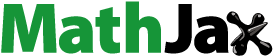
Abstract
This study aims to evaluate the radiotherapy planning performance considering the clinical results of glioblastoma multiforme (GBM)-treated patients from both physical and radiobiological perspectives. Radiotherapy plans are done for 20 patients with GBM tumors who have received radical VMAT treatment on Eclipse-13.5. Dosimetric and radiobiological evaluation metrics are estimated to compare the two plans in terms of TCP, NTCP, and P+ in the context of the evaluated physical metrics. Both conventional and hypofractionation give better dose homogeneity and conformity to the tumour. In addition, hypofractionation schedule coverage targets cells with radiation doses higher than conventional schemes. However, both schedules are at acceptable and safe levels. Statistically, the calculated metrics showed good significance between conventional and hypofractionated radiotherapy schedules. The results have led to the safe use of hypofractionated radiotherapy that can be extended for different tumours, which helps in breaking down the waiting list of patients in many hospitals, especially in cases of pandemics such as COVID-19.
1. Introduction
Fractionated radiotherapy (FRT) is defined as transferring an overall radiation dose to a target volume (TV) in a sequence of smaller radiation doses per fraction. Conventional (standard) fractionation indicates treating with only one fraction per day (5 days per week) at a dose of 1.8–2 Gy per fraction, usually given within 6–7 weeks. Standard fractions are commonly used in most of cancer types.
The reason for choosing (2 Gy per fraction) was experientially agreed upon in the early twentieth century, as evidenced by rapid skin reactions, control rates in squamous cells (tumours) of head and neck cancer, and the uterine cervix [Citation1], in addition to the concept of normal cells recovering from the radiation effects.
Hypofractionation RT delivers a higher dose (>2 to <5 Gy) per fraction, and its regimens have long been used for radical and palliative schedules; commonly, patients who cannot be treated with a conventional fractionated schedule can be treated with a hypofractionated schedule [Citation2]. Hypofractionation decreases the number of fractions delivered by increasing daily treatment doses gives a dose greater than (2 Gy) per fraction. Breast cancer, prostate, lungs, brain, head and neck can be treated with it.
Hypofractionated radiotherapy compared to conventional fractions in some tumour types can reduce RT wait times, machine workloads, patient visits to radiation departments, and medical expenses [Citation3].
The importance of FRT has been known according to an efficient consideration of the repair procedures in malignant and normal tissues based on the time factor. The enhancement of advanced RT is largely dependent on treatment radiation responses. The essential aim of RT treatment is to determine the best dose to destroy tumour cells while reducing dose delivery to organs at risk (OARs) [Citation4].
Oncologists and physicists are responsible for estimating treatment plans and selecting the best one, as well as detecting the possibility of radiation damage. These evaluations commonly depend on reports, information, and the experience of physicists. Radiotherapy plan valuation may be performed by determining physical parameters obtained from dose-volume histograms (DVH). Despite the limitations of the DVH data, treatment plans can be evaluated and the best ones chosen based on the DVH results, and clinical outcomes (effects) of treatment plans can be estimated using physical and radiobiological models [Citation5].
The quantification of the physical and radiobiological impacts caused by radiation absorption is important in advanced radiotherapy. Physically, there are numerous metrics that represent the dose distribution, homogeneity, and conformity within the treatment planning volume (PTV), such as the homogeneity index (HI), target coverage index (TCI), prescription isodose to target volume (PITV) ratio, conformity index (CI), conformity number (CN), gradient index (GI), gradient measure (GM), critical organ scoring index (COSI), modified critical organ scoring index (MCOSI), and quality factor (QF).
Three important metrics to evaluate the radiobiological impact of radiotherapy are cancer local control, the response of normal tissue after exposure to the radiation, and complication-free tumour control probability. Malignant local control is described as “tumour control probability” (TCP), while normal tissue response is defined as “normal tissue complication probability” (NTCP), and complication-free tumour control probability is represented as (P+). As TCP and NTCP are functions of dose, sigmoid curves appear in dose–response curves. To represent the exposure dose response of tumour cells (the target) and normal tissues to irradiation, in addition to their influence by the fractionation scheme, a radiobiological model is developed. For a given dose distribution, TCP and NTCP can be calculated using mathematical models. Finally, radiobiological models must be used in the treatment planning optimization procedure [Citation6].
