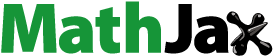
Abstract
Refreshing scents are encapsulated and bonded to the fabric, and when they stay for a long time, they have a positive effect on people. In the study, limonene was microencapsulated with polyurethane-urea, which formed a strong and stable shell, and was fixed to cotton fabric with intermediate binders (butane tetracarboxylic acid, succinic acid, citric acid) containing multiple carboxyl groups at different curing temperatures. The dimensions of the microcapsules, the effect of mixing speed on the size of the capsule, the efficiency of microencapsulation and the effectiveness of intermediate binders, the fastness of microencapsulated fabrics to UV rays, rubbing and washing were investigated. The following results were obtained: Average microcapsule size 3.18–13.70 µm, microencapsulation efficiency of 73.16–96.24%, active substance loss of 5.7–15.1% for 8 weeks at 20°C and under UV rays 3.32%, binder BTCA that best binds capsules to fabric and capsules adherence to fabric up to 8 washes.
1. Introduction
Scents plays an important role in consumer satisfaction and influences further consumption of products. In humans, the impact of odours on psychology is quite high. Fragrances can reduce anxiety, help recall personal memories, enhance mood, facilitate optimism, stimulate vitality and inspire innovative thinking [Citation1,Citation2]. Smell may also play an active role in certain circumstances and social experiences in making decisions about persons, objects and locations [Citation3–5]. Fragrances that are used in shopping centres impact people's shopping habits and purchase preferences, and fragrant items are more preferable than other products [Citation6]. In a study conducted, it has been observed that the customers prefer the same products in the scented places to the products in the odourless places [Citation7,Citation8]. The power of smell on buying preferences and customer behaviour is confirmed, which further illustrates the major influences of scents in individual behaviours [Citation9].
Changes have been observed in innovation products with the discovery of new technology and scientific methods. Nowadays, people spend large sums of money on personal care products, health, and comfort products [Citation10–12]. In order for industrial products to compete on a global basis, new products must have different or superior aspects. Microencapsulated products not only add superiority to the materials they are used, but also increase the added value of the product [Citation13]. Microencapsulation of active substances has different purposes for various sectors. The aim of encapsulation is to protect the core material from external factors such as moisture, air, heat, friction, light and micro-organism but also to protect the coexisting substances from physical and chemical effects [Citation14–16].
In the textile sector, by attaching different active substances to textile surfaces, it adds functionality to fabrics. chief among them is resistance to UV rays, antibacterial, anticancer, removal of harmful chemicals from liquid fuels, antiviral, antifungal, antibacterial, fire retardant, conductive fabrics are available [Citation17–30].
Due to their volatile properties, fragrances cannot be directly applied to the textile material and are encapsulated in the polymeric wall material in order to prevent their evaporation due to heat, moisture and other external factors. Capsules that trap the fragrance substance, after being attached to the textile material, break off as a result of temperature, pH, humidity and mechanical effects and release this scent substance, which they have trapped [Citation31,Citation32].
The proper protection under environmental conditions of the encapsulated active material depends on the stability of the capsule shell. Polyurethane capsule shell is a polymeric structure formed by isocyanate, glycol and amine groups and shows good resistance to external conditions. Polyurethan-urea (PUU) emerging as useful biomaterials for biomedical applications, drug release systems, adhesives, agricultural control, food, fragrance, automotive industry, thermal insulation and industrial applications because of their good compatibility with core material, their synthetic versatility, excellent mechanical properties and good biocompatibility. Their potential uses for a number of pharmaceutical, medical and cosmetic applications, such as drug encapsulation and controlled release, have been discussed [Citation33–38].
The possible chemical formulation processes of the received PUU capsules synthesized with water-soluble diols/diamines. Due to the higher reactivity of the amine groups compared to the hydroxyl (the reactivity decreases as follows: R–NH2 > NH3 > Ph–NH2> R–OH > H2O > R–NH–CO–NH–R > R–NH–COOR > R–COOH), the reaction between the diamine and diisocyanate runs more rapidly, especially at room temperature [Citation39].
A modified fabric should preserve this feature for a long time. this depends on the fabric, the microcapsule shell strength and the intermediate binder that connects the microcapsule to the fabric. In previous studies, microencapsulation experiments were carried out with compounds containing multiple isocyanate groups. However, no research has been done on the microcapsule efficiency of the change of alcohol and amine groups in the PUU, which forms the microcapsule shell. In this study, a robust microcapsule shell, high microencapsulation efficiency and an intermediary that keeps the microcapsules on the fabric for a long-time during washing have been revealed.
