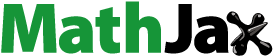
Abstract
Perovskite oxides act as an efficient electrocatalyst, but their limited active surface area has made it challenging to enhance their electrocatalytic activity. Thus, researchers found that changing the crystalline surface to an amorphous surface having oxygen vacancy can create an enriched active zone. In this research, we adopt a top-down approach for the amorphization of the crystalline CeCoO3 nanostructure that creates crystal defects, producing materials with a higher specific surface area, potential electrocatalysis for oxygen evolution reaction (OER) and greater stability. The calculated overpotential (η) and Tafel slope for defective CoCO3 (D-CCO3) is 265 and 35.95 mV dec−1 very low as compared to the crystalline CoCO3 (C-CCO3, 384 and 76.11 mV dec−1). The electrochemical analysis also suggests that the defective CoCO3 (D-CCO) exhibited the 33.96 mF and ECSA of 849 cm2. The current research enables a valuable approach for improving and changing the material properties and electrochemical efficiency of nanoscale perovskite oxide electrocatalysts attributed to crystal defects and nitrogen doping. However, further modifications to the D-CCO structure in the near future may be employed to address other environmental challenges.
1. Introduction
Our diminishing fossil fuel supply and the consequent environmental impact need the development of sustainable technology and renewable resources of energy [Citation1–4]. Renewable resources of energy, like wind power, can be used in conjunction with electrically powered electrocatalysis to create environmentally friendly fuels and easily accessible chemicals [Citation5–9]. An example of a clean, renewable energy source is the electrocatalytic water-splitting process, which produces hydrogen through the half-reactions involving oxygen evolution as well as hydrogen evolution [Citation10–13]. Since oxygen evolution reaction (OER) has a high activation barrier, multiple electrons along with proton transfers and slack reaction kinetics, it is one of these processes and the limiting factor in the efficiency of related energy devices [Citation14–17]. Scientists have put a lot of time and effort over the past decade, looking for more efficient and long-lasting OER electrocatalysts [Citation18–23]. Examples include transition-metal complexes, nanomaterials based on IrO2 or RuO2, atomically dispersed metals and others [Citation24–26].
To get active, stable and cost-effective results in OER, researchers are looking into perovskite oxides with the general formula ABO3, in which A along with B sites have been filled with lanthanide metals (or alkaline-earth metals) alongside transition metals, as possible replacements regarding nanocatalysts made of precious metals [Citation27–30]. Perovskite catalysts have several advantageous properties, but an essential one is those materials at the A or B sites have a high propensity to be heavily leached away in an electrochemical process [Citation31–34]. In addition, an amorphous layer on the catalyst’s surface is possible to be formed, surface reconstruction and chemical development in the case of BaIrO3 [Citation35], LaCo0.8Fe0.2O3 [Citation36], LaNiO3 thin film [Citation35], and La0.8 Sr0.2MnO3 [Citation37] double perovskites, among others [Citation38]. The electrochemical water oxidation performance of perovskites is enhanced via a passive amorphous rebuilding process, but the convolution of the whole reconstruction mechanism is hindered by an unidentified reaction process along with inadequate stability concerning OER [Citation39–41]. However, crystalline materials have a well-defined periodic arrangement of atoms, which creates a rigid structure. This regular arrangement of atoms can enhance the electrical conductivity of the substance by helping the movement of electrons through the crystal lattice. Additionally, the well-defined structure of crystalline materials can provide sites for electrochemical reactions to occur, such as the adsorption or desorption of ions, which can affect the material’s capacitance and electrochemical reactivity. On the other hand, defective products lack the long-range arrangement of crystalline substances and have a disordered atomic arrangement. The lack of regularity in the atomic structure can lead to lower electrical conductivity in amorphous materials compared to their crystalline counterparts. However, the disordered structure of amorphous materials can also provide an enriched active zone for the electrochemical process to occur, which can enhance their electrochemical reactivity [Citation42]. One way to address these challenges is to keep a close eye on the atomic-level chemical dissolution and surface reconstructive change that occur during electrocatalytic activities. Double perovskite nanopowders had distinctive characteristics that enabled during OER, the surface of a material can rearrange itself dynamically, therefore generating a self-assembled metal hydroxide active layer. The interfacial transition of SrIrO3 was explored by Suntivich et al. They discovered that crystalline SrIrO3’s surface became coated in amorphous SryIrOx composed of Ir4+O6 octahedra and Sr2 +‐Ir3+ residue after an electrochemical OER operation. Amorphization concerning SrIrO3 resulted from lattice oxygen activation, step-coupled Sr2+ and O2- diffusion at (oxide-electrolyte) interface. Zhiwang Li et al. developed the crystalline CeCoO3 via a hydrothermal approach and changed it into the defective CeCoO3 via the reduction of urea. The scientist explored that the defective material exhibited lower overpotential as well as a small Tafel slope in comparison to crystalline CeCoO3 [Citation43].
A second possibility is that an amorphous perovskite catalyst is made early on in the preparation process, which prevents the occurrence of any unnecessary random rearrangement during electrocatalytic water oxidation that will be responsible for the creation of crystal defects [Citation44–46]. Therefore, crystal defects significantly contributed to the enhancement of OER electrocatalytic reactions [Citation47–49]. Additionally, remarkable is the fact that amorphous nanocatalysts, in contrast to their nanocrystalline counterparts, generally, display unique features that can be linked back to their disorganized atomic structure as well as unsaturated coordination environments [Citation50,Citation51]. The process that involves these strategies for amorphization concerning nanoscale perovskite catalysts has not been widely explored due to the absence of flexible ways for the fabrication of amorphous perovskite nanostructures as well as re-crystallization at higher temperatures. As a result, it is clear that a straightforward technique for manufacturing amorphous nanoscale perovskite catalysts is urgently required [Citation52–54].
