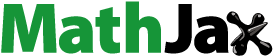
Abstract
Analysis of nanofluid flow phenomena through a stretching sheet in a non-Darcy porous medium has great importance in enhancing transport processes in energy systems like hybrid fuel cell technology, glass blowing, etc. The current study looks at two-dimensional flow over a convectively heated linear stretching sheet in presence of a magnetic field. The boundary layer flow caused by a sheet that is linearly stretched has been investigated numerically. Employing the appropriate similarity transformation, the governing partial differential equations, which define the flow regime, are converted into a set of ordinary differential equations. The mathematical calculations are carried out using a finite difference algorithm with the aid of Newton's linearization method, which allows us to handle non-linear terms very smoothly. The outcomes of this work have been demonstrated are based on eight parameters, such as Prandtl number , Lewis number
, Brownian motion parameter
, thermophoresis parameter
, magnetic parameter
, porosity parameter
, Frochemier number
, and convection Biot number
. The impacts of aforementioned parameters on thermal and concentration boundary layers are depicted graphically. It has been found that the heat transfer in the boundary layer rises as
enhances, and deposits aggravating particle away from the fluid region and increases the volume percentage of nanoparticles. Moreover, it has been found that as the
number rises, the concentration profiles become steeper and the species border layer becomes thinner. Furthermore, it has been encountered that the reduced Sherwoord number rises as Le number increases for
but decreases as
number increases for
.
Nomenclature
b | = | favourable constant related to linear stretching |
= | Biot number | |
= | coefficient of drag | |
= | coefficient of Brownian diffusion | |
Dl | = | coefficient of thermophoretic diffusion |
g | = | acceleration due to gravity |
h | = | coefficient of convective heat transfer |
k | = | thermal conductivity of base fluid |
= | Lewis number | |
= | brownian motion parameter | |
= | thermophoresis parameter | |
= | Nusselt number | |
= | reduced Nusselt number | |
= | Prandtl number | |
P | = | Pressure |
= | surface (wall) heat flux | |
= | surface wall mass flux | |
= | local Reynolds number | |
= | Sherwood number | |
= | reduced Sherwood number | |
= | temperature of hot fluid | |
= | magnetic parameter | |
= | dimensional parameter at the sheet surface | |
= | large values of | |
HT | = | rate of heat transfer |
TC | = | thermal conductivity |
HE | = | heat exchange |
NF | = | Nanofluid |
NP | = | Nanoparticle |
DF | = | Darcy-Forchheimer |
= | sheet surface (wall) temperature | |
= | ambient temperature | |
= | non-uniform inertia coefficient of porous medium | |
= | components of velocity along the directions of x and y | |
x | = | coordinate along the sheet |
y | = | coordinate normal to the sheet |
D | = | nanoparticle volume fraction |
= |
| |
= |
| |
= | thermal diffusivity of base fluid | |
= | non-dimensional form of | |
= | similarity variable | |
= | dimensionless temperature | |
= | absolute viscosity of base fluid | |
= | kinematic viscosity of base fluid | |
= | base fluid density | |
= | nanoparticle mass density | |
= | stream function | |
= | dimensionless concentration at sheet surface | |
= | ||
= | fluid temperature | |
= | inertia coefficient parameter | |
= | permeability of porous medium | |
= | porosity parameter | |
FDM | = | finite difference method |
= | non-dimensional stream function | |
HMT | = | heat and mass transfer |
TBL | = | thermal boundary layer |
NDP | = | non-Darcy porous |
CC | = | Cattaneo-Christov |
1. Introduction