Radiobiologically, hypofractionation may be beneficial, especially for tumours with low α/β ratios, where rising fraction size causes a proportional excess in the target dose. Furthermore, the reduced treatment period gives flexibility in scheduling different treatment modalities [Citation7]. According to the linear quadratic model, higher radiation doses per treatment fraction can result in a developed therapeutic index ratio (TI) while sparing OARs. Glioblastoma (GBM) is the most dangerous of the brain tumours and the most frequent in adults. It is distinguished by its extremely aggressive behaviour, such that even after surgical resection, followed by radiation treatment and chemotherapy, the average survival time is only one year [Citation8].
Iocolano et al. [Citation9] treated 5378 patients with conventional fractionated RT of 66 Gy in 2 Gy fractions versus 58.5 Gy in 2.5 Gy fractions for hypofractionated RT with non-small cell lung cancer (NSCLC). They treated the patients without chemotherapy and concluded that the patients with locally advanced NSCLC who are not suitable for chemotherapy or surgical resection may have hypofractionated RT.
Wang et al. [Citation10] studied 810 breast cancer patients and compared two types of fractionation, conventional and hypofractionated RT. 409 are treated with conventional fractionated RT (50 Gy in 25 fractions over 5 weeks), while 401 are treated with hypofractionated RT (43.5 Gy in 15 fractions over 3 weeks). They found that hypofractionated RT is safer and has less early skin toxicity than conventional RT.
Lei et al. [Citation11] treated 3871 post-mastectomy breast cancer patients. They were treated with hypofractionated RT versus conventional fractionated RT. Their results show that hypofractionated radiotherapy is not significantly different with respect to efficacy or toxicity in post-mastectomy breast cancer. Furthermore, large randomized clinical trials are required to confirm this conclusion.
Akl et al. [Citation12] treated 100 breast cancer patients with radiation therapy. They were divided into two groups. The first group contained 50 patients treated with hypofractionated RT (40 Gy in 15 fractions), and the other group also had 50 patients treated with conventionally fractionated RT (50 Gy in 25 fractions). This fractionation schedule appears to have obtained hypofractionated RT with similar survival and toxicity to conventionally fractionated RT.
Reddy et al. [Citation13] explained that for GBM cancer patients, hypofractionated IMRT to 60 Gy with 6 Gy in fractions over two weeks with chemotherapy TMZ show to be safe. The performance of this treatment regimen in this small Phase II trial appeared similar to that with the current standard of care therapy. Moreover, studies are certainly needed to determine whether the radiation effect using such a hypofractionated regimen improves local tumour control and overall survival.
This study presents a dosimetric and radiobiological evaluation of hypofractionated rapid-arc treatment plans for glioblastoma multiforme (GBM) brain tumours compared with the conventional treatment plan. Radiation treatment planning plays an important role in modern radiation therapy; it could achieve the radiobiological and dosimetric aspects of the therapy using radiation inverse planning and optimization. Choosing the optimum legitimate plan is a highly important process. To select the optimum plan, a detailed evaluation of the dose-volume histogram (DVH) and isodose distribution is needed by an experienced and attentive oncologist [Citation14]. The ideal solution for the comparison of different plans is the formation of an index that combines all the data and presents it in a simple and quantitative manner [Citation15].
2. Material and methods
For any dosimetric plan, the DVH data supplied by treatment planning systems are used to provide estimates on target coverage as well as on sparing organs at risk and the remaining healthy tissue. In this study, we used identified CT data sets from 20 patients with GBM brain cancer that had been treated previously in the Department of Oncology, Radiotherapy, and Nuclear Medicine, Faculty of Medicine, Ain Shams University, Egypt, with VMAT using a conventional schedule of 60 Gy in 30 fractions, 2 Gy per fraction. The VMAT treatment plans of those patients have been recalculated using a hypofractionated schedule with 40 Gy in 15 fractions, 2.68 Gy per fraction. In all applied plans, an Anisotropic Analytical Algorithm (AAA) was used for dose calculation in the Eclipse Software Treatment Planning System Version 13.5.