Doping is considered as a potent strategy to increase the electrochemical feature of the perovskite substances. There are various types of organic precursor employed as sources of nitrogen precursor. Among the different precursors of nitrogen, urea is considered economical, wide availability, controlled nitrogen release, high nitrogen content and efficient source for doping of nitrogen [Citation55]. The doping of nitrogen provided several advantages such as the creation of new active sites on the interfaces of the electrocatalyst, modify the electronic structure and it can also enhance the selectivity of the materials [Citation56].
Nanoscale crystalline CeCoO3 (COO) perovskite is amorphized evolving, changes into highly disordered condition with improved electrocatalytic OER efficiency. Highly crystalline COO (C-CCO) nanopowders were first produced via a wet chemical approach with higher-temperature calcination for successfully changing the structure of CCO3 perovskite. C-CCO nanopowders and urea were mixed and heated at 400°C for 3 h before proceeding to cool at room temperature, yielding reduced cerium cobaltite powders. Defective CeCoO3 (D-CCO) in other contexts show diffraction peaks that have almost completely disappeared and show a transition through the crystalline phase towards a defective state that occurred during the reduction of urea. The electrochemical outcome shows that the D-CCO contains a smaller overpotential of around 300 mV at 10 mA cm−2 along with a Tafel slope around 57.2 mV dec−1 than C-CCO.
2. Experimental work
2.1. Materials
Cobalt nitrate (Co(NO3)2·6H2O, 99%), citric acid (C6H8O7, 99%), cerium nitrate (Ce(NO3)3.6H2O, 99%), urea (CO(NH2)2, 99%) and ammonia solution (NH3, 30%) are all chemicals of analytical grade supplied by Sigma Aldrich and used in the fabrication of CeCoO3.
2.2. Synthesis of CeCoO3 (CCO)
Cobalt nitrate, citric acid and cerium nitrate were dissolved in DI H2O at molar ratios of 1:4:1 in a beaker, gradually by the incorporation of NH3 with an increase of pH to 7. This solution was stirred for 2 h in order to produce a fluffy precursor. This precursor was used for calcination in an electric furnace at 750°C for 2 h, the temperature being changed at a rate of 5°C per minute to produce pure cerium cobaltite powder. The reaction suspension was then allowed to cool at ambient temperature for obtaining (pristine-CeCoO3). After that a consistent combination of urea (as reducing agent and nitrogen precursor) and CeCoO3-pristine nanopowders (mass ratios of 1:5) was created before being put in a tube furnace. Mixed nanopowders were heated to 500°C at 5°C per minute and this temperature was maintained for 2 h in an N2 environment alongside a flow rate of 40 mL per minute for producing defective cerium cobaltite powders (D-CeCoO3). The schematic illustration for the synthesis of defective D-CCO using the urea reduction method is display in Scheme 1.
2.3. Physical measurements
Characterization of the synthesized crystalline and defective CeCoO3 was analysed by using various techniques that include X-ray diffraction (Bruker D8), Fourier transformed infrared (JASCO 6800), scanning electron microscopy configured with energy-dispersive X-ray spectroscopy (SEM-EDX, NanoNova SEM 200 FEG) and Brunauer Emmett Teller (BET, V3.01 ASAP 2020) for investigation of crystal structure, surface functionality, morphology and textural property of the both pristine and defective CeCoO3.
2.4. Electrochemical measurements
The 1.0 M KOH solution was employed for all electrochemical analyses at room temperature. Based on the electrochemical workstation (CHI760e), a three-electrode system was used, with an Ag/AgCl electrode along with a platinum plate selected as a standard electrode as well as an axillary electrode, respectively. The following was employed to convert the potential into RHE.
(1)
(1)
The samples were activated via cyclic voltammetry (CV) that ranges from 0 to 2 V (V vs. silver/silver chloride) at a scan rate of 5 mV s−1. The linear sweep voltammetry (LSV) curves were produced in the range of 0.1–0.8 V vs. Ag/AgCl at a scan rate of 5 mV s−1. Electrochemically active surface area (ECSA) was investigated by utilizing CV curves in the 0–0.1 V vs. Ag/AgCl range at a scan rate of 10–50 mV s−1 using a sweeping speed of 10–50 mV s−1. Electrochemical impedance spectroscopy (EIS) curves were measured in the frequency range of 0.1–100 kHz at a voltage of 0.55 V vs. Ag/AgCl. Chronopotentiometry analysis was acquired at 10 mA cm−2 for 24 hours. For a comparative analysis, RuO2 was used as a benchmark in all the electrochemical tests under the same experimental condition. However, the working electrode was synthesized with 5 mg of the fabricated electrocatalyst dispersed in the DI-H2O and then sonicated for 10 min to create a homogeneous ink. This electrocatalytic ink is then deposited in the Ni foam (NF) by the drop casting strategy.
