as follows, is a crucial metric to describe the electrocatalytic performance and kinetics of a particular reaction:
(5)
(5)
Where α denote the number of the electrons that takes part in the reaction, η is the overpotential, t is the charge transfer coefficient, j stands for current density, F represents the Faraday constant, and the slope is determined by 2.3RT/nF. Another crucial statistic that may be computed to analyze the OER activity of a catalyst is the turnover frequency (TOF). The number of catalytic cycles the centre per unit of time determines the turnover frequency, which quantifies a catalytic centre’s particular activity for the OER under specified reaction circumstances.
(6)
(6)
Where m depicted the amount of moles of the active catalyst, F is the Faraday constant, and I is the current in amperes.
3. Result and discussion
3.1. Structural and morphological analysis
The XRD measurement examined the crystal structure of composite (ZnSe/GO) nano-structures and its individuals. The powder XRD patterns of the GO, ZnSe and GO/ZnSe nano-composite are exposed in Figure (a). The appearance of the plane (2 0 0) is clear, suggesting the unique plane of GO. A succession of peaks that correspond to the (1 1 1), (2 2 0), (3 1 1), (4 0 0), and (3 3 1) planes of ZnSe are shown in the XRD pattern at 2θ value of 27°, 45.3°, 53.8°, 66.2° and 72.6° of the ZnSe material (matched with the JCPDS# 00-001-0690) [Citation45]. On the other hand, all the peaks of ZnSe, and a large peak corresponding to GO is also visible in the composite material, which verifies the hybrid structure of ZnSe/GO nano-composite.
Figure 1. (a) XRD images of GO, ZnSe and ZnSe/GO and (b–d) SEM images of GO, ZnSe and ZnSe/GO nanocomposite.

The ZnSe/GO hybrid electro-catyst was synthesized by a one-pot hydrothermal process. The morphology of the ZnSe/GO nano-composite was determined by scanning electron microscopy (ZEISS Supra55, SEM) and the result is shown in Figure (b–d) for GO, ZnSe and its nanocomposite. Figure (b) shows the morphology of the GO, which is in the shape of the sheets. The morphology of the ZnSe was in the form of nanorods, as depicted in Figure (c). On the other hand, it is clear that the morphology of the nano-composite that the ZnSe nano-rods were well arranged on the graphene oxide sheet, as given in Figure (d). This particular structure reveals that the ZnSe/GO nano-composite has additional active sites for the electrolyte throughout the electrochemical reaction, which is necessary for the most efficient electro-catalyst.
Figure (a–c) displays the EDX analysis to confirm the elemental composition of the synthesized material. The EDX examination proved that the synthetic material contained Zn, Se, and C, which are the major elements. The investigation verified that the related elements were present in all the fabricated materials for GO, ZnSe, and GO/ZnSe nano-composite, respectively, which confirms the purity of the fabricated materials.
Additionally, nitrogen (N2) adsorption/desorption analysis was used to calculate the porous texture of ZnSe, GO and ZnSe/GO. The surface areas of ZnSe, GO and ZnSe/GO observed by N2 adsorption or desorption were calculated using the Brunauer-Emmett-Teller research. Additionally, as shown in Figure , the presence of pores and holes in the synthesized materials was demonstrated by an increase in nitrogen adsorption as the relative pressure increased. The results of BET demonstrate that ZnSe, GO and ZnSe/GO nano-composite have type IV adsorption isotherms with BET surface areas of 50 cm3/g, 68 cm3/g, and 120 cm3/g, respectively. Due to the presence of graphene oxide as a nano-sheet responsible for extremely high active sites promotes the catalytic oxidation activity that is promising for the electro-catalytic splitting of water, as depicted in (Table ). With the use of the BJH approach, the BET isotherm also provides data regarding the pore size of manufactured samples and the mesoporous sizes of ZnSe, GO and ZnSe/GO as 0.09 nm, 0.105 nm, and 0.175 nm, respectively, as shown in Figure (b).