The aim of this study is to compare quantitatively the two applicable radiation therapy schedules (conventional and hypofractionated) using various radiobiological evaluation factors (TCP, NTCP, P+, and TI) in the context of the evaluated physical metrics. The treatment planning for each patient is performed twice, once for each schedule (conventional and hypo fraction). The beam geometry for the two plans is similar, as illustrated in the case shown in Figure . Despite the similarity of the beam geometry between the two plans, the dose distributions change because of the variations in the applied dose constraints in each plan because of the various dose schedules. Due to the variability in target position and the nearby essential organs, the beam geometry varied for various patients. The data for the dose-volume coverage are taken from the DVH, which is usually taken from the planning. However, the DVH fails to provide more information, like the location of hot and cold spots. The hypofractionated schedule was evaluated because we expected to obtain promising results, especially in the case of a large patient waiting list, to save time and reduce the number of visits to the hospital, especially during pandemic conditions to avoid infections such as COVID-19.
Figure 1. Horizontal and Sagittal planning sections for both (a) conventional (b) hypofractionation.

2.1. RT-Plan physical evaluation
The RT planning schedules in this study are made by using VMAT technique, and we applied the dose-volume constraints based on Quantitative Analyses of Normal Tissue Effects in the Clinic (QUANTIC) and Radiation Therapy Oncology Group (RTOG) [Citation16]. In hypofractionated treatment plan, the applied constrains were calculated using biological equivalent dose (BED) model to be equivalent to the original constrains which have been used in the conventional fractionated plan as shown in Table . Dosimetric evaluation metrics used to compare the two schedules are: Homogeneity Index (HI), Conformity Index (CI), critical organ scoring index (COSI), modified critical organ scoring index (MCOSI) and quality factor (QF) [Citation17,Citation18].
Table 1. OARs dose constraints.
Nearly all the dosimetric indices that are routinely used for RT plan evaluation were utilized to evaluate the treatment plans in order to provide accurate and safer treatment, as represented and calculated previously and Overall comparison of the Physical evaluation metrics between conventional and hypofractionated schedules for 20 patients with GBM tumour [Citation18].
2.1.1. Homogeneity index (HI)
HI is used to evaluate uniformity (homogeneity) of dose inside the planning target volume (PTV) and is calculated from the equation
(1)
(1) where Dmax is expressed in terms D1, and Dmin is expressed in terms of D99. This formula of HI is the most sensitive to the dose homogeneity difference. The lower (near to one) the index, the better the dose homogeneity of the PTV [Citation19].
2.1.2. Conformity index (CI)
CI is generally used to indicate the portion of a prescription dose that is delivered inside the PTV. The CI is defined as the ratio of the total volume receiving at least the prescription dose, Vp, to the target volume receiving at least the prescription dose, Vtp [Citation20,Citation21].
(2)
(2)
2.1.3. Critical Organ Scoring Index (COSI)
COSI is a measure of both target coverage and critical organ overdose. It can be expressed as
(3)
(3) where
is the fractional volume of ith OAR receiving more than tolerance dose (tol) as listed in Table , and the relative weight (Wi) of fractional volume of each organ is
[Citation22].
2.1.4. Modified critical scoring organ index (MCOSI)
It is defined by the formula
(4)
(4) However the COSI index concentrate only on OARs that receive high-dose region volumes, the modified COSI takes into account both organs that receive high-dose and low-dose regions [Citation22].
2.1.5. Quality factor (QF)
QF is an index that evaluates the quality of the full plan, and expressed as
(5)
(5) where Xi demonstrates all the PTV indices used for evaluating the plan, containing the HI, TCI, PITV, CI, CN, GI, GM, COSI, and MCOSI. The weighting factor (Wi) takes the values between 0 and 1 for all relatively weighted indices (N). Generally, the QF is evaluated to compare the conformity of plans throughout various trials of a treatment [Citation23].
In addition to the above estimated physical metrics, the overall comparison of both physical evaluations for conventional and hypofractionated schedules are represented in Figure . It represents the physical metrics as: homogeneity index (HI), target coverage index (TCI), prescription isodose to target volume (PITV) ratio, conformity index (CI), conformity number (CN), gradient index (GI), gradient measure (GM), critical organ scoring index (COSI), modified critical organ scoring index (MCOSI) and quality factor (QF).