3. Results and discussion
3.1. Material analysis
X-ray diffraction (XRD) peak of both pristine and defective CeCoO3 electrocatalyst is depicted in Figure (a). These diffraction curves at 2θ = 28.32°, 32.40°, 47.29°, 55.86° and 59.10° are attributed to CeO2 (111), (200), (220), (311), (222) and those diffraction peaks at 30.85°, 37.17°, 44.76° and 64.94° are corresponding to Co3O4 (220), (311), (222) and (400). However, the existence of both phases in the XRD diffractogram confirms the successful synthesis of the CeCoO3 materials in a single phase and their results are well matched with the reported work [Citation57]. The pristine CeCoO3 XRD diffraction pattern shows the crystalline phases of the electrocatalyst and on the other hand, defective CeCoO3 shows diffraction peaks that have virtually completely disappeared, suggesting that a nearly complete change from the crystalline phase towards an amorphous state responsible for defective sites providing enriched active site during the reduction of urea [Citation58]. Therefore, these data of XRD show the role of urea in supporting the annealing process that proves advantageous for the creation of defective catalysts.
The FT-IR spectrum was used for the investigation of the chemical structure as well as functional groups of materials as illustrated in Figure (b). The stretching vibrations of Co–O and CeO are linked to three distinct bands in the CeCoO3 perovskite’s spectrum that appeared below 1000 cm−1. The large intensity transmittance peak observed at 1650.06 cm−1 is due to the OH- group adsorbed at the surface of the electrocatalyst [Citation59]. However, a large adsorption band appeared at 3300–3600 cm−1 corresponding to the absorption of the OH group and the absence of additional peaks suggests the pureness of the material. While the fabricated material shows the same reflectance pattern but the C-CCO exhibited a little bit narrow band in origin and the broadness in the peak indicating the defective nature of the D-CCO nanostructure. However, the broadness of the peaks was responsible for the lack of long-range orderness and amorphous surface [Citation60].
Figure (a–c) displays the SEM micrograph of the C-CCO and D-CCO at a magnification of 500 nm. Figure (a) displays sharp with a defined boundary of irregular nanoparticles, indicating the crystalline morphology of C-CCO nanoparticles. Besides this, Figure (b) depicted the agglomerated, random, diffused and with no prominent nanoparticles showing the D-CCO morphology of the nanostructures. To evaluate the purity of the D-CCO, we employed the EDX analysis as illustrated in Figure (c). The presence of Ce, Co, N, C and O element in the EDX spectrum indicates the purity and successful fabrication of the D-CCO nanostructure.
Figure 2. SEM micrograph of (a) C-CCO, (b) D-CCO, (c) EDX of D-CCO, (d) BET isotherm and (e) pore size of C-CCO and R-C-CCO.

The surface area analysis results imply that both samples possess a mesoporous structure since they exhibit an IV Type of isotherm along with a hysteresis curve of H4 in P/Po ranging from 0.0 to 1.0. The specific interface area (89 m2 g−1) of the D-CCO nanomaterial is higher than that of C-CCO nanoparticles (55 m2 g−1) due to their large number of active zones as displayed in Figure (d). The porous structure allows electrolyte ions to more efficiently diffuse to the electrolyte ion [Citation61]. Figure (e) displays the pore size distribution for both the D-CCO and C-CCO with a value of 18 and 22 nm and the pore volume of D-CCO and C-CCO are 0.06 and 0.03 cm3 g−1 accordingly. The mesoporous structure of D-CCO and C-CCO has a vast surface area that plays a viable part in increasing electrochemical performance by facilitating shorter diffusion pathways, increased surface area for electrochemical reactions and increased speed of electrolyte transport [Citation62].
X-ray photoelectron spectroscopy (XPS) was utilized to investigate the chemical nature and electronic structure of CeCoO3 and their impact on the adsorption behaviour of active regions in electrocatalytic processes. Figure (a) illustrates that the C-CCO and D-CCO show the elemental makeup of CeCoO3 are Ce, Co, O, N and C. The XPS results revealed that the binding energy of D-CCO is positively shifted due to the reduction of XPS than the C-CCO indicating a significant increase in oxygen vacancies. The presence of a small nitrogen peak in the XPS of D-CCO suggests that some nitrogen was doped into the crystalline structure of CeCoO3 during the reduction process. Figure (b) represented the Co 2p display of the peaks at 780.13 and 795.93 eV for Co 2p3/2 and Co 2p1/2, respectively. The reduced form of CeCoO3 exhibits a positive shift in the binding energies of Co 2p, which can be attributed to the additional Co2+ present, in contrast to the unaltered state of CeCoO3 [Citation63]. The alterations observed in the electronic configuration of Co 2p in CeCoO3-reduce are linked to the presence of defective sites that have been induced by urea reduction. The O 1s shows peaks at 529.83, 530.55 and 532.1 eV ascribing to lattice oxygen, O-H and adsorbed oxygen, respectively, as represented in Figure (c). The band appeared at 531.5 in O 1s spectra of D-CCO indicating the high amount of oxygen vacancy than the C-CCO [Citation64]. The Ce 3d XPS shows peaks at 886.77, 884.15, 883.66 and 881.08 eV that are assigned to Ce4+ 3d5/2, Ce3+ 3d3/2, Ce4+ 3d5/2 & Ce3+ 3d5/2, respectively, as represented in Figure (d). The enhanced surface states observed in CeCoO3-reduce can be attributed to the structural transformation from a crystalline phase to a defective state, as compared to CeCoO3-pristine [Citation65]. In addition, there is a noticeable positive core-level shift of 0.5-1 eV in the Ce 3d binding energy in D-CCO compared to C-CCO. This improvement is indicative of an enhancement in O2 vacancies being created on the interfaces of the D-CCO.