The manipulation of NF’s involves immersing of NP’s of an average size of less than nm in conventional HT fluids like ethylene glycol, oil, and water. Thermal transmission of water, oil, and ethylene glycol with NP’s simulates a significant portion of the HT between the HMT medium and surface. The fluids’ heat transmission behaviour has been significantly improved due to the increase in the TC. Many engineering applications require high heat transfer efficiency. The TC of NF varies linearly with temperature and variable in many engineering and industrial uses. The mixture of water and nanomaterials is known as a nanofluid. Thermal conductivity and convective heat movement are improved when a surfactant is added to a base fluid. A modern and efficient method of heat transmission is nanofluid technology. Nanofluids have applications in cancer therapy, surgery based on heat treatment applications, as well as solar energy applications. Better oils and lubricants can be made using nanofluid technology for practical uses [Citation1]. Over the last few decades, several researchers have been working on fluid to increase efficiency for various thermal uses. By utilizing nanotechnology for HT, the word “NF” is used to discuss about new HT issues [Citation2–7]. Numerous authors have projected that NF’s will be more effective at convective HT than basic fluids. Nanofluids’ ability to transfer heat and mass has incorporated many practical uses for thermal and subatomic stratification [Citation8–13]. Patil et al. [Citation14] studied an unsteady mixed convection NF flow in an exponentially stretching surface with a transverse magnetic field. Using homotopy analysis, [Citation15] showed the entropy analysis and gyrotactic microorganisms of Buongiorno's NF within a pair of stretchable rotating discs. Nainaru et al. [Citation16] showed that how HT characteristics impact on 3D MHD flows of a NF on stretching a surface using thermal radiation. They noticed that the variable TC increased along with the fluid temperature and movement. [Citation17] studied the forced convection NF flow through a horizontal microchannel with cross-flow injection and slip velocity on sidewalls of the microchannel. Increasing the angle of injection up to 940, they noted that both velocity slip and HT rate are increased. Suhail and Siddiqui [Citation18] studied the natural convection NF flows through a vertical annular region numerically. They discussed the simulations for different heat flux values and nanoparticle concentrations.
The convective boundary layer is crucial for supplying heat to the fluid via a particle surface with a limited HE because of its numerous and practical applications, particularly in various manufacturing processes like transpiration cooling, laser pulse heating etc. [Citation19–21]. Convective HT is crucial in a variety of devices, including thermal insulation, gas turbines, and nuclear power plants [Citation22,Citation23]. Magnetohydrodynamics is the study of electrically conducting fluids, such as seawater, antioxidants, plasma, and metal compounds (MHD). The acronym MHD was created by Alfven [Citation24]. MHD is influenced by magnetic induction intensity. Numerous technical and commercial uses for the MHD fluid flow include crystal growth, nuclear freezing, MHD detectors, and energy generation, which has numerous uses in the medical and biopharmaceutical industries, including hyperglycemia, emergency treatment, radiation therapy, and many others. Using the Keller box method, [Citation25] evaluated the melting HT and non-uniform heat source on magnetic NF flow past a porous cylinder. Jha and Malgwi [Citation26] examined the effects of buoyant forces, pressure gradients, Hall and Ionslip currents on the mixed MHD convection flow of a viscous fluid through a microchannel placed vertically. Using explicit finite difference method, Haque [Citation27] investigated the impacts of magnetic field and a heat sink on the micropolar fluid flows through a semi-infinite vertical porous plate. They discovered that the species concentration is influenced by both thermal diffusion and heat sink and that lighter particles concentrated in the fluid highly than heavier particles Alam et al. [Citation28] investigated the biomagnetic flows of blood with gold nanoparticles over a stretched surface with suction, and velocity slip using bvp4c method. They took into consideration the principles of magneto hydrodynamics and ferrohydrodynamics.