2.2. RT plan radiobiological evaluation
The radiobiological parameters that are regularly applied for RT plan estimation was utilized to evaluate the treatment plans in order to deliver accurate and safer treatment. Radiobiological evaluation metrics used to compare the two schedules are: Tumour Control Probability (TCP), Normal Tissue Complication Probability (NTCP), Complication-Free Tumour Control Probability (P+) and Therapeutic Index (TI).
2.2.1. Tumour control probability (TCP)
TCP is proportional to dose but also inversely related to the number of malignant cells, or target volume. Tumour parameters influencing the TCP are the radiosensitivty of tumour cells, the tumour's location in the human body and its size, and its oxygen sensitivity. Fractionation schedules, radiation intensity (high or low LET), dose rate, the need for radio sensitizers, the addition of radiotherapy with surgery and/or chemotherapy, and treatment technique (such as brachytherapy, conformal RT, IMRT, VMAT, and IGRT) are all treatment-related parameters that influence the TCP. The logistic TCP model is the most often used models for computing TCP. The logistic TCP model is defined as
(6)
(6) where TCD50 represents the tumour dose to likelihood control 50% of the tumours, γ50 is a unit-less parameter that is unique to the normal structure or cancer cells and terms the slope of the dose response curve and EUD is equivalent uniform dose and can be expressed as
(7)
(7) where a is a unit-less model factor that is definite for the normal structure or tumour, and vi is a unit-less that represents the ith part volume getting dose Di in Gy. As the related volume of the full structure relates to 1, the totality of all part volumes vi is equal 1. The EUD reflects the uniform dose for tumours, which has the same likelihood of local control as the real non-uniform dose distribution. Local tumour control is usually determined by the volume that corresponds to the minimum dose. As a result, the EUD for tumour cells will be near the minimum dose, and parameter a should have a high negative value [Citation24,Citation25].
2.2.2. Normal tissue complication probability
NTCP like TCP is a commonly used parameter that represents the complexity of a normal organ. Like TCP, the NTCP curve has a sigmoid shape too. There major NTCP model is the logistic model, which is expressed as
(8)
(8) The TD50 represents the tolerance dose for a 50% complication in normal organs. Destroying one of the links in the serial organ arrangement, such as the spinal cord, will destroy the overall organ function. If the critical organ (serial) receives a higher dose than its tolerance dose, it is more likely to break the chain and miss all its functions. Consequently, for tissues with a serial (critical) structure, the EUD will be near the maximal dose and parameter a will generally is a positive value [Citation26].
2.2.3. Complication-free tumour control probability
Another concept that associates TCP and NTCP and is often used to determine treatment plan quality is complication-free tumour control probability, or tumour control without normal tissue complications (P+). It provides a single value for a treatment regimen that accounts for both expected tumour control and normal tissue effects. It usually takes the form [Citation27].
(9)
(9)
2.2.4 Therapeutic index
A perfect treatment plan must have a 100% probability of healing the cancer and a 0% probability of generating normal tissue problems, which will never ever happen. However, the dose required to shrink the tumour may cause serious toxicity to normal tissues, since the tumour may not get a suitable dose if the normal organs need protection. For different doses, the therapeutic index (TI) indicates how the normal tissue complication probability (NTCP) corresponds to the tumour control probability (TCP). The primary goals of radiotherapy are TCP and NTCP, for a good RT treatment plan, TCP ≥ 50% and NTCP < 5%. Therefore, the treatment outcome is to transfer the TCP curve to the left and the NTCP curve to the right [Citation28].
(10)
(10) TCP, NTCP, P+ and TI are calculated by applying the relevant logistic models using the information of the Eclipse software. The Eclipse system allows the users to calculated the values of the TCP and NTCP radiobiological indices taking into account some parameters such as (TD50, a, γ50 and α/β) which are presented in Table according to the references [Citation29–31].
Table 2. Parameters of TCP and NTCP functions that applied in this study for PTV and different tissues.