2. Material and method
Polyvinyl alcohol (PVA, Aldrich, Mw:130000), Triglyceride (Lipestrol), Takenate D-110N(Mitsui, 75%), Guanidine carbonate (Sigma-Aldrich, 99%), PEG-400(Sigma-Aldrich), Limonene (MG International Fragrance Company, Gebze, Turkey), Dibutyltin dilaurate (DBTL, sigma, 95%) Guanidine carbonate (Sigma-Aldrich, 99%) Hydrazine hydrate (HYD, Sigma-Aldrich, 50-60%), Butane tetracarboxylic acid(BTCA, Sigma-Aldrich, 99%), Succinic acid(SUC, Sigma-Aldrich, ≥ 99.0%), Citric acid (CA, Sigma-Aldrich, ≥ 99.5%), Sodium hypophosphite monohydrate (Sigma-Aldrich, ≥ 99.0%). Chemicals of analytical purity were used in the experiment without any prior purification.
2.1 Microencapsulation
Microencapsulation was carried out by making some changes in Mertgenc (2021) et al., method (37). 3.0 grams of PVA were dissolved in 10.0 mL of water. 0.8 g takenate d-110N was dissolved in 5.0 mL scents and mixed with 1.0 mL triglyceride. Water and scents solutions were blended and homogenized at 3000–9000 rpm to obtain 1–50 µm droplets. pH of these emulsified solution was adjusted 7.0 with 2% NaOH, which were put into a reactor and then mixed at 600 rpm for 5 min at room temperature. Then, 3.0 mL PEG 400 of 10% and 30 µL dibutyltin dilaurate (DBTL) mixture was added dropwise in one hour. Meanwhile, the temperature was gradually increased from 35°C to 55°C, and the mixture was stirred at this temperature for an hour. 3.0 mL guanidine carbonate solution of 1% added to the solution in the reactor, and mixed at the same temperature for another 1.0 h. Finally, 2.5 mL of a 3 percent hydrazine hydrate solution was added, stirred, and maintained at the same temperature for an additional hour. The mixture was then cooled to room temperature and kept there for analysis. The substances used in the production of microcapsules and their amounts are given in Table . Figures and show the microencapsulating scheme and the microcapsule manufacturing technique, respectively.
Table 1. PUU shell prepared in different molar ratios with isocyanate/glycol/amines.
2.2 Impregnation of microspheres to textile
Attaching the scent-containing PUU microcapsules to the fabric was applied with a slight change in the impregnation process performed by Aracil et al. [Citation40]. Microcapsules dispersion was prepared in a glass vessel. Three tests were performed in the study. The first one did not contain binders, while the others used BTCA, CA and SA. All the samples contained 5% of microcapsules, 4% of binder (excluding control group) and 2% of catalyst over all weight of fibre. The working conditions performed are summarized in Table .
Table 2. Laundry condition.
2.3 Microcapsulation efficiency
To make the calibration chart, limonene was dissolved in hexane at different concentrations (10 ppm-50 ppm). While limonene absorbs UV light at a wavelength of 222–226 nm. It was made in a spectrophotometer at a wavelength of 224 nm. The PUU microcapsule solution was filtered using blue band filter paper, and the capsules were dried in a 75°C for 1 d. In a closed test tube, 0.3 g of dry capsules were added to 6.0 mL of hexane, and the limonene in the capsule was transported to the hexane phase within 24 h. The test tube was centrifuged at 3000 rpm for 4.0 min after shaking twice for 3 min in the vortex device, and the hexane phase was separated.
100.0 mL of limonene-containing hexane phase was taken and mixed with 5.0 mL of hexane in a second test tube. This procedure was repeated and carried out for a total of five dilutions. Following the measurement of the absorbance value of the diluted solution in the UV device, the amount of limonene in the dilute solution was calculated using the calibration graph, and the limonene in the PUU microcapsule was calculated using backward calculations. Using the equation below, the microencapsulation efficiency (ME) of limonene in microcapsules was computed.
2.4 Washing durability
Limonene microencapsulated fabrics fixed on cotton fabric were subjected to washing durability test using a normal washing machine containing 2 g/L detergent (ECE non-phosphate reference detergent) and following the standard evaluation procedure (AATCC) for 20 min. The durability of the microcapsules application to textile fabric was evaluated by measuring the effect of washing cycles on treated cotton according to the ISO 105-C10 standard [Citation41].
After 1,2, … , and 8 wash cycles, each sample was examined for odour. Within a day after washing, washed samples were tested by identifying a probable odour with a judge scratching with fingernail and then sniffing the evaluation sample. The presence of the fragrance was judged by four jury members who answered “yes” or “no”. A light microscope was also used to check for the presence of microcapsules on the fabric.
2.5 Rubing test
For the rubbing test, dry fabric samples were subjected to rubbing with dry cotton fabric, using a standardized Crockmeter tester according to the TS 717 EN ISO 105-X12 [Citation42]. They were rubbed for 10, 20 and 30 cycles by a finger covered with a fabric sample at a pressure of 9 N.