The N 1s XPS spectra displayed in Figure (e) contain two deconvoluted bands that appeared at 398.34 and 400.43 eV for N-Ce and N-H band, respectively. The presence of the enriched O2 in D-CCO is indicative of the large number of oxygen vacancies introduced into the material during the urea reduction process. Here, the N-H-O-Co configuration is formed as NH4+ is liberated during urea pyrolysis displacing Ce3+ and weakly interacts with the CoO6 octahedra. CeCoO3’s amorphous structure is formed as N-H is removed, and any residual NH4+ is eliminated [Citation66]. which has the potential to significantly accelerate the electrochemical OER due to the fact that active oxygen species are an example of electrophilic reagents with high catalytic oxidation activity.
3.2. Electrochemical analysis
Its electrocatalytic property regarding C-CCO and D-CCO electrode material was analysed with the three-electrode system under an alkaline solution. Figure (a) illustrates that D-CCO shows the highest current density along with the prominent faradic behaviour than C-CCO under the same 0–2 V (V vs. RHE). The C-COO shows greater alkaline OER initial potential (onset) at 1.68 V and a steady spike in current density as the applied potential increases, as displayed in Figure (b). Whereas D-COO shows a small onset potential (1.44 V) and acts as efficient electrocatalytic activity for the OER process and only needs ultralow overpotentials around 265 and 384 mV for the attaining current densities of 10 cm−2, accordingly as displayed in Figure (c). D-COO has an amorphous shape, nitrogen doping and a modified electronic structure, which likely account for the observed increase in OER activity. The Tafel slope of the defective D-CCO (35.95 mV dec−1) is less than crystalline C-CCO-pristine (76.11 mV dec−1) indicating the enhanced reaction kinetics concerning OER in the amorphous material as represented in Figure (d). The OER analysis revealed that D-CCO exhibited the highest OER electrocatalytic efficiency than the other C-CCO and Ni foam, it might be due to several factors. (i) Defective materials typically have a large density of active zone on their surface, which enhances the electrocatalytic behaviour of the material towards the OER process. (ii) Defective materials can have a larger surface area than their crystalline counterparts, which can offer more surface area for catalytic reactions to occur and increase the efficiency of the OER process. (iii) The amorphous structure can lead to a more irregular surface morphology, which enhances the interfacial roughness and provide more active regions for the OER process [Citation67]. (iv) Nitrogen doping also increase the OER activity. Overall, defective materials can offer significant advantages over crystalline materials for the OER process, making them an attractive choice for several applications in energy storage and conversion (Table ). Table presented the present article with reported previous work.
Figure 4. (a) CV, (b) LSV, (c) overpotential and (d) Tafel slope of all fabricated materials and Ni foam.

Table 1. Comparative analysis of reported research and fabricated materials.
Additionally, double-layer capacitance (Cdl) experiments were performed for evaluating the ECSA of fabricated electrocatalysts as represented in Figure (a,b). By utilizing the CV procedures, we find that the Cdl of R-COO is 33.96 mF, which is much greater than that of C-CCO (19.86 mF), a higher Cdl value indicating a more active surface and greater amounts of stored electrolyte ions as depicted in Figure (c,d). Remarkably, D-CCO (8496 cm2) had an ECSA that was higher than the C-CCO (4500 cm2) and was calculated using the following equation [Citation74]:
(2)
(2)
Herein, Cdl represents the double layer capacitance as well as Csp represents the specific capacitance regarding conductive substrate (NF, 0.04 mF cm−2). The larger surface area of the fabricated electrode material is then responsible for diverse morphology, small crystallite size, nitrogen doping and remarkable electronic structure.
For further evaluating charge transfer rates concerning electrocatalysts, EIS measurements performed at applied potential 0.55 V in frequency ranging from 100 Hz to 100 kHz as shown in Figure (a). The declined semi-circle indicates charge transfer resistance (Rct) among electrode material as well as electrolyte ion. when OER is activated, its charge transfer rate increases and the Rct value decreases and vice versa. The obtained Nyquist plot shows that the Rct of D-CCO (0.38 Ω) is smaller than that of C-CCO (1.2 Ω). This result suggests that the amorphization of the perovskite nanostructure of R-COO makes fast electron transport among electrodes and electrolyte feasiblity in C-COO [Citation75]. Additionally, there are some other factors that also contribute to reducing the value of Rct. (i) The lack of long-range order in defective materials results in the presence of defects and disorder in the material. These defects can act as catalytic zones and promote the OER reaction, resulting in lower charge transfer resistance [Citation76], (ii) defective materials often have a porous structure that permits effective mass transportation of reactants and products to and from the reaction sites [Citation77]. This can increase the reaction rate and decline the charge transfer resistance. (iii) Defective materials typically have a high surface area, which provides an enriched active area for the OER process to occur and (iv) nitrogen doping also increases the OER activity of D-CCO [Citation78]. Figure (b) depicts that the R-COO shows a straight line over the current density of 96 mA cm−2 at an applied potential of 0.75 V for 50 h. The chronoamperometry result indicates that the material exhibited stable behaviour than that of the C-COO and RuO2 as a benchmark due to existence of a large number of active sites responsible for the high charge transfer rate.
Therefore, D-CCO demonstrates better OER properties than C-CCO and recently reported perovskite OER catalysts including low Tafel slope and overpotential, quick charge transfer, along with remarkable long-term flexibility. One benefit is that the amorphization of CCO perovskite nanostructures and nitrogen doping creates the structure defect that results in high active-site densities as well as large exposed surface areas that are required for the resultant CCO electrocatalytic activities for water oxidation.