Porous media is a surface with fluid-filled holes (voids). Reverse osmosis, heterogeneous catalysts, and evaporative freezing are among the industrial applications for porous materials [Citation29–31]. Researchers are engaged in analyzing the fluid flow processes in porous media for having numerous applications in production of scientific, biological, and industrial goods, including processing of crude oil, casting etc. [Citation32–36]. Darcy established a theory in 1856 that asserts the pressure gradient is directly related to the volumetric flux of fluid across a medium. Practically, the Darcy term [Citation37] is frequently applied to modelling and analysis issues involving flow-saturating porous regions. Only when the velocity is low and the porosity is low does Darcy's rule apply. This rule does not take into account inertia or boundary effects. The NDP space that integrates inertial and boundary impacts is the outcome of a modified version of the traditional Darcy's principle. As a consequence, Forchheimer [Citation38] used a term in the momentum term to account for inertia. Non-Darcian variants are variations on the basic Darcy idea, which also takes into account inertial drag, vorticity dispersion, and their combined effects. It is claimed that the Darcy law fails in the presence of high velocities, highly porous media, and extremely high
. This technique incorporates the Forchheimer expression into the square velocity term of the momentum equation to account for the inertia effects of pressure drop. Temperature shifts in HMT flows of DP and NDP media were studied by Pop, and Ingham [Citation39] and Vafai [Citation40]. Using the ND solve method, [Citation41] performed the DF 3D flows of NF over a rotating disc considering activation energy effects and increase and decrease in heat. Using the shooting method, Asma et al. [Citation42] investigated the convective HT of DF 3D flows of NF through a rotating disc with Arrhenius activation energy. [Citation43] used the bvp4c technique to study the impacts of DF permeable media, CC heat flux, and homogeneous-heterogeneous reactions on the melting HT between two parallel discs. Using the successive over-relaxation method, [Citation44] performed the MHD convection flows of a hybrid NF past a stretching porous surface with viscous dissipation impacts. By homotopy analysis method, Kareem and Abdulhadi [Citation45] looked into the axisymmetric MHD DF flows of
grade fluid over a stretching cylinder considering the CC effects. Use of homotopy analysis scheme has been made, Jawad et al. [Citation46] to provide the entropy analysis of MHD radiative DF 3D Casson NF flow over a rotating disc taking into account the Arrhenius activation energy. Buongiorno's model was employed by Sheremet and Pop [Citation47] to quantitatively analyze the NF flow in the cavity. In recent years, Bhatti et al. [Citation48] studied the flow of an incompressible, 3-D, unsteady, axisymmetric squeezed Williamson NF between circular and rotating parallel plates with magnetic field, porosity, and suspension of motile gyrotactic microorganisms. They concluded that the axial and tangential velocity curves fall as the squeezing
rises. In addition, they stated that the magnetic field weakens both axially and tangentially as the magnetic
rises.
Despite the highly anticipated future of Buongiorno NF flow over a stretching surface saturated with a NDP medium, no study has been done till now. The majority of the work has been performed on DP medium for different types of NF flow. The aforementioned reasons encourage us to accomplish the current work. In the current manuscript, the non-Darcian NF flow and HT across a stretched surface in a NF-saturated porous media has been addressed. The aim is to study the influence of , and
of non-Darcy NF over a stretching surface with magnetohydrodynamics. Using the finite difference approach and Newton's linearization technique, the system of coupled nonlinear differential equations has been numerically solved. Graphical representations have been made of how embedded factors affect fluid velocity, temperature, and particle concentration. There is a good agreement between the findings of the current research and those of [Citation49–52]. This work is an extension of the study of Makinde and Aziz [Citation51], Khan and Pop [Citation52], Kuznetsov and Nield [Citation53], and Nield and Kuznetsov [Citation54]. It is anticipated that the findings will complement earlier research in addition to offering useful information for applications. The findings in this study are particularly beneficial to many technical communities, including those that work with transpiration cooling, fabric cleaning, laser pulse heating, etc. The authors claim that no previous integrated effort of this nature has been made so far.
2. Geometry configuration
We have considered the steady and incompressible MHD nano fluid flow induced by linear movement of the stretching sheet with a linear velocity variation with distance i.e.
(1)
(1) embedded in a fully saturated NDP medium. The physical model has been illustrated in Figure . With the exception of the fact that the current problem involves the boundary layer flow of nanofluid contained in a non-Darcy porous medium, the geometry of the problem is similar to that of Makinde and Aziz’s [Citation51] work. It is supposed that
and
acquire constant values
and
, respectively, at the stretching surface. Here,
and
are indicated by
and
, when
In the normal direction,
(strong magnetic field) is employed. The effects of viscous and ohmic discharge are both ignored. By selecting a small
, the aspects of the induced magnetic field are disregarded.