2.6 Limonene release from microcapsules under UV rays
PUU microcapsules on slides and fixed on cotton fabric were exposed to UV rays of 2 × 18 W for 15 days from a height of 15 cm. Weight loss was measured every three days.
2.7 Characterization
The stability of emulsified drops, the structures during the formation of the PUU microcapsules, the time of formation of stable capsules, the formation stage of the capsules’ shell, and the impurities in the environment were observed with the Olympus CH20 optical microscope. The images of microcapsules were taken with 4X and 10X magnification.
FT-IR was performed in 3000–400 cm−1 operating range, by forming KBr pellets. Empty microcapsules without limonene were completely dried, then mixed with 2.0–6.0 mg KBr which powdered in mortar to form discs under pressure to make measurements in the IR device. The liquid materials used in the shell material of the capsule were dripped over the KBr pellets which formed under pressure to measure their spectra.
After drying and coating with gold, the encapsule samples were analysed with SEM instrument. Microencapsule size distribution was analysed in aqueous medium using the Malvern Nano-Zs device.
TGA device was used to measure mass change in decomposition and evaporation of microencapsules with and without (empty capsule) fragrance. From the microencapsules containing and not containing fragrance, 2.0–5.0 mg of samples were taken separately, and the temperature was raised to 450°C as to increase by 10°C per minute in the nitrogen environment. Then, the mass change was measured and recorded as a function of temperature.
The limonene-PUU microencapsules were vacuum-filtered on blue filter paper and stored in the lab for 24 h before being dried in a 75oC oven. At the end of this time, 2.2 g microcapsule samples were placed in a petri dish and the mass loss in the capsules was measured at 20oC every seven days at 8 week.
3. Results and discussion
3.1. Infrared spectra
The structure of the shell of PUU was confirmed by FT-IR spectra and chemical analysis. Multiple hydrogen bonds can be formed by two types of proton donors (urethane N-H and urea N-H groups) and three types of proton acceptors (urethane C = O, urea C = O, and C–O–C groups) in the PUU. The hydrogen bond geometry of urea groups can be quite different from that of urethane groups. One urea group is made up of one carbonyl group and two N-H groups. By contrast, one urethane group has only one N-H group. The FT-IR spectra of isocyanate and PUU limonene free microencapsules are presented in Figure , and Table summarizes the major peaks of isocyanate and PUU shell structure. Numerous infrared vibrations are visible in spectrums; N-H and O-H stress peaks between 3200 and 3600 cm−1, urea carbonyl peaks between 1550–1600 cm−1, and 1650–1700cm−1 (urethane carbonyl) peaks with a considerable vibration intensity indicate that the reaction has occurred. The amide's vibration peaks appeared at 1420 and 1260 cm−1. The vibration observed in 1080 cm−1 is from the ether group C–O–C stress. Isocyanates stretch at 2265 cm−1, but the limonene-free shell of the microcapsule doesn't have that vibration. This shows that all the takenate d 110N is used up by reactions with glycol, GC, and HYD.
Table 3. Main peak assignments for isocyanate (takenate d 110) and the PUU shell.
3.2 TGA studies
In Figure , the red line represents the microcapsules of limonene, the blue represents the 9000 rpm obtained microcapsules, the bordeaux, 3000 rpm obtained microcapsules, and the black line, the polyurea capsule shell, shows the mass loss with temperature increase. It has been understood that the mass loss of limonene odour occurs between 50 and 180°C, the loss of active ingredient in small capsules produced with maximum efficiency is between 120 and 230°C, and the loss of active ingredient in large capsules obtained with maximum efficiency is between 170 and 240°C. Figure shows that mass loss in the crust material begins at 210oC and continues at a rate of 75% up to 500oC. The shells of the big capsules are more resistant than the shells of the small capsules, and they begin to release the active core substances at greater temperatures.
Figure 4. TGA graph of poliurea capsule shell (black line), limonene (red line), big microcapsule produced at low mixing speed (3000 rpm, bordeaux line) and small microcapsule at high mixing speed (9000 rpm, blue line).

The mass losses shown in Figure are from microcapsules containing the active ingredient limonene in the core, which were produced in Experiments 1, 2, and 3. The percentage of polyurethane is higher in the capsule shell obtained in test one, and this ratio is the opposite in the shell obtained in test three. There is mass loss in three distinct areas in all of these capsules, and this feature is more obvious in Experiments 3 and 4. It is thought that the first mass loss seen in all three capsules is due to the polyurethane shell, the mass loss seen in the second degradation is due to the polyurea shell formed later, and the final mass loss is due to large-molecule polyurethaneurea.
Figure 5. TGA graph of limonene (blue line), microcapsules produced experiment 2 (dark lue line), experiment 3 (green line) and experiment 4 (bordeaux line).