4. Conclusion
In conclusion, we suggest that a straightforward urea reduction procedure can successfully convert a nanometer-sized CCO catalyst into an amorphous form and nitrogen doping also create defective sites. This inexpensive, readily available, and simple-to-build defective nanoscale CCO catalyst with disordered structure exhibits a lower overpotential of 265 mV at 10 mA cm−2, a low Tafel slope of 35.95 mV dec−1, along with significant anodic currents in alkaline solutions. The D-CCO nanocatalyst also demonstrates outstanding stability for catalysis, with virtually full activity retention after 50 h. However, several characterization techniques, such as XRD confirm the synthesis of urea aid towards defective CCO and their diffused and agglomerated morphology was also observed via SEM. The BET analysis also showed a larger surface area of D-CCO (89 m2 g−1) than the C-CCO (55 m2 g−1). In addition to confirming a highly muddled nanoscale structure and an optimized electronic arrangement with many defects, this material analysis also provided a fine explanation for the electrochemical behaviours that resulted in OER’s accelerated activity and extraordinary durability. A possible active, highly stable and reasonably priced electrocatalyst may be produced by combining perovskite nanoparticles with the amorphous state. Our finding opens a new avenue for sutural engineering to enhance the electrochemical property of the electrocatalyst towards water splitting and it can be employed for solutions of other environmental issues.
Disclosure statement
No potential conflict of interest was reported by the author(s).
Data availability statement
Data will be made available on reasonable request.
Additional information
Funding
References
- Abdelkader A, Rabeh A, Mohamed Ali D, et al. Multi-objective genetic algorithm based sizing optimization of a stand-alone wind/PV power supply system with enhanced battery/supercapacitor hybrid energy storage. Energy. 2018;163:351–363. doi:10.1016/J.ENERGY.2018.08.135
- Onar OC, Uzunoglu M, Alam MS. Dynamic modeling, design and simulation of a wind/fuel cell/ultra-capacitor-based hybrid power generation system. J Power Sources. 2006;161:707–722. doi:10.1016/J.JPOWSOUR.2006.03.055
- Onar OC, Uzunoglu M, Alam MS. Modeling, control and simulation of an autonomous wind turbine/photovoltaic/fuel cell/ultra-capacitor hybrid power system. J Power Sources. 2008;185:1273–1283. doi:10.1016/J.JPOWSOUR.2008.08.083
- Zhang G, Tang X, Qi Z. Research on battery supercapacitor hybrid storage and its application in MicroGrid. Asia-Pacific Power Energy Eng Conf APPEEC. 2010. doi:10.1109/APPEEC.2010.5448231
- Shrestha NK, Patil SA, Han J, et al. Chemical etching induced microporous nickel backbones decorated with metallic Fe@hydroxide nanocatalysts: an efficient and sustainable OER anode toward industrial alkaline water-splitting. J Mater Chem A. 2022;10:8989–9000. doi:10.1039/D1TA10103J
- Do TN, You C, Kim J. A CO2 utilization framework for liquid fuels and chemical production: techno-economic and environmental analysis. Energy Environ Sci. 2022;15:169–184. doi:10.1039/D1EE01444G
- Saha D, Desipio MM, Hoinkis TJ, et al. Influence of hydrogen peroxide in enhancing photocatalytic activity of carbon nitride under visible light: an insight into reaction intermediates. J Environ Chem Eng. 2018;6:4927–4936. doi:10.1016/J.JECE.2018.07.030
- Stambouli AB, Traversa E. Solid oxide fuel cells (SOFCs): a review of an environmentally clean and efficient source of energy. Renew Sustain Energy Rev. 2002;6:433–455. doi:10.1016/S1364-0321(02)00014-X
- Zhou CH, Xia X, Lin CX, et al. Catalytic conversion of lignocellulosic biomass to fine chemicals and fuels. Chem Soc Rev. 2011;40:5588–5617. doi:10.1039/C1CS15124J
- Macfarlane DR, Tachikawa N, Forsyth M, et al. Energy applications of ionic liquids. Energy Environ Sci. 2013;7:232–250. doi:10.1039/C3EE42099J
- Wang T, Chen HC, Yu F, et al. Boosting the cycling stability of transition metal compounds-based supercapacitors. Energy Storage Mater. 2019;16:545–573. doi:10.1016/j.ensm.2018.09.007
- Winter M, Brodd RJ. What are batteries, fuel cells, and supercapacitors? Chem Rev. 2004;104:4245–4269. doi:10.1021/CR020730K/ASSET/IMAGES/MEDIUM/CR020730KE00045.GIF
- Mei J, Liao T, Ayoko GA, et al. Cobalt oxide-based nanoarchitectures for electrochemical energy applications. Prog Mater Sci. 2019;103:596–677. doi:10.1016/J.PMATSCI.2019.03.001
- Liu H, Tao HB, Liu B. Kinetic insights of proton exchange membrane water electrolyzer obtained by operando characterization methods. J Phys Chem Lett. 2022;13:6520–6531. doi:10.1021/ACS.JPCLETT.2C01341/ASSET/IMAGES/LARGE/JZ2C01341_0007.JPEG