Figure 3. BET isotherms (a) Specific surface area (SSA) of GO, ZnSe and ZnSe/GO, (b) Pore size distribution of GO, ZnSe and ZnSe/GO.

Table 1. Comparison table of BET specific surface area (SSA) and distributed pore size of ZnSe, GO and ZnSe/GO.
3.2. OER activity
The CV study was performed to find the active surface area of electro-catalytic systems, which should be higher for the efficient catalyst and the roughness of the catalysts [Citation46]. Figure displays the obtained findings from the CV runs, which were taken in the potential range of 0.90 to 1.15 V with various scan rates.
(7)
(7)
(8)
(8)
Where Ageom is the geometric surface area of the electrode, and A is the predicted electro-active surface area of the catalytic electrode. The high roughness surface (Rf) gives more active sites for reaction and enhances the catalyst activity. ZnSe, GO, and ZnSe/GO have Rf values of 218, 732 and 1023, respectively. The hydrothermal reaction-produced ZnSe/GO composites were also examined for electro-activity and contrasted with other materials. The ZnSe/GO nanostructure has the largest electro-active surface area as compared to ZnSe and GO, also illustrated in Figure (a–c), the ECSA of the synthesized electro-catalyst was evaluated with a 0.90 to 1.15 V potential window at various (20, 40, 60, 80, and 100 mV/s) at scan rates. The ECSA investigation was used to calculate the Cdl value (double layer capacitance) of the electrochemical ZnSe, GO and ZnSe/GO electrodes, which are 6.23 mF and 8.71 mF and 15.41 mF, respectively Figure (d–f). The greater Cdl value of ZnSe/GO, which denotes a rapid rate of charge assembly and more active sites manifesting on the electro-catalyst surface, is responsible for the improved electrochemical performance (Table ).
Figure 4. (a–c) CV curves at different scan rates, and (e–f) Cdl of fabricated materials at different scan rates in basic media.

Table 2. Comparison table of ECSA and Cdl values of ZnSe, GO and ZnSe/GO at various scan rates.
The CV and LSV were performed at 10 mV/s sweeping scans in the basic medium for all the electrodes. According to the CV curves in Figure (a), ZnSe/GO exhibits higher efficiency at a 10 mA/cm2 current density vs. Ag/AgCl, but GO and ZnSe exhibits a lower level of catalytic activity under the same circumstances. Figure (b) displays the LSV curves of bare Ni, GO, ZnSe, and ZnSe/GO. When the LSV plots were analyzed, it was shown that bare Ni, GO, ZnSe, and ZnSe/GO all exhibit current densities of 10 mA/cm2, but among all the nano-hybrid showed the high current density. This is a result of the composite material’s high surface area, numerous active sites, and the synergistic interaction between GO and ZnSe. We estimated the Tafel slopes of the produced electro-catalyst materials, as shown in Figure (c), which were derived from the linear parts of the LSV curves in order to further restore the material’s OER catalytic activity. It was demonstrated that ZnSe/GO exhibited a quicker rate of reaction throughout the OER course, and had a lower Tafel slope value of 97 mV/dec, whereas bare Ni, GO, and ZnSe had a higher Tafel slope value of 170 mV/dec, 122 mV/dec and 123 mV/dec. On the other hand, the overpotential of 392 mV, 272 mV, 343 mV and 155 mV, were resulted for bare Ni, GO, ZnSe, and ZnSe/GO, respectively, Figure (d) demonstrating the material’s overpotential at 10 mA/cm2 current density.
Figure 5. (a) Cyclic voltammetry (b) LSV (c) tafel slope (d) overpotential of GO, ZnSe and ZnSe/GO in alkaline media.

The EIS study can explain the kinetics and conductivity of the OER. The Nyquist plots of EIS results are presented in Figure (a). It is evident that ZnSe/GO has a significantly lower charge transport barrier than the other two catalysts. Because ZnSe and graphene oxide sheets have a good connection, the synthetic material has a higher OER rate and good electronic conductivity. One important aspect affecting the actual application of OER electrodes is their cycling capacity. At 10 mA/cm2 current density, we assessed ZnSe/GO cycle stability. The specific capacitances of ZnSe/GO are 95% after 1000 consecutive cycles, as shown in Figure (b).