Figure shows how the mass losses of only polyurethane and polyurea microcapsule-shelled microcapsules change with increasing temperature. It has been determined that the polyurethane capsules leave the core active material between 110 and 320oC, but the polyurea capsules leave it between 170 and 310oC. However, this graph shows that the polyurea shell degrades earlier than the polyurethane. Furthermore, it is understood from this figure that polyurea capsules have higher active substance storage properties than polyurethane.
Figure 6. TGA graph of poliurea capsule shell (black line), limonene (red line), polyurea-shell microcapsules (bordoaux line) and polyuretahane-shell microcapsules (green line).

The amine and glycol groups have a great effect on the formation of the microcapsule shell structure. Amine groups react with isocyanate more rapidly than glycol groups [Citation40]. Here, the reaction rate can be adjusted by slowly introducing the amine solution into the cyanide medium. The amine-formed shells have been found to contain the odour component better than the glycol-formed shells. It was found that capsules having a high amine group in the capsule shell had a high encapsulation efficiency (96.24%, Figure ) and a low loss of active ingredient (5.7%, Figure ) over time at room temperature. When capsule shells created with hexa-methylene diisocyanate, toluene diisocyanate, and takenate d 110 are examined, it is seen that those made with takenate have a smoother and less brittle surface.
3.3. Size distribution in microcapsules
Figure shows different particle size distributions of Limonene-PUU microcapsules. Particle size analysis on the Malvern Nano-Zs device reveals a primary mode in the intermediate range 2.18–7.25 µm for 9000 rpm, 3.80–14.24 µm for 6000 rpm, and 4.36–34.67 µm for 3000 rpm. As seen at all three mixing speeds, most of the limonene-PUU microcapsule fell into the size range around the mean diameter, while the average particle size decreased with increasing mixing speed. Mertgenc et al. found that as the mixing rate increased, the mean diameter of the microcapsules decreased and their scale distribution got narrower [Citation37].
3.4 Yield in microcapsules
Limonene encapsulation was assessed by measuring the quantity of limonene contained in each sample and comparing it to the total amount of limonene added at the beginning. Figure shows the computed values for limonene mass in PUU microcapsules. Based on GC mmol ratios in the PUU shell, the yields in the first, second, third, fourth, and fifth tests were 73.16, 85.31, 91.05, 95.16, and 96.24 percent, respectively.
Figure 8. Percent yields of microcapsules containing guanidine carbonate (GC) different molar ratios in the PUU-capsule shell. (1: GC free; 2: 0.4 mmol; 3:0.6 mmol; 4:0.8 mmol; 5:1.2 mmol, mean ± SD, n = 3).

The maximum efficiency was observed in the fifth experiment at 96.24 percent, according to these findings the capsule shell includes 1.2 mmol GC but no PEG 400. The lowest efficiency was found in capsules containing maximum PG 400 no GC in the capsule shell.
The fundamental reason for the low encapsulation efficiency is that the PVA material utilized as an emulsifier cannot keep the emulsified droplets stably following the formation of an oil/water emulsion. PUU capsule shell formation rate reaction of amine groups is faster than that of glycols [Citation39]. When the amount of amine concentration increased from 0.4 to 0.8 mmol, and glycol concentration decrease from 0.8 to 0.4 mmol, the encapsulation efficiency increased from 85.31% to 95.16%. This finding reveals that the emulsified droplets, which were prone to exploding due to their inability to build a suitably solid shell with polyurethane, produced a more rigid shell with polyurea, preventing the emulsified droplets from bursting.
3.5 Loss of limonene in microcapsules over time
The Limonene-PUU microencapsules were vacuum-filtered on blue filter paper and stored in the laboratory for 24 h. Figure illustrates the mass changes during an 8-week period at room temperature after drying in an oven at 75o C for 24 h. According to Figure , in PUU microcapsules containing 1.2 mmol GC with at least 5.7% mass loss, 7.01% mass loss in the 0.8 mmol GC, 8.52% mass loss in the 0.6 mmol GC, 12.87% mass loss in the 0.4 mmol GC and maximum mass loss of 15.1% were observed in GC-free capsules. It was found that there is a correlation between the encapsulation efficiency (Figure ) and the essence loss (Figure ) in the microcapsules While the loss of essence in high-yield PUU capsules was low over time, the loss of essence in low-yield capsules was high.
Figure 9. Weight loss of PUU-microcapsules with different molar ratios of glycol in the microcapsule shell at room temperature (mean ± SD, n = 3)

Figures and show microcapsules exposed to UV light and mass loss in microcapsules, respectively.
Figure 11. Weight loss of PUU-microcapsules over time at room temperature under UV irradiation (mean ± SD, n = 3).