- Wang C, Wang D, Ma X, et al. Isotropy-induced stress relaxation and strong-tolerance for high-rate and long-duration sodium storage by amorphous structure engineering. Adv Funct Mater. 2022;32:2204687, doi:10.1002/ADFM.202204687
- Bae Y, Hong J. Enhancement of surface morphology and catalytic kinetics of NiAl2O4 spinel-derived Ni catalyst to promote dry reforming of methane at low temperature for the direct application to a solid oxide fuel cell. Chem Eng J. 2022;446:136978, doi:10.1016/J.CEJ.2022.136978
- Azzouzi M, Gallop NP, Eisner F, et al. Reconciling models of interfacial state kinetics and device performance in organic solar cells: impact of the energy offsets on the power conversion efficiency. Energy Environ Sci. 2022;15:1256–1270. doi:10.1039/D1EE02788C
- Yasin G, Ali S, Ibraheem S, et al. Simultaneously engineering the synergistic-effects and coordination-environment of dual-single-atomic iron/cobalt-sites as a bifunctional oxygen electrocatalyst for rechargeable zinc-Air batteries. ACS Catal. 2023;13:2313–2325. doi:10.1021/ACSCATAL.2C05654
- Musa A B, Tabish M, Kumar A, et al. Microenvironment engineering of Fe-single-atomic-site with nitrogen coordination anchored on carbon nanotubes for boosting oxygen electrocatalysis in alkaline and acidic media. Chem Eng J. 2023;451:138684, doi:10.1016/J.CEJ.2022.138684
- Yasin G, Ibrahim S, Ibraheem S, et al. Defective/graphitic synergy in a heteroatom-interlinked-triggered metal-free electrocatalyst for high-performance rechargeable zinc–air batteries. J Mater Chem A. 2021;9:18222–18230. doi:10.1039/D1TA05812F
- Yasin G, Ibraheem S, Ali S, et al. Defects-engineered tailoring of tri-doped interlinked metal-free bifunctional catalyst with lower Gibbs free energy of OER/HER intermediates for overall water splitting. Mater Today Chem. 2022;23:100634, doi:10.1016/J.MTCHEM.2021.100634
- Ibraheem S, Yasin G, Kumar A, et al. Iron-cation-coordinated cobalt-bridged-selenides nanorods for highly efficient photo/electrochemical water splitting. Appl Catal B Environ. 2022;304:120987. doi:10.1016/J.APCATB.2021.120987
- Yasin G, Ibrahim S, Ajmal S, et al. Tailoring of electrocatalyst interactions at interfacial level to benchmark the oxygen reduction reaction. Coord Chem Rev. 2022;469:214669. doi:10.1016/J.CCR.2022.214669
- Cong Y, Huang S, Mei Y, et al. Metal–organic frameworks-derived self-supported carbon-based composites for electrocatalytic water splitting. Chem – A Eur J. 2021;27:15866–15888. doi:10.1002/CHEM.202102209
- Ejigu A, Fujisawa K, Spencer BF, et al. On the role of transition metal salts during electrochemical exfoliation of graphite: antioxidants or metal oxide decorators for energy storage applications. Adv Funct Mater. 2018;28:1804357. doi:10.1002/ADFM.201804357
- Sultan S, Tiwari JN, Singh AN, et al. Single atoms and clusters based nanomaterials for hydrogen evolution, oxygen evolution reactions, and full water splitting. Adv Energy Mater. 2019;9:1900624. doi:10.1002/AENM.201900624
- Zhu Y, Zhou W, Yu J, et al. Enhancing electrocatalytic activity of perovskite oxides by tuning cation deficiency for oxygen reduction and evolution reactions. Chem Mater. 2016;28:1691–1697. doi:10.1021/ACS.CHEMMATER.5B04457/ASSET/IMAGES/LARGE/CM-2015-044574_0003.JPEG
- Aftab S, Nawaz T, Bilal Tahir M. Recent development in shape memory based perovskite materials for energy conversion and storage applications. Int J Energy Res. 2021;45:20545–20558. doi:10.1002/ER.7151
- Dai J, Zhu Y, Zhong Y, et al. Enabling high and stable electrocatalytic activity of iron-based perovskite oxides for water splitting by combined bulk doping and morphology designing. Adv Mater Interfaces. 2019;6:1801317. doi:10.1002/ADMI.201801317
- Xu X, Su C, Zhou W, et al. Co-doping strategy for developing perovskite oxides as highly efficient electrocatalysts for oxygen evolution reaction. Adv Sci. 2016;3:1500187. doi:10.1002/ADVS.201500187
- Lah NA C. Late transition metal nanocomplexes: applications for renewable energy conversion and storage. Renew Sustain Energy Rev. 2021;145:111103. doi:10.1016/J.RSER.2021.111103
- Reddy DA, Kim HK, Kim Y, et al. Multicomponent transition metal phosphides derived from layered double hydroxide double-shelled nanocages as an efficient non-precious co-catalyst for hydrogen production. J Mater Chem A. 2016;4:13890–13898. doi:10.1039/C6TA05741A
- Askari MB, Salarizadeh P, Beitollahi H, et al. Electro-oxidation of hydrazine on NiFe2O4-rGO as a high-performance nano-electrocatalyst in alkaline media. Mater Chem Phys. 2022;275:125313. doi:10.1016/J.MATCHEMPHYS.2021.125313
- Wang M, Zhang L, He Y, et al. Recent advances in transition-metal-sulfide-based bifunctional electrocatalysts for overall water splitting. J Mater Chem A. 2021;9:5320–5363. doi:10.1039/D0TA12152E