3. Mathematical formulation
The work of Makinde and Aziz [Citation51], Khan and Pop [Citation52], Kuznetsov and Nield [Citation53], and Nield and Kuznetsov [Citation54] has been extended in the present work to take into account Buogiorno NF flow through a NDP medium, which is governed by the following conservation of mass, momentum, thermal energy, and volume fraction equations in 2-D Cartesian coordinates.
(2)
(2)
(3)
(3)
(4)
(4)
(5)
(5)
(6)
(6)
As per the studies of Makinde and Aziz [Citation51], Khan and Pop [Citation52], Kuznetsov and Nield [Citation53], and Nield and Kuznetsov [Citation54], the following boundary conditions have opted for.
(7)
(7)
(8)
(8)
The following dimensionless quantities are introduced,
(9)
(9) where
(10)
(10)
The equation (2) can satisfied by the equation (10). Following is an analysis of the direction momentum equation (normal to the sheet) by order of magnitude employing the standard BL approximations:
(11)
(11)
(12)
(12)
Using the concept of equations (11–12) in equation (4), we have
(13)
(13)
Now, the equations (2–8) are thus reduced to the following set of equations (14–16) when the pressure gradient in the y direction is omitted (equation 13).
(14)
(14)
(15)
(15)
(16)
(16)
The boundary conditions (7–8) become,
(17)
(17)
(18)
(18)
Due to the buoyancy effects caused by the gradients in nanoparticle concentration, there is no heat transfer when . Here, we perceive that equation 14 has a closed-form solution, which is given by
, along with the corresponding boundary conditions on
provided by expression (17).
4. Numerical calculations and validation
Using the boundary conditions (17–18), the equations (14–16) have been solved by FDM. At the outset, the finite difference equation corresponding to the order ordinary differential equation (14) is derived as follows:
Using the substitution of
(19)
(19) in equation 14, we have
(20)
(20)
Using the concept of finite difference form in equation 20, we obtain
(21)
(21) Since the preceding equation is non-linear, the accompanying generalization of Newton's linearization technique is employed to linearize the equation, which is then followed by the Thomas algorithm. In this procedure, the variable
’s solution at the
step is written as
(22)
(22)
The linearized forms of equations (21–22) generate a system of tri-diagonal equations as follows:
(23)
(23)
Where and
are at the boundaries since the solution at
and
as
,
are known.
at
and
is zero. The expression for
and
in equation 23 are given:
(24)
(24)
The linear system of equation 23 is solved to determine
. Subsequently, the solution of
is modified through equations (20–21), where
is obtained by Tri diagonal matrix algorithm (TDMA) from the following equation
(26)
(26)
Similarly non-linear order ordinary differential equations (15–16) are expressed as below:
(27)
(27)
(28)
(28)
Equations (27–28) are also solved numerically in the similar way. The numerical outcomes of are compared with studies of [Citation49–52] in Table to evaluate the accuracy of our numerical calculations. These asserted to circumstances where the temperature of the stretching sheet is fixed and thermophoresis effects are not present. The findings presented here to agree to three decimal places with those of [Citation49–52] for various values of
listed in table . The findings in table show a pattern resembling natural convection from a vertical plate in a regular fluid, where the
rises as the
rises.
Table 1. Numerical outcomes for at
.
The constant temperature findings have been retrieved by putting in expression 17, yielding the condition
(isothermal condition). Figures (a-b) compare our findings for the
and
to those reported by [Citation52] at
The outcomes showed a flawless agreement between our findings and the results reported in [Citation52] for every combination value of
,
used in our computations. From the figure (a), it has been demonstrated that the profile of
sharply declines as
is increased from 0.1–0.5 for fixed
Even so, the
is improved by a rise in
(figure (b)), which is consistent with the findings of [Citation52]. The thermal boundary layer thickens as the Brownian motion expands, affecting a greater area of the fluid, which reduces the
.