3. Results and discussion
In this study, 20 patients with GBM tumours have been selected and treated with the Rapid-Arc technique. These patients had been treated previously in the Department of Oncology, Radiotherapy, and Nuclear Medicine, Faculty of Medicine, Ain Shams University, Egypt, using Rapid-Arc with conventional fractionation. In the new plan, we have used the same technique with the same field geometry but with a different fractionation schedule and different dose constraints. In the hypofractionated treatment plan, the applied constraints were calculated using the biological equivalent dose (BED) model to be equivalent to the original constraints that have been used in the conventional fractionated plan.
The new fractionation schedule is hypofractionation, and the two plans are compared. Although the same field geometry has been applied in the two plans, the dose distributions are different because of the differences in the dose constraints. The applied dose constraints in the first treatment plan (conventional schedule) had been taken according to RTOG and QUANTIC [Citation16]. However, in the new plan with the hypofractionation schedule, the dose constraints are changed (Table ) because of the total dose change in the dose fractionation. Accordingly, the dose distribution and DVH are different in the two plans, and because of the differences in the dose schedules used to perform the dosimetric and radiobiological calculations.
We have started with evaluating HI, which is calculated as shown in Figure . As the HI value is close to one, it refers to better dose homogeneity of the PTV. It can be easily seen that HI for most of the patients in both schedules is almost the same; this implies that the dose constraints and the dose fractionation did not affect the dose homogeneity in the PTV. In order to compare the PTV dose conformity and coverage in the two plans, CI is calculated and represented in Figure .
The values of CI are closer to one; they denote well-targeted conformity in the treatment scheme. But practically, the values of CI are always greater than one. As represented in Figure , the values of CI are always greater than unity in both conventional and hypofractionated schedules for all patients in this study. In other words, the above-calculated indices show good PTV coverage and dose conformity in the two plan schedules.
To measure both target coverage and critical organ overdose, COSI and MCOSI are calculated and represented in Figures and , respectively, for both conventional and hypofractionated schedules. To assure planning quality and effectiveness, planning quality factors (QF) are estimated and plotted in Figure . The overall comparison between the conventional and hypofractionation schedules is averaged over the 20 patients, represented in Figure , and showed very good results. The results are in very good agreement with the acceptable dosimetric calculations [Citation32–34]. The significance of the differences between these indices in conventional and hypofractionation is determined and gives a good statistical correlation.
Figure 7. Overall comparison of the Physical evaluation metrics between conventional and hypofractionated schedules for 20 patients with GBM tumour.

In the radiobiological evaluation of different treatment plans, we have used (TCP, NTCP, P+, and, TI) in this study, we have started with evaluating the TCP, and its EUD values for both treatment schedules are calculated by MATLAB and illustrated in Table and Figure , respectively.
Figure 8. EUD of TCP for 20 patients with GBM tumour for conventional and hypofractionated planning.

Table 3. TCP and NTCP of 20 GBM patients for conventional and hypofractionated schedules.
We found that TCP values for a hypofractionated schedule give better dose distribution and target coverage. In other words, the above-calculated indices show good coverage and dose distribution in two plan schedules with a significant difference in p-value (0.05). As the TCP value is ≥50%, it refers to better target coverage and dose distribution. EUD of TCP represents the minimum dose coverage of the tumour, and it is clear that EUD for most of the patients in both schedules are almost at the same level. This means that the dose per fraction did not affect the dose distribution in the PTV. Statistically, it shows a significant difference with p-value = 0.05. This is because of the similarity in prescribed dose and dose normalization in both plans, and the dose-volume constraints are validated.
The NTCP and its EUD values for OARs (brainstem, optic chiasm, cochlea, eyes, lenses, optic nerves, pituitary gland, and spinal cord) of 20 patients for both schedules are calculated by a MATLAB program and illustrated in Table and Figure , respectively. It is found that the conventional fractionation has the highest NTCP value, while the hypofractionated schedule gives the lowest NTCP values, close to zero. However, both schedules are still in the safe and acceptable range. As the NTCP value is <5%, it refers to better sparing and avoiding OARs. In addition, the calculated EUD of NTCP (which corresponds to the maximum dose delivered to the normal organs) represents better values for hypofractionated than conventional fractionation. It is graphed in Figure , and it implies good significance with a p-value = 0.01.