- Li N, Cai L, Wang C, et al. Identification of the active-layer structures for acidic oxygen evolution from 9R-BaIrO3 electrocatalyst with enhanced iridium mass activity. J Am Chem Soc. 2021;143:18001–18009. doi:10.1021/JACS.1C04087/SUPPL_FILE/JA1C04087_SI_001.PDF
- Liu J, Guo L. In situ self-reconstruction inducing amorphous species: a key to electrocatalysis. Matter. 2021;4:2850–2873. doi:10.1016/J.MATT.2021.05.025
- Li L, Shao Q, Huang X. Amorphous oxide nanostructures for advanced electrocatalysis. Chem – A Eur J. 2020;26:3943–3960. doi:10.1002/CHEM.201903206
- Hamza MA, Abd El-Rahman SA, El-Shazly AN, et al. Facile one-pot ultrasonic-assisted synthesis of novel Ag@RGO/g-C3N4 ternary 0D@2D/2D nanocomposite with enhanced synergetic tandem adsorption-photocatalytic degradation of recalcitrant organic dyes: kinetic and mechanistic insights. Mater Res Bull. 2021;142:111386. doi:10.1016/J.MATERRESBULL.2021.111386
- Gao L, Cui X, Sewell CD, et al. Recent advances in activating surface reconstruction for the high-efficiency oxygen evolution reaction. Chem Soc Rev. 2021;50:8428–8469. doi:10.1039/D0CS00962H
- Xia D, Gao H, Li M, et al. Transition metal vanadates electrodes in lithium-ion batteries: a holistic review. Energy Storage Mater. 2021;35:169–191. doi:10.1016/J.ENSM.2020.10.023
- García Núñez C, Manjakkal L, Dahiya R. Energy autonomous electronic skin. Npj Flex Electron. 2019;3:1–24. doi:10.1038/s41528-018-0045-x
- Lv J, Wang L, Li R, et al. Constructing a hetero-interface composed of oxygen vacancy-enriched Co3O4 and crystalline-amorphous NiFe-LDH for oxygen evolution reaction. ACS Catal. 2021;11:14338–14351. doi:10.1021/ACSCATAL.1C03960/ASSET/IMAGES/LARGE/CS1C03960_0009.JPEG
- Xuchen L, Tingxian X, Xianghong D. Preparation and characterization of LaNiO3 A/F ratio-sensitive thin film by sol–gel process based on amorphous citrate precursors. Sensors Actuators B Chem. 2000;67:24–28. doi:10.1016/S0925-4005(99)00379-2
- Khan K, Tareen AK, Aslam M, et al. Recent advances in two-dimensional materials and their nanocomposites in sustainable energy conversion applications. Nanoscale. 2019;11:21622–21678. doi:10.1039/C9NR05919A
- Deng C, Wang Z, Feng L, et al. Electrocatalysis of sulfur and polysulfides in Li–S batteries. J Mater Chem A. 2020;8:19704–19728. doi:10.1039/D0TA05964A
- Adhikari S, Murmu M, Kim DH. Core-shell engineered WO3 architectures: recent advances from design to applications. Small. 2022;18:2202654. doi:10.1002/SMLL.202202654
- El-Shazly AN, Hamza MA, Allam NK. Enhanced photoelectrochemical water splitting via engineered surface defects of BiPO4 nanorod photoanodes. Int J Hydrogen Energy. 2021;46:23214–23224. doi:10.1016/J.IJHYDENE.2021.04.134
- Fawzy SM, Omar MM, Allam NK. Photoelectrochemical water splitting by defects in nanostructured multinary transition metal oxides. Sol Energy Mater Sol Cells. 2019;194:184–194. doi:10.1016/J.SOLMAT.2019.02.011
- Zhu Y, Zhong X, Jin S, et al. Oxygen defect engineering in double perovskite oxides for effective water oxidation. J Mater Chem A. 2020;8:10957–10965. doi:10.1039/D0TA04362A
- Wu J, Guo Y, Liu H, et al. Room-temperature ligancy engineering of perovskite electrocatalyst for enhanced electrochemical water oxidation. Nano Res. 2019;12:2296–2301. doi:10.1007/S12274-019-2409-5
- Song S, Zhou J, Su X, et al. Operando X-ray spectroscopic tracking of self-reconstruction for anchored nanoparticles as high-performance electrocatalysts towards oxygen evolution. Energy Environ Sci. 2018;11:2945–2953. doi:10.1039/C8EE00773J
- Shi Z, Wang X, Ge J, et al. Fundamental understanding of the acidic oxygen evolution reaction: mechanism study and state-of-the-art catalysts. Nanoscale. 2020;12:13249–13275. doi:10.1039/D0NR02410D
- Zhai Y, Ren X, Yan J, et al. High density and unit activity integrated in amorphous catalysts for electrochemical water splitting. Small Struct. 2021;2:2000096. doi:10.1002/SSTR.202000096
- Baxter J, Bian Z, Chen G, et al. Nanoscale design to enable the revolution in renewable energy. Energy Environ Sci. 2009;2:559–588. doi:10.1039/B821698C
- Cummins DR, Martinez U, Sherehiy A, et al. Efficient hydrogen evolution in transition metal dichalcogenides via a simple one-step hydrazine reaction. Nat Commun. 2016;7:1–10. doi:10.1038/ncomms11857
- Park S, Hu Y, Hwang JO, et al. Chemical structures of hydrazine-treated graphene oxide and generation of aromatic nitrogen doping. Nat Commun. 2012;3:1–8. doi:10.1038/ncomms1643
- Ghobadifard M, Feizi G, Mohebbi S. Enhanced photocatalytic conversion of organic dyes using CeCoO3/MoS2 heterojunction as a highly effective visible-light-driven photocatalyst. Appl Organomet Chem. 2022;36:e6911. doi:10.1002/AOC.6911