Every volatile liquid has a vapour pressure. The microcapsule shell is weakened by friction. The microcapsules exploded easily due to the vapour pressure created by the limenon substance in the microcapsule and the effect of UV rays Figure .
3.6 Weight loss over time in microencapsulated fabrics exposed to UV rays
In Figure , the percent mass losses of fabrics fixed at 150oC using TCA intermediate binder and rubbed and untreated fabrics under UV light are given. The mass losses in PUU-microcapsules on cotton fabrics exposed to UV rays for 15 days were found to be 3.87% in normal fabric, 5.19% in 10 rubbings, 9.39% in 20 rubbings, and 14.64% in 30 rubbings, respectively. According to these results, it was concluded that friction causes damage to the microcapsule shells, and this damage breaks down faster with UV rays because the mass loss of normal microcapsules [Citation37] under the same conditions was 3.38%.
Figure 13. Stability of PUU-microcapsules on cotton fabrics over time at room temperature under UV irradiation (mean ± SD, n = 3).

As can be seen in Figures and , it was found that both microencapsulated cotton fabric and microcapsules exposed to UV rays yellowed. This is mainly because the benzene ring is directly attached to the isocyanate group, so the large π-bond on the benzene ring forms a conjugate with the adjacent NCO. Under the influence of light, heat, oxygen, and especially ultraviolet light, it causes the urethane bond to decompose and produce aromatic amines. After the benzene core on aromatic amines is oxidized and rearranged, yellowing will occur by forming chromophoric groups such as quinone structures.
3.7. SEM images of microcapsules
A low molecular weight prepolymer is formed during the interfacial polymerization of isocyanate (takenate d 110) with alcohol and amine in the aqueous phase. The small molecular weight prepolymer formed surrounds the emulsified droplets and the chain length of the polymer begins to increase. By reacting with the -OH and -NH2 groups of the triple isocyanate in Takenate, PUU is formed, which develops the wall of the emulsified droplet with three-dimensional cross-links.
The capsule shell was observed to be spherical, smooth, and large microencapsulated had more depressions on their surfaces based on the data obtained from SEM images. As seen in Figure , there is an inverse relation between mixing speed and microcapsule diameter size. It has been observed that mixing speed has significant effects on capsule size and the effects of amines and glycols on capsule shell formation. Many studies have reported that mixing speed has an inverse effect on microcapsule size [Citation36–38]. In our study, it was found that the average size of the 3000 rpm capsule changed from 13.18 µm to 9000 rpm by 4.7 µm (Figure ).
Figure 14. SEM images of microcapsules at 500 X magnification and different mixing speeds (A: 9000 rpm, B: 6000 rpm, C: 3000 rpm).

While many parameters influence the lifetime of the capsules on the fabric, the key ones are the type of fixator that binds the capsules to the fabric and the curing temperature at a specific time interval. If the fixing agent does not effectively hold the capsule during washing, the capsules will separate from the tissue and the finished sample will lose its odorous character. To avoid this, the capsule must be attached to the textile at multiple locations, with additional polymer chains linked for a strong chemical binding. It usually results in greater binder reactions and improved capsule fixation under vigorous curing conditions over a period of time. During the curing process at high temperatures, the odour in the capsule will evaporate from the weak capsules and reduce their effectiveness. Theoretically, stronger curing conditions might result in more vaporization of the capsule fragrance. For this reason, the fastness of the capsules and the loss of odour from the capsules should be considered when choosing the curing conditions. Li et al. tested various fixators and curing procedures to attach the capsules to the fabric in their investigation and found that the acrylic binder was the strongest fixator for bonding the microcapsules to the fabric [Citation42].
Limonene-PUU microcapsules were fixed on cotton fabric with the different fixators in a certain time(in Table ). The fragrance durability of the finished samples was evaluated through washing and odour assessment. After a few washing cycles, the samples preserved their smell under these curing conditions. According to the SEM images in Figure , the best method of attaching the microcapsules to the cotton fabric was obtained with BTCA as fixator, 150oC temperature and 2 s curing time. Micro-encapsulated fabrics were washed in a household washing machine using the sensitive washing programme, and results are listed in Table .
Table 4. Microcapsules’ bonding methods to fabric and how many washing cycles microcapsules can withstand.