P+ has been estimated to help in selecting the best RT plan. We pointed out the P+ value, which integrated both TCP and NTCP, to clarify the difference in radiobiological outcomes of the plans, as shown in Figure . It is found that the P+ values for the two plan schedules are close and in the safe level with a significant difference of p-value = 0.05. As the P+ value is near unity, it refers to a high quality treatment plan. The results are in very good agreement with the acceptable radiobiological calculations [Citation32,Citation35].
TI is calculated from Equation (10), and it is represented in Table . We found that TI gives a very low value (close to zero), which is better because it means that the TCP and NTCP sigmoid curves are shifting to the left and right, respectively [Citation28]. All the previous results are in very good agreement with the acceptable radiobiological calculations [Citation36,Citation37].
Table 4. Therapeutic Index (TI) for conventional and hypofractionated schedules.
The main concern addressed by this study is: what is the best option for GBM tumour RT treatment, conventional or hypofractionation? The comparison is performed by dose-based analysis of the PTV range and critical organs. The physical metrics and radiobiological indices, including (TCP, NTCP, P+, and TI) are calculated and illustrated graphically. The statistical p-values of all the physical and radiobiological parameters are summarized in Table to show the significance between the conventional and hypofractionation plans.
Table 5. p-values for the significance analysis for conventional and hypofractionated schedules.
Almost all the dosimetric and radiobiological planning goals are on the safe side for hypofractionation plans for each of the 20 patients. The main overall benefits of the hypofractionation are (1) reducing the number of treatment sessions, (2) reducing the overall treatment dose, (3) reducing the number of patient visits to the hospital, (4) reducing the spread of epidemics and infections such as COVID-19, and (5) reducing the load on hospitals. In addition, deliver the appropriate dose to the target while optimizing the plan to adhere to the OAR constraints. Moreover, reducing the dose and the fractions according to the hypofractionation schedules is very useful to reduce the probability of metastases in bone, adjacent brain tissues, lung, skin, and lymph nodes.
4. Conclusion
Calculations of HI, CI, COSI, MCOSI, QF, TCP, NTCP, P+, and TI showed a good level of safety of the hypofractionation schedule from a biological point of view. The NTCP function clarifies the difference in biological outcomes between the two plans. The quality of the radiobiological evaluation of the RT treatment plan is determined by calculating P+ rather than TCP and NTCP alone. The obtained results of this study have shown that a hypofractionated RT plan can be applied because it saves time and reduces the radiation dose for the studied type of brain tumour (GBM).
Hypofractionated plans are more sparing of OARs than conventional plans in the treatment of GBM patients. The results have led to a significant and safe use of hypofractionated RT that breaks down the waiting list of patients in many hospitals, especially in cases of pandemics such as COVID-19. It is very important to reduce patient presence in hospitals, especially during epidemics, and the exposure of hospital personnel to radiation should be limited. The obtained results of this study have shown that the hypofractionated RT plan is better than the conventional plan because it saves time and reduces the radiation dose for the studied type of brain GBM tumour. The future work will consider other different tumours to apply the hypofractionated RT plan, calculating the physical and radiobiological evaluations for an effective and safe treatment.
Disclosure statement
No potential conflict of interest was reported by the author(s).
References
- Joiner MC, van der Kogel AJ. Basic clinical radiobiology. CRC Press; 2018.
- Maringe C, Spicer J, Morris M, et al. The impact of the COVID-19 pandemic on cancer deaths due to delays in diagnosis in England, UK: a national, population-based, modelling study. Lancet Oncol. 2020;21(8):1023–1034. doi:10.1016/S1470-2045(20)30388-0
- Hashemi FA, Barzegartahamtan M, Mohammadpour RA, et al. Comparison of conventional and hypofractionated radiotherapy in breast cancer patients in terms of 5-year survival, locoregional recurrence, late skin complications and cosmetic results. APJCP. 2016;17(11):4819.