- Chen N, Du YX, Zhang G, et al. Amorphous nickel sulfoselenide for efficient electrochemical urea-assisted hydrogen production in alkaline media. Nano Energy. 2021;81:105605. doi:10.1016/J.NANOEN.2020.105605
- El-Shazly AN, Hegazy AH, El Shenawy ET, et al. Novel facet-engineered multi-doped TiO2 mesocrystals with unprecedented visible light photocatalytic hydrogen production. Sol Energy Mater Sol Cells. 2021;220:110825. doi:10.1016/J.SOLMAT.2020.110825
- Samsudin AS, Saadiah MA. Ionic conduction study of enhanced amorphous solid bio-polymer electrolytes based carboxymethyl cellulose doped NH4Br. J Non Cryst Solids. 2018;497:19–29. doi:10.1016/J.JNONCRYSOL.2018.05.027
- Kim T, Jung G, Yoo S, et al. Activated graphene-based carbons as supercapacitor electrodes with macro- and mesopores. ACS Nano. 2013;7:6899–6905. doi:10.1021/NN402077V/SUPPL_FILE/NN402077V_SI_001.PDF
- Wang R, Li Y, He YL. Achieving gradient-pore-oriented graphite felt for vanadium redox flow batteries: meeting improved electrochemical activity and enhanced mass transport from nano- to micro-scale. J Mater Chem A. 2019;7:10962–10970. doi:10.1039/C9TA00807A
- Zhao S, Luo Y, Li C, et al. High-performance photothermal catalytic CO2 reduction to CH4 and CO by ABO3 (A=La, Ce; B=Ni, Co, Fe) perovskite nanomaterials. Ceram Int. 2023;49:20907–20919. doi:10.1016/J.CERAMINT.2023.03.224
- Idriss H. On the wrong assignment of the XPS O1s signal at 531–532 eV attributed to oxygen vacancies in photo- and electro-catalysts for water splitting and other materials applications. Surf Sci. 2021;712:121894. doi:10.1016/J.SUSC.2021.121894
- Paul A, Ghosh S, Kolya H, et al. New insight into the effect of oxygen vacancies on electrochemical performance of nickel-tin oxide/reduced graphene oxide composite for asymmetric supercapacitor. J Energy Storage. 2023;62:106922. doi:10.1016/J.EST.2023.106922
- Gross P, Höppe HA. Unravelling the urea-route to boron nitride: synthesis and characterization of the crucial reaction intermediate ammonium bis(biureto)borate. Chem Mater. 2019;31:8052–8061. doi:10.1021/ACS.CHEMMATER.9B02515/ASSET/IMAGES/MEDIUM/CM9B02515_M002.GIF
- Anantharaj S, Noda S. Amorphous catalysts and electrochemical water splitting: an untold story of harmony. Small. 2020;16:1905779. doi:10.1002/SMLL.201905779
- Li Z, Xie Y, Huang Z, et al. Amorphization of LaCoO3 perovskite nanostructures for efficient oxygen evolution. ACS Appl Nano Mater. 2022;5:14209–14215. doi:10.1021/ACSANM.2C02982/ASSET/IMAGES/LARGE/AN2C02982_0005.JPEG
- Arandiyan H, Mofarah SS, Wang Y, et al. Impact of surface defects on LaNiO3 perovskite electrocatalysts for the oxygen evolution reaction. Chem – A Eur J. 2021;27:14418–14426. doi:10.1002/CHEM.202102672
- Swathi S, Yuvakkumar R, Ravi G, et al. Rare earth metal (Sm)-doped NiMnO3 nanostructures for highly competent alkaline oxygen evolution reaction. Nanoscale Adv. 2022;4:2501–2508. doi:10.1039/D2NA00022A
- Du J, Zhang T, Cheng F, et al. Nonstoichiometric perovskite CaMnO3-δ for oxygen electrocatalysis with high activity. Inorg Chem. 2014;53:9106–9114. doi:10.1021/IC501631H/SUPPL_FILE/IC501631H_SI_001.PDF
- Junita J, Jayalakshmi D, Rodney JD. Combustion-derived BaNiO3 nanoparticles as a potential bifunctional electrocatalyst for overall water splitting. Int J Hydrogen Energy. 2023;48:14287–14298. doi:10.1016/J.IJHYDENE.2022.12.291
- Weng Z, Huang H, Li X, et al. Coordination tailoring of epitaxial perovskite-derived iron oxide films for efficient water oxidation electrocatalysis. ACS Catal. 2023;37:2751–2760. doi:10.1021/ACSCATAL.2C05147/ASSET/IMAGES/LARGE/CS2C05147_0004.JPEG
- Wang Q, Wang H, Qi S, et al. Coral-Like LaNixFe1−xO3 perovskite catalyst for high-performance oxygen evolution reaction. J Electrochem Soc. 2022;169:026508. doi:10.1149/1945-7111/AC4AB0
- Zhang ZH, Zhang Y, Barras A, et al. Preparation of flower-shaped Co-Fe layer double hydroxide nanosheets loaded with Pt nanoparticles by corrosion engineering for efficient electrocatalytic water splitting. ACS Appl Energy Mater. 2022;5:15269–15281. doi:10.1021/ACSAEM.2C02905/ASSET/IMAGES/LARGE/AE2C02905_0009.JPEG
- Li J, Shu C, Hu A, et al. Tuning oxygen non-stoichiometric surface via defect engineering to promote the catalysis activity of Co3O4 in Li-O2 batteries. Chem Eng J. 2020;381:122678. doi:10.1016/J.CEJ.2019.122678
- Huang X, Shen T, Zhang T, et al. Efficient oxygen reduction catalysts of porous carbon nanostructures decorated with transition metal species. Adv Energy Mater. 2020;10:1900375. doi:10.1002/AENM.201900375
- Hu F, Zhu S, Chen S, et al. Amorphous metallic NiFeP: a conductive bulk material achieving high activity for oxygen evolution reaction in both alkaline and acidic media. Adv Mater. 2017;29:1606570. doi:10.1002/ADMA.201606570