SEM images of cotton fabrics with and without microcapsule additives subjected to the friction test are shown in Figure . Here, the durability of the capsules on the fabric is demonstrated using BTCA, which binds the capsules best to the fabric (highest washing fastness). These images show the changes in microcapsule added and undoped cotton fabric after rubbing for 10, 20, and 30 cycles at 9 N pressure. In Figure , the effects of three different conditions on cotton were investigated. In the first of these, it was checked whether there was any deformation on the fabric in different cycles by applying 9N force (A, B, C, D). It was seen that different friction numbers did not change the surface of the cotton fabric according to both physical examination and SEM images. In the second, the situation of microcapsules in fabrics that applied 9N force at different friction numbers to cotton fabrics attached to PUU-microcapsules was investigated (E,F, G, and H). It has been revealed that the 9N force applied to the microcapsule-attached fabric breaks the microcapsules, and the breakage in the capsules increases as the number of cycles increases. In the third study, the stability of the microcapsules on the fabric was investigated by giving 2 × 18 W UV light from a height of 15 cm for 15 days, similar to the ones performed in the second study (J, K, L, and M). It is understood from the decrease in the microcapsules on the cotton fabric that the UV rays applied to the E, F, G, and H microcapsule fabrics in Figure break the microcapsules.
Figure 15. SEM images of microencapsules fixed on cotton at different binders and temperatures (A: binder free at 110°C; B: BTCA at 110°C; C: BTCA at 150°C; D: SA at 110°C; E: SA at at 150°C; F: CA at 110°C; G: CA at at 150°C).

Figure 16. SEM images of treated and untreated cotton fabrics(A: no rubbs, B: 9N 10 rubbs, C: 9N 20 rubbs, D: 9N 30 rubbs, E: microcapsules 150°C fixed + no rubbs, F: microcapsules 150°C fixed + 9N 10 rubbs, G: microcapsules 150°C fixed + 9N 20 rubbs, H: microcapsules 150°C fixed + 9N 30 rubbs, J: microcapsules 150°C fixed + UV, K: microcapsules 150°C fixed + 9N 10 rubbs + UV, L: microcapsules 150°C fixed + 9N 20 rubbs + UV, M: microcapsules 150°C fixed + 9N 30 rubbs + UV).

Figure 17. Showed the XRD pattern of the cellulosic structure of the treated and untreated cotton fabric(CF)(CF1: normal CF, CF2: CF + TCA-binder; CF3: CF + microcapsule, CF4: CF + microcapsule + UV, CF5: CF + microcapsule + 30 rubbs, CF 6: CF + microcapsule + 30 rubbs + UV).

Figure shows the XRD patterns on the modified and unmodified cotton fabrics. The 2θ peaks are located at around 15.12o, 17.74o, 23.87o and 34.65o which correspond to the (110), (110), (200), and (004) planes, planes, ensuring the crystalline structure of the cellulosic modified and untreated cotton fabric. XRD patterns show that adding microcapsules to cotton fabric did not cause any change in the crystal structure of the cotton fabric during fixation [Citation42]. XRD patterns of unmodified and modified cotton fabrics were taken, and no difference on the peaks was obtained between them, probably due to the amount of PUU microcapsules on the cotton fabric being at an undetectable level.
4. Conclusion
Limonene scent was encapsulated in high yield in different sizes with different crosslinker ratios by the interfacial polymerization method, and the encapsulation efficiency was between 73.16 and 6.24%. While the loss of active substance in microcapsules was between 5.7 and 15.1% in 8 weeks at 20oC, it was 3.38% in 15 days at the same temperature under UV rays. Low size microcapsules with high encapsulation efficiency were fixed on cotton fabric with three different intermediate binders and catalysts at 150oC in 2 min. Small size microcapsules with high encapsulation efficiency were fixed on cotton fabric with three different intermediate binders and catalysts at 150oC for 2 min, and it was revealed that the best interconnector agent was TCA. These capsules were kept on the fabric for up to 8 washes in the normal washing machine. Various techniques have been used to characterize both PUU microcapsules and their stability on cotton fabrics, including FTIR, SEM, TGA, UV rays, rubbing, and washing tests. Microcapsule shell strengths were evaluated according to TGA results, and it was observed that the active substance in polyurea microcapsules was released at a higher temperature than polyurethane microcapsules, and the shells of large diameter microcapsules were found to be more robust than small microcapsules. It has been observed that microcapsules are broken as a result of rubbing in different cycles with 9 N force on microencapsulated fabrics, and UV lights on microcapsule fabrics with and without rubbing break the microcapsules. Another result is that although UV rays cause yellowing in both microcapsules and microencapsulated fabrics, the loss of active substance is low. As a result, microencapsulated fabrics with higher washing fastness can be obtained with better fixative agents.
Acknowledgements
The author would like to thank to Mitsui Chemicals Inc. and MG International Fragrance Company for providing Takenate D-110 and Limonene substances supporting this work. We would like to thank Sennur Alay Aksoy, who helped us with the rubbing tests.
Disclosure statement
No potential conflict of interest was reported by the author(s).