Figure 6. (a) EIS of GO, ZnSe and ZnSe/GO (b) CV cycles (c) Chronoamperometry stability of ZnSe/GO nanocomposite and (d) XRD analysis before and after stability test.

Long-term stability is necessary for the material to perform better OER activity [Citation47]. By using a chronoamperometry measurement, the stability of the nanocomposite material ZnSe/GO as an effective OER catalyst was examined, and the outcomes are demonstrated in Figure (c). The chronoamperometry curve shows that after 40 h of testing at a potential of 10 mV, little reduction in the current density was seen, confirming the materials possess extremely high stability in the alkaline media (1 M KOH solution). Additionally, there is no discernible difference between the LSV curves acquired after the OER durability and stability test of 50 h and the original curves obtained. The XRD has been performed after the stability test to check the structural stability of the investigated electrocatalyst and found that all the peaks are at the same 2-theta position as were before the stability test which confirmed its good structural stability but a change in the peaks intensities has been observed which may be due to the blockage of the active sites on the surface of the catalyst.
4. Conclusion
ZnSe/GO nanostructure composite material was fabricated using the hydrothermal technique. ZnSe/GO shows a good response towards OER because of the strong bonding between the ZnSe and the graphene sheets, the electro-active sites of the material have a synergistic impact, resulting in a large electro-active surface area and enhanced electrochemical catalytic activity. With a very low starting potential of 1.38 V and an excellent water-splitting capacity in basic media, the catalyst functions as an incredibly effective and inexpensive electrochemical catalyst. The nanocomposite constructed of ZnSe/GO had a reduced overpotential of 155 mV and a lower 97 mV/dec Tafel slope value, according to the electrochemical results. Additionally, the high electron conduction of GO and the major reactive oxygen functional moieties for OER enhance the process of water splitting. Based on these findings, it is suggested that ZnSe/GO is a promising low-cost OER electro-catalyst for alkaline electrolysis. According to our research, the large-scale OER can be driven by the water splitting process using this efficient and novel combination.
Acknowledgment
One of the author (K. M) is thankful to Bahauddin Zakariya University Multan, Pakistan for providing facilities for this work. K.F.F express appreciation to the Deanship of Scientific Research at King Khalid University Saudi Arabia for funding through research groups programme under grant number R.G.P. 2/279/44.
Disclosure statement
No potential conflict of interest was reported by the author(s).
Additional information
Funding
References
- Ganguly S, Das P, Banerjee S, et al. Advancement in science and technology of carbon dot-polymer hybrid composites: a review. Funct Compos Struct. 2019;1:022001
- Holdren JP. Science and technology for sustainable well-being. Science. 2008;319:424–434.
- Stanislaus A, Marafi A, Rana MS. Recent advances in the science and technology of ultra low sulfur diesel (ULSD) production. Catal Today. 2010;153:1–68.
- Islim OF, Cagiltay K. EURASIA J Math Sci Technol Edu. 2016;12:559–567.
- Du C, Li P, Zhuang Z, et al. Highly porous nanostructures: rational fabrication and promising application in energy electrocatalysis. Coord Chem Rev. 2022;466:214604.
- Muritala IK, Guban D, Roeb M, et al. High temperature production of hydrogen: assessment of non-renewable resources technologies and emerging trends. Int J Hydrogen Energy. 2020;45:26022–26035.
- Amin M, Shah HH, Fareed AG, et al. Hydrogen production through renewable and non-renewable energy processes and their impact on climate change. Int J Hydrogen Energy. 2022;7:33112–33134.
- Capellán-Pérez I, Mediavilla M, de Castro C, et al. Fossil fuel depletion and socio-economic scenarios: an integrated approach. Energy. 2014;77:641–666.