- Ågren A, Brahme A, Turesson I. Optimization of uncomplicated control for head and neck tumors. Int J Radiat Oncol Biol Phys. 1990;19(4):1077–1085. doi:10.1016/0360-3016(90)90037-K
- Chaikh A, Docquière N, Bondiau P-Y, et al. Impact of dose calculation models on radiotherapy outcomes and quality adjusted life years for lung cancer treatment: do we need to measure radiotherapy outcomes to tune the radiobiological parameters of a normal tissue complication probability model? Transl Lung Cancer Res. 2016;5(6):673. doi:10.21037/tlcr.2016.11.04
- Chargari C, Magne N, Guy J-B, et al. Optimize and refine therapeutic index in radiation therapy: overview of a century. Cancer Treat Rev. 2016;45:58–67. doi:10.1016/j.ctrv.2016.03.001
- Brand D, Kirby AM, Yarnold JR, et al. How low can you go? The radiobiology of hypofractionation. Clin Oncol. 2022;34(5):280–287. doi:10.1016/j.clon.2022.02.009
- Holland EC. Glioblastoma multiforme: the terminator. Proc Natl Acad Sci USA. 2000;97(12):6242–6244. doi:10.1073/pnas.97.12.6242
- Iocolano M, Wild AT, Hannum M, et al. Hypofractionated vs. conventional radiation therapy for stage III non-small cell lung cancer treated without chemotherapy. Acta Oncol. 2020;59(2):164–170. doi:10.1080/0284186X.2019.1675907
- Wang S-L, Fang H, Song Y-W, et al. Hypofractionated versus conventional fractionated postmastectomy radiotherapy for patients with high-risk breast cancer: a randomised, non-inferiority, open-label, phase 3 trial. Lancet Oncol. 2019;20(3):352–360. doi:10.1016/S1470-2045(18)30813-1
- Liu L, Yang Y, Gao Q, et al. Comparing hypofractionated to conventional fractionated radiotherapy in postmastectomy breast cancer: a meta-analysis and systematic review. Radiat Oncol. 2020;15(1):17. doi:10.1186/s13014-019-1449-z
- Powell AC, Rogstad TL, Jacob NM, et al. The association between use of hypofractionation and treatment completion among recipients of radiation therapy post-mastectomy. Pract Radiat Oncol. 2020;10(4):e244–e249. doi:10.1016/j.prro.2019.10.009
- Reddy K, Damek D, Gaspar LE, et al. Phase II trial of hypofractionated IMRT with temozolomide for patients with newly diagnosed glioblastoma multiforme. Int J Radiat Oncol Biol Phys. 2012;84(3):655–660. doi:10.1016/j.ijrobp.2012.01.035
- Alfonso L, Herrero JMA, Nunez L. A dose-volume histogram based decision-support system for dosimetric comparison of radiotherapy treatment plans. Radiat Oncol. 2015;10(1):1–9. doi:10.1186/s13014-015-0569-3
- Feuvret L, Noël G, Mazeron J-J, et al. Conformity index: a review. Int J Radiat Oncol Biol Phys. 2006;64(2):333–342. doi:10.1016/j.ijrobp.2005.09.028
- Oermann E, Collins BT, Erickson KT, et al. Cyberknife® enhanced conventionally fractionated chemoradiation for high grade glioma in close proximity to critical structures. J Hematol Oncol. 2010;3(1):1–9. doi:10.1186/1756-8722-3-22
- Deasy JO, Blanco AI, Clark VH. CERR: a computational environment for radiotherapy research. Med Phys. 2003;30(5):979–985. doi:10.1118/1.1568978
- Emami B. Tolerance of normal tissue to therapeutic radiation. Rep Radiother Oncol. 2013;1(1):123–127. https://applications.emro.who.int/imemrf/Rep_Radiother_Oncol/Rep_Radiother_Oncol_2013_1_1_35_48.pdf
- Yoon M, Park SY, Shin D, et al. A new homogeneity index based on statistical analysis of the dose–volume histogram. J Appl Clin Med Phys. 2007;8(2):9–17. doi:10.1120/jacmp.v8i2.2390
- Dhabaan A, Elder E, Schreibmann E, et al. Dosimetric performance of the new high-definition multileaf collimator for intracranial stereotactic radiosurgery. J Appl Clin Med Phys. 2010;11(3):197–211. doi:10.1120/jacmp.v11i3.3040