5. Results and discussion
Using graphical representations, illustrative numerical findings are presented in this section. Calculations have been performed for various values of physical factors, like
5.1. Analysis of temperature profile
At various ,
and at
, Figure (a) displays the temperature distributions in the TBL. The
parameter has a significant effect on the nanoparticle volume fraction.
is the process of moving particles in such a way that the particles become cool under the influence of temperature gradient. With a rise in
values, a significant improvement in the temperature profile is seen due to the enhancement of molecular movement. HT in the boundary layer increases as
increases, aggravating particle deposition away from the fluid region and increasing the volume percentage of nanoparticles. Due to spontaneous diffusivity, the nanoparticles reconfigure themselves and form a novel structure, which in turn enhances the NF's TC. The particle fury and increase in the fluid temperature in the boundary layer caused by the
, increases the temperature distributions. With the increase of the values of
and
, it has been found that the surface temperature rises, the boundary layer thickens, and the curves become steep, as a result, the profile of
decreases. Figure (b) displays the profiles of temperature distributions for various values of
at
number has an impact on temperature profiles in a region near to the sheet only, as shown in figure (b), as the curves tend to merge further away from the sheet.
In the boundary layer region, number is a measure of the thermal diffusion rate, which contributes to the species diffusion rate. When
is equal to 1, heat and species disseminate equally, and when
is greater than 1.0, heat diffuses more quickly than species. When
number increases, the species border layer become thin, and the temperature profiles become steeper. At
the impact of
number on the distribution of temperature has been depicted in Figure (a). The
number links surface convection and solid conduction. Physically, the surface thermal resistance drastically decreases as the
number increases. The rise in convection raises the surface temperature. As the
number rises, the buoyancy force grows and the fluid transfers heat energy more quickly. The density of fluid changes with temperature due to thermal expandability, and the buoyancy force affects the movement. An inadequate convective thermal scenario is represented taking Bi = 0.1. Considering of high values of
number allows to achieve the constant wall temperature case
. A rise in
number is accompanied by a significant increase in the temperature profiles. The impact of
number on the distribution of temperature has been demonstrated in figure (b) at
In general, as
numbers increase, the temperature profiles tend to decrease. In engineering and production procedures, the parameter
is plays a crucial role. The
number relates force diffusivity to warm diffusivity. Thermal diffusivity varies inversely with
. For higher values of
, the viscosity of the fluid becomes high, and for lower values of
, the fluid becomes less viscous. With an increase in
values, a substantial reduction in temperature profiles is seen. This pattern resembles the boundary layer movement of a free convective fluid [Citation55]. The physical meaning of the
number's findings is thus very consistent.
5.2. Analysis of velocity profile
At fixed value of Figures (a) depicts the influence of M on the distribution of velocity. Velocity exhibits a decreasing tendency as M rises. With higher M values, a strong Lorentz effect significantly reduces the velocity. Applying a perpendicular magnetic field to an electrically conducting fluid, Lorentz force is produced. The velocity distribution diminishes due to the resistive nature of the Lorentz force, which reduces the motion of the particles. Higher values of the magnetic field indicate a larger body force, which can slow down the liquid flow and increases the thickness of the momentum boundary layer, as viscous force and body force are proportional to hydromagnetic force, as described by
. It shows unequivocally how the transverse magnetic field interferes with the transport phenomena. Figure (b) shows how the distribution of velocity is affected by the parameter
. The velocity profiles start to decay as
numbers rise. Porosity physically prevents fluid from moving freely, reducing the fluid's movement. The presence of permeable space improves the safety of the fluid stream by reducing the rate of the fluid. Porousness generally raises fluid flow resistance, which leads to thicker thermal boundary layers and higher temperature profiles. For fixed value of
the impact of the
number on the distribution of velocity has been shown in Figure . Since the inertia coefficient is directly proportional to the drag coefficient, higher values of
reduce the velocity profiles. As a result, the drag coefficient rises as
rises. As a response, the resistance force of the fluid rises, and the velocity decreases (figure ). A rise in
values of thickens of the thermal boundary layer makes difficult for the fluid to pass. The scientific justification for this fact is that raising the permeability values reduces the resistance of the porous medium, which tends to decrease the fluid velocity and improvements in the inertia coefficient
decrease the velocity due to a rise in the quadratic drag.