- Zou C, Zhao Q, Zhang G, et al. Energy revolution: from a fossil energy era to a new energy era. Natural Gas Industry B. 2016;3:1–11.
- Siddik M, Islam M, Zaman A, et al. Int J Energy Environ Econ. 2021;28:103–119.
- Xu W, Wu K, Wu Y, et al. High-efficiency water splitting catalyzed by NiMoO4 nanorod arrays decorated with vacancy defect-rich NiTex and MoOy layers. Electrochim Acta. 2023;439:141712.
- Zhang L, Liang J, Yue L, et al. Benzoate anions-intercalated NiFe-layered double hydroxide nanosheet array with enhanced stability for electrochemical seawater oxidation. Nano Research Energy. 2022;1:e9120028.
- Fan F, Hui Y, Devasenathipathy R, et al. Composition-adjustable Mo6Co6C2/Co@carbon nanocage for enhanced oxygen reduction and evolution reactions. J Colloid Interface Sci. 2023;636:450–458.
- Zhou S, Tong Q, Yu S, et al. Role of non-fossil energy in meeting China’s energy and climate target for 2020. Energy Policy. 2012;51:14–19.
- Winter C-J. Hydrogen energy — abundant, efficient, clean: a debate over the energy-system-of-change. Int J Hydrogen Energy. 2009;34:S1–S52.
- van Biert L, Godjevac M, Visser K, et al. A review of fuel cell systems for maritime applications. J Power Sources. 2016;327:345–364.
- Chen Z, Wei W, Ni B-J. Cost-effective catalysts for renewable hydrogen production via electrochemical water splitting: recent advances. Curr Opin Green Sust Chem. 2021;27:100398.
- McHugh PJ, Stergiou AD, Symes MD. Decoupled electrochemical water splitting: from fundamentals to applications. Adv Energy Mater. 2020;10:2002453.
- Huang J, Wang Y. Efficient renewable-to-hydrogen conversion via decoupled electrochemical water splitting. Cell Rep Phy Sci. 2020;1:100138.
- Youngblood WJ, Lee S-HA, Maeda K, et al. Visible light water splitting using Dye-sensitized oxide semiconductors. Acc Chem Res 2009;42:1966–1973.
- Fan F, Huang Q, Devasenathipathy R, et al. Composite-structure-defined nitrogen-doped carbon nanocage embedded Co/CoxP for enhanced oxygen reduction and evolution reactions. Electrochim Acta. 2023;437:141514.
- Zheng F, Zhang W, Zhang X, et al. Sub-2 nm ultrathin and robust 2D FeNi layered double hydroxide nanosheets packed with 1D FeNi-MOFs for enhanced oxygen evolution electrocatalysis. Adv Funct Mater. 2021;31:2103318.
- Roger I, Shipman MA, Symes MD. Earth-abundant catalysts for electrochemical and photoelectrochemical water splitting. Nat Rev Chem. 2017;1:1–13.
- Pindur U, Lutz G, Otto C. Acceleration and selectivity enhancement of diels-alder reactions by special and catalytic methods. Chem Rev. 1993;93:741–761.
- Li W, Zhang H, Hong M, et al. Defective RuO2/TiO2 nano-heterostructure advances hydrogen production by electrochemical water splitting. Chem Eng J. 2022;431:134072.
- Wang VCC. Exploring the kinetic and thermodynamic aspects of four-electron electrochemical reactions: electrocatalysis of oxygen evolution by metal oxides and biological systems. Phys Chem Chem Phys. 2016;18:22364–22372.
- Dotan H, Landman A, Sheehan SW, et al. Decoupled hydrogen and oxygen evolution by a two-step electrochemical–chemical cycle for efficient overall water splitting. Nature Energy. 2019;4:786–795.
- Chen S, Kucernak A. Electrocatalysis under conditions of high mass transport rate: oxygen reduction on single submicrometer-sized Pt particles supported on carbon. J Phys Chem B. 2004;108:3262–3276.