References
- Darabi K, Mirabi VR. The effect of ambient scent on consumer experience: evidence from mobile industry. Manag Sci Lett. 2018;8:1199–1206. doi:10.5267/j.msl.2018.8.005
- Chatterjee S. Olfactory marketing and market innovation: a systematic literature review and agenda for future research direction. Int J Manag Concepts Philos. 2022;15:37–56. doi:10.1504/IJMCP.2022.120546
- Pierce JD, Ulrich PM, Cohen AB. The role of odors in influencing individual preferences for people. Objects, and Places, J Comp Psychol. 2004;118:14–19. doi:10.1037/0735-7036.118.1.14
- Bercik J, Neomániová K, Mravcova A, et al. Review of the potential of consumer neuroscience for aroma marketing and Its importance in various segments of services. Appl Sci. 2021;11:1–20.
- Cao MT, Dong QN. Effect of ambient scents and behavior responses of customer. Rev Argent Clin Psic. 2021;15:133–144.
- Biswas D, Szocs C. The smell of healthy choices: cross-modal sensory compensation effects of ambient scent on food purchase. J Mark Res. 2019;56:123–141. doi:10.1177/0022243718820585
- Lin SH, Sun X. Ethnic differences in consumer preference for scented textile produc. J Text Appar Technol Manag. 2018;10:3.
- De Luca R, Botelho D. The unconscious perception of smells as a driver of consumer responses: a framework integrating the emotion-cognition approach to scent marketing. AMS Revi. 2021;11:145–161. doi:10.1007/s13162-019-00154-8
- Madzharov AV, Block LG, Morrin M. The cool scent of power: effects of ambient scent on consumer preferences and choice behavior. J Mark. 2015;79:83–96. doi:10.1509/jm.13.0263
- Zev L. Microencapsulation: an overview of the technology landscape. In: R Meyer, editor. Delivery system handbook for personal care and cosmetic products. William Andrew Publishing; Norwich, 2005. p. 181–190.
- Encarnaçao T, Pais A, Campos MG, et al. Endocrine disrupting chemicals: impact on human health, wildlife and the environment telma. Sci Prog. 2019;102:3–42. doi:10.1177/0036850419826802
- Costa R, Moggridge GD, Saraiva PM. Chemical product engineering: an emerging paradigm within chemical engineering. AIChE J. 2006;52:1976–1986. doi:10.1002/aic.10880
- Michael H. Chemical product engineering—the third paradigm. Comput Chem Eng. 2009;33:947–953. doi:10.1016/j.compchemeng.2008.11.013
- Gupta S, Khan S, Muzafar M, et al. Encapsulation: entrapping essential oil/flavors/aromas in food. In: Grumezescu AM, editor. Nanotechnology in the agri-food industry, encapsulations. Academic Press; London, 2016. p. 229–268.
- Alic B, Sebenik U, Krajnc M. Microencapsulation of Butyl Stearate with Melamineformaldehyde resin: effect of decreasing the pH value on the composition and thermal stability of microcapsules. Express Polym Lett. 2012;6:826–836. doi:10.3144/expresspolymlett.2012.88
- Babazadeh A, Jafari SM, Shi B. Encapsulation of food ingredients by nanophytosomes. In: Jafari SM, editor. Nanoencapsulation in the food industry. lipid-based nanostructures for food encapsulation purposes. Academic Press; London, 2019. p. 405–443.
- Gharibzahedi SMT, George S, Greiner R, et al. New trends in the microencapsulation of functional fatty acid-rich oils using transglutaminase catalyzed crosslinking. Compr. Rev. Food Sci. 2018;17:274–289. doi:10.1111/1541-4337.12324
- Cheng W, Liu W, Wang Q. Durable hydrophobic and antibacterial textile coating via PDA/AgNPs/ODA in situ assembly. Cellulose. 2022;29:1175–1187. doi:10.1007/s10570-021-04339-y
- Martinez MA, Gende LB, Alvarez VA, et al. Biofunctional textiles: functional polymer-carriers with antiviral, antibacterial, antifungal, and repellent activity. In: Mishra AK, Hussain CM, editor. Biobased materials. Singapore: Springer; 2023. p. 227–258.