5.3. Analysis of concentration profile
Figure (a) presented the distribution of concentration for various values of at
A higher
suggests a lower Brownian diffusion coefficient for a base fluid of particular kinematic viscosity. In the boundary layer region,
number is a measure of how much the thermal diffusion rate contributes to the species diffusion rate. When
is equal to 1, heat and species disseminate equally, and when
is greater than 1.0, heat diffuses more quickly than species. As the
number rises, the concentration profiles become steeper and the species border layer becomes thinner. At
the distribution of concentration has been shown in figure (b) for various
and
. The nanoparticle volume fraction is greatly influenced by the parameter
. The procedure for transporting particles reduce temperature due to the effect of temperature gradient, which is known as
. The boundary layer's HT rises as
rises, aggravating particle deposition away from the fluid area and, as a result, increasing the volume fraction of nanoparticles. The
parameter gauges how much the suspended nanoparticles in the NF are moving randomly. Physically, an increase in the temperature causes the particles’ energy to grow, which in turn increases their random motion and rapid collision, i.e. increases the Brownian motion. Due to spontaneous diffusivity, the nanoparticles reconfigure themselves and form a novel structure, which in turn enhances the NF's TC. The Brownian motion pushes the particles away from the fluid system and heats the fluid in the boundary layer. As the values of
and
increases, the strength of the Brownian motion and thermophoresis are found to have lowest impact on the distribution of concentration.
5.4. Analysis of 
and 

Figure (a) has been illustrates the influence of number on the distribution of
.
measures the dimensionless HT at the sheet. The thickness of TBL's diminishes as the
number rises (figure b), while the steepness of the curves increases. It is observed that the profile of
increases as
increases, and decreases as
increases. This is because for greater values of
, the convection process is dominant compared to conduction, which increases the HT. The higher thermophoretic force that propels the high thermal conductivity nanoparticles from the hot sheet towards the quiescent fluid, and.
causes this decrease as a result of the increase in the parameter. Impact of
number on the distribution of
has been shown in figure (b). It has been found that the distribution of
decreases with the increase of the values of
number. Thermal boundary layer thickness rises with Le number, which in turn causes
to decrease. The influence of
number on
has been depicted in Figure . It has been found that the profile of
increases with the increase of
number. When the surface velocity exceeds the free stream velocity,
rises with an increase in
number for
but falls with an increase in
number for
greater than 0.1.
6. Conclusion
In the current article, the non-Darcian nanofluid flow and heat transfer across a stretched surface in a nano-fluid-saturated porous media with the influence of a magnetic field has been studied. Using suitable similarity transformations, the basic equations of flow are transformed into ODE's. Then, the mathematical calculations are carried out using a finite difference algorithm with the aid of Newton's linearization method. The effects of different flow-controlled variables have been illustrated numerically and graphically for the distributions of velocity, temperature, concentration, , and
. The important outcomes of this work are listed below:
It has been found that the distribution of fluid temperature and nanoparticle concentration enhances with the increase of the values of
, and
. With the increase of the values of
and
, the strength of the Brownian motion and thermophoresis has been found to have a minimal impact on the distribution of concentration.
It has been demonstrated that the increment of
number causes the reduction of temperature distribution. On the other hand, increasing
number enhances the temperature distribution.
It has been found that as the
number rises, the concentration profiles become steeper and the species border layer becomes thinner. In addition, it has been noticed that a rise in
number is accompanied by a significant increase in the temperature profiles.
It has been revealed that as the number of
,
, and
increases, the distribution of fluid velocity decreases. Moreover, it has been demonstrated that the profile of
increases as
increases, and decreases as
increases.
At the end, it has been encountered that the
number raises as
number increases for
but decreases as
number increases for
.
The obtained results of the current study will become very much helpful in various engineering applications such as glass blowing, spinning of fibers, and continuous casting of metals, particularly in various manufacturing processes like transpiration cooling, fabric cleaning, and laser pulse heating.
Acknowledgment
The authors are grateful to the reviewers for their significant comments and suggestions to improve the quality of the manuscript. The reviewer’s valuable comments helped us a lot to update the manuscript.
Disclosure statement
No potential conflict of interest was reported by the author(s).