- Chen JG. NEXAFS investigations of transition metal oxides, nitrides, carbides, sulfides and other interstitial compounds. Surf Sci Rep. 1997;30:1–152.
- Sun Y, Zhang T, Li C, et al. Compositional engineering of sulfides, phosphides, carbides, nitrides, oxides, and hydroxides for water splitting. J Mater Chem A. 2020;8:13415–13436.
- Theerthagiri J, Lee SJ, Murthy AP, et al. Fundamental aspects and recent advances in transition metal nitrides as electrocatalysts for hydrogen evolution reaction: a review. Curr Opin Solid State Mater Sci. 2020;24:100805.
- Zhang P, Cao M, Feng Y, et al. Uniformly growing Co9S8 nanoparticles on flexible carbon foam as a free-standing anode for lithium-ion storage devices. Carbon. 2021;182:404–412.
- Yang Y, Kang Y, Zhao H, et al. An interfacial electron transfer on tetrahedral NiS2/NiSe2 heterocages with dual-phase synergy for efficiently triggering the oxygen evolution reaction. Small. 2020;16:1905083.
- Zhao J, Chen J, Xu S, et al. CoMn-layered double hydroxide nanowalls supported on carbon fibers for high-performance flexible energy storage devices. J Mater Chem A. 2013;1:8836–8843.
- Zheng W, Li Y, Lee LYS. Earth-abundant metal-based nanomaterials for electrochemical water splitting. In: Wong WY, Dong Q, editors. Functional nanomaterials: synthesis, properties, and applications. Hoboken (NJ): Wiley; 2022. p. 1–40.
- Arif M, Yasin G, Shakeel M, et al. Coupling of bifunctional CoMn-layered double hydroxide@graphitic C3N4 nanohybrids towards efficient photoelectrochemical overall water splitting. Chem–Asian J. 2018;13:1045–1052.
- Shi X, Wang H. Electrochemical transformation of renewable compounds. (FL): CRC Press; 2022, p. 195–236.
- Zhou T, Bai J, Gao Y, et al. Selenide-based 3D folded polymetallic nanosheets for a highly efficient oxygen evolution reaction. J Colloid Interface Sci. 2022;615:256–264.
- Tang S, Zhou Y, Lu X, et al. Surface/interface engineering for fabricating hierarchical Ir doped NiMoO4 covered by CoMn layered double hydroxide toward oxygen evolution reaction. J Alloys Compd. 2022;924:166415.
- Kumar A, Sharma K, Dixit AR. A review of the mechanical and thermal properties of graphene and its hybrid polymer nanocomposites for structural applications. J Mater Sci. 2019;54:5992–6026.
- Wu X, Mu F, Zhao H. Recent progress in the synthesis of graphene/CNT composites and the energy-related applications. J Mater Sci Technol. 2020;55:16–34.
- Son DI, Kwon BW, Park DH, et al. Emissive ZnO–graphene quantum dots for white-light-emitting diodes. Nat Nanotechnol. 2012;7:465–471.
- Ramírez C, Belmonte M, Miranzo P, et al. Applications of ceramic/graphene composites and hybrids. Materials. 2021;14:2071.
- Norby P. Hydrothermal conversion of zeolites: an in situ synchrotron X-ray powder diffraction study. J Am Chem Soc 1997;119:5215–5221.
- Kirsanova M, Nemchinov A, Hewa-Kasakarage NN, et al. Synthesis of ZnSe/CdS/ZnSe nanobarbells showing photoinduced charge separation. Chem Mater. 2009;21:4305–4309.
- Neyerlin K, Bugosh G, Forgie R, et al. Combinatorial study of high-surface-area binary and ternary electrocatalysts for the oxygen evolution reaction. J Electrochem Soc. 2009;156:B363.
- Jin H, Ruqia B, Park Y, et al. Nanocatalyst design for long-term operation of proton/anion exchange membrane water electrolysis. Adv Energy Mater. 2021;11:2003188.