- Knöös T, Kristensen I, Nilsson P. Volumetric and dosimetric evaluation of radiation treatment plans: radiation conformity index. Int J Radiat Oncol Biol Phys. 1998;42(5):1169–1176. doi:10.1016/S0360-3016(98)00239-9
- Menhel J, Levin D, Alezra D, et al. Assessing the quality of conformal treatment planning: a new tool for quantitative comparison. Phys Med Biol. 2006;51(20):5363. doi:10.1088/0031-9155/51/20/019
- Pyakuryal A, Myint WK, Gopalakrishnan M, et al. A computational tool for the efficient analysis of dose-volume histograms for radiation therapy treatment plans. J Appl Clin Med Phys. 2010;11(1):137–157. doi:10.1120/jacmp.v11i1.3013
- Niemierko A. Reporting and analyzing dose distributions: a concept of equivalent uniform dose. Med Phys. 1997;24(1):103–110. doi:10.1118/1.598063
- Okunieff P, Morgan D, Niemierko A, et al. Radiation dose-response of human tumors. Int J Radiat Oncol Biol Phys. 1995;32(4):1227–1237. doi:10.1016/0360-3016(94)00475-Z
- Gay HA, Niemierko A. A free program for calculating EUD-based NTCP and TCP in external beam radiotherapy. Phys Med. 2007;23(3-4):115–125. doi:10.1016/j.ejmp.2007.07.001
- Kallman P, Lind B, Brahme A. An algorithm for maximizing the probability of complication-free tumour control in radiation therapy. Phys Med Biol. 1992;37(4):871–890. doi:10.1088/0031-9155/37/4/004
- Mallick S, Rath GK, Benson R. Practical radiation oncology. Springer; 2019.
- Zeman EM, Schreiber EC, Tepper JE. Basics of radiation therapy. In: Abeloff's clinical oncology. Elsevier; 2020. p. 431–460.e3. https://www.sciencedirect.com/science/article/abs/pii/B978032347674400027X
- Burman C, Kutcher GJ, Emami B, et al. Fitting of normal tissue tolerance data to an analytic function. Int J Radiat Oncol Biol Phys. 1991;21(1):123–135. doi:10.1016/0360-3016(91)90172-Z
- Banisharif S, Shahbazi-Gahrouei D, Akhavan A, et al. Determining the optimum tumor control probability model in radiotherapy of glioblastoma multiforme using magnetic resonance imaging data pre-and post-radiation therapy. J Res Med Sci. 2022;27:10. doi:10.4103/jrms.JRMS_1138_20
- Amin A, El Sayed S, Ezzat A. Dosimetric evaluation of advanced radiotherapy techniques in treating patients with prostatic cancer. Res Oncol. 2017;13(2):40–45. doi:10.21608/resoncol.2017.1654.1036
- Cao T, Dai Z, Ding Z, et al. Analysis of different evaluation indexes for prostate stereotactic body radiation therapy plans: conformity index, homogeneity index and gradient index. Precis Radiat Oncol. 2019;3(3):72–79. doi:10.1002/pro6.1072
- Inal A, Duman E, Ozkan EE. Evaluating different radiotherapy treatment plans, in terms of critical organ scoring index, conformity index, tumor control probability, and normal tissue complication probability calculations in early glottic larynx carcinoma. J Cancer Res Ther. 2020;16(3):485–493. doi:10.4103/jcrt.JCRT_888_18
- MacDonald SM, Ahmad S, Kachris S, et al. Intensity modulated radiation therapy versus three-dimensional conformal radiation therapy for the treatment of high grade glioma: a dosimetric comparison. J Appl Clin Med Phys. 2007;8(2):47–60. doi:10.1120/jacmp.v8i2.2423
- Patel GR, Mandal A, Mishra R, et al. Role of overall treatment time when estimating TCP & NTCP of head & neck radiotherapy treatment plans in altered fractionation. Iran J Med Phys. 2022.
- Spohn SK, Sachpazidis I, Wiehle R, et al. Influence of urethra sparing on tumor control probability and normal tissue complication probability in focal dose escalated hypofractionated radiotherapy: a planning study based on histopathology reference. Front Oncol. 2021;11:652678. doi:10.3389/fonc.2021.652678