- Ehsanimehr R, Sonnier P, Najafi F, et al. Layer-by-layer polymer deposited fabrics with superior flame retardancy and electrical conductivity. React Funct Polym. 2022;173:1–11. doi:10.1016/j.reactfunctpolym.2022.105221
- Xiong W, Jiang Y, Huang G, et al. Preparation of graphene conductive fabrics and the study of their degradation behavior. Coatings. 2022;12:1–13. doi:10.3390/coatings12101432
- Emam HE, Zaghloul S, Ahmed HB. Full ultraviolet shielding potency of highly durable cotton via self-implantation of palladium nanoclusters. Cellulose. 2022;29:4787–4804. doi:10.1007/s10570-022-04567-w
- Emam HE, El-Rafie MH, Rehan M. Functionalization of unbleached flax fibers by direct integration of nano-silver through internal and external reduction. Fibers Polym. 2021;22:3014–3024. doi:10.1007/s12221-021-0993-y
- Mehta S, MacGillivray M. Aromatherapy in textiles: A systematic review of studies examining textiles as a potential carrier for the therapeutic effects of essential oils. Textiles. 2022;2:29–49. doi:10.3390/textiles2010003
- Emam HE, El-Shahat M, Taha M, et al. Microwave assisted post-synthetic modification of IRMOF-3 and MIL-68-NH2 onto cotton for fuel purification with computational explanation. Surf. Interfaces. 2022;30:1–17. doi:10.1016/j.surfin.2022.101940
- Marae IS, Sharmoukh W, Bakhite EA, et al. Durable fluorescent cotton textile by immobilization of unique tetrahydrothienoisoquinoline derivatives. Cellulose. 2021;28:5937–5956. doi:10.1007/s10570-021-03871-1
- Emam HE, El-Shahat M, Hasanin MS, et al. Potential military cotton textiles composed of carbon quantum dots clustered from 4–(2, 4–dichlorophenyl)–6–oxo–2–thioxohexahydropyrimidine–5–carbonitrile. Cellulose. 2021;28:9991–10011. doi:10.1007/s10570-021-04147-4
- Ahmed HB, Abualnaja KM, Ghareeb RY, et al. Technical textiles modified with immobilized carbon dots synthesized with infrared assistance. J Colloid Interface Sci. 2021;604:15–29. doi:10.1016/j.jcis.2021.07.014
- Tariq Z, Izhar F, Zunera GMD, et al. Fabrication of highly durable functional textile through microencapsulation of organic citronella oil. Ind Crops Prod. 2022;190:115878. doi:10.1016/j.indcrop.2022.115878
- Sumathi S, Ancy T. Microencapsulation of herbal composites for mosquito repellent finishes in bamboo and modal fabrics. Int. J. Res. Ayurveda Pharm. 2018;9:197–200. doi:10.7897/2277-4343.09392
- Rodrigues SN, Fernandes I, Martins IM, et al. Microencapsulation of limonene for textile application. Ind Eng Chem Res 2008;47:4142–4147. doi:10.1021/ie800090c
- Ruiz GM, Lesmes PM, Garcia ML, et al. Design of biocompatible surface-modified polyurethane and polyurea nanoparticles. Polymer. 2012;53:6072–6080. doi:10.1016/j.polymer.2012.10.039
- Karagönlü S, Başal G, Özyıldız F, et al. Preparation of antimicrobial loaded microcapsules for textile applications. Int J Eng Technol. 2018;4:20.
- Lim P, Setthayanond J. Factors affecting release of microencapsulated essential oils from finished silk fabric for automotive and home textile products. IJEAT. 2019;8:2249–8958.
- Maciulyte S, Mamaviciute I, Straksys A. New poly(urethane-urea) microcapsules from PVA modified with APTES: preparation, characterization and enzyme encapsulation. Polym Bull. 2021;78:1867–1886. doi:10.1007/s00289-020-03189-1
- Yılmaz H, Enginar H, Çifci C. Microencapsulation of lambda-cyhalothrin with polyurethane-urea and application on peppermint plant leaves containing a two-spotted red spider mite (tetranychus urticae). J Taibah Univ Sci. 2021;15:63–70. doi:10.1080/16583655.2021.1878671
- Mertgenç C, Enginar H, Yılmaz H. Microencapsulation of fragrance with polyurethane—urea and application on different fabrics. Iran J Sci Technol Trans Sci. 2021;45:1677–1687. doi:10.1007/s40995-021-01135-y
- Yılmaz H, Enginar H, Çifci C. Microencapsulation of pendimethalin with polyurethane-urea and determination of its stability. J Taibah Univ Sci. 2021;15:685–694. doi:10.1080/16583655.2021.1985861
- Torini L, Argillier JF, Zydowicz N. Interfacial polycondensation encapsulation in miniemulsion. Macromol. 2005;38:3225–3236. doi:10.1021/ma047808e
- Aracil B, Bou-Belda E, Monllor P, et al. Binder effectiveness of microcapsules applied onto cotton fabrics during laundry. J Text Inst Proc. 2016;107:300–306. doi:10.1080/00405000.2015.1029808
- Li S, Lewis JE, Stewart NM, et al. Effect of finishing methods on washing durability of microencapsulated aroma finishing. J Text Inst. 2008;99:177–183. doi:10.1080/00405000701489701
- Tözüm MS, Alay Aksoy S. Investigation of tactile comfort properties of the fabrics treated with microcapsules containing phase change materials (PCMs microcapsules). J Text Inst. 2016;107:1203–1212. doi:10.1080/00405000.2015.1099374