References
- Ellahi R, Zeeshan A, Shehzad N, et al. Structural impact of kerosene-Al2O3 nanoliquid on MHD poiseuille flow with variable thermal conductivity: application of cooling process. J Mol Liq. 2018;264:607–615. doi:10.1016/j.molliq.2018.05.103
- Choi SU. Enhancing thermal conductivity of fluids with nanoparticles, developments and applications of non-newtonian flows. In: Siginer DA, Wang HP, editors. FED-The American society of mechanical engineers. New York: ASME; 1995; 231/MD(66). p. 99–105.
- Ellahi R, Hassan M, Zeeshan A. Study of natural convection MHD nanofluid by means of single and multi-walled carbon nanotubes suspended in a salt-water solution. IEEE Trans Nanotechnol. 2015;14:726–734. doi:10.1109/TNANO.2015.2435899
- Ellahi R, Zeeshan A, Waheed A, et al. Natural convection nanofluid flow with heat transfer analysis of carbon nanotubes–water nanofluid inside a vertical truncated wavy cone. Math Methods Appl Sci. 2021; doi:10.1002/mma.7281
- Ellahi R, Zeeshan A, Hussain F, et al. Thermally charged MHD bi-phase flow coatings with non-newtonian nanofluid and hafnium particles along slippery walls. Coatings. 2019;9:300. doi:10.3390/coatings9050300
- Ellahi R, Zeeshan A, Hussain F, et al. Study of shiny film coating on multi-fluid flows of a rotating disk suspended with nano-sized silver and gold particles: A comparative analysis. Coatings. 2018;8:422. doi:10.3390/coatings8120422
- Bezi S, Souayeh B, Ben-Cheik N. Numerical simulation of entropy generation due to unsteady natural convection in a semi-annular enclosure filled with nanofluid. Int J Heat Mass Transfer. 2018;124:841–859. doi:10.1016/j.ijheatmasstransfer.2018.03.109
- Souayeh B, Hammami F, Hdhiri N, et al. Unsteady state fluid structure of two-sided nonfacing lid-driven cavity induced by a semicircle at different radii sizes and velocity ratios. Int J Mod Phys C. 2019;30:1950060. doi:10.1142/S0129183119500608
- Ahmed N, Vieru D, Fetecau C, et al. Convective flows of generalized time-nonlocal nanofluids through a vertical rectangular channel. Phys Fluids. 2018;30:052002. doi:10.1063/1.5032165
- Tanveer M, Ullah S, Ali Shah N. Thermal analysis of free convection flows of viscous carbon nanotubes nanofluids with generalized thermal transport: a prabhakar fractional model. J Therm Anal Calorim. 2021;144:2327–2336. doi:10.1007/s10973-021-10643-3
- Zhang KZ, Shah NA, Vieru D, et al. Memory effects on conjugate buoyant convective transport of nanofluids in annular geometry: a generalized cattaneo law of thermal flux. Int Commun Heat Mass Transfer. 2022;135:106138. doi:10.1016/j.icheatmasstransfer.2022.106138
- Souayeh B, Ramesh K. Numerical scrutinization of ternary nanofluid flow over an exponentially stretching sheet with gyrotactic microorganisms. Mathematics. 2023;11:981. doi:10.3390/math11040981
- Batool S, Rasool G, Alshammari N, et al. Numerical analysis of heat and mass transfer in micropolar nanofluids flow through lid driven cavity: finite volume approach. Case Stud Therm Eng. 2022;37:102233. doi:10.1016/j.csite.2022.102233
- Patil PM, Shashikant A, Momoniat E. Transport phenomena in MHD mixed convective nanofluid flow. Int J Numer Methods Heat Fluid Flow. 2020;30:769–791. doi:10.1108/HFF-04-2019-0365
- Khan NS, Shah Q, Bhaumik A, et al. Entropy generation in bioconvection nanofluid flow between two stretchable rotating disks. Sci Rep. 2020;10:4448. doi:10.1038/s41598-020-61172-2
- Nainaru TPV, Narayana S, Venkateswarlu B. Numerical simulation of variable thermal conductivity on 3D flow of nanofluid over a stretching sheet. Nonlinear Eng. 2020;9:233–243. doi:10.1515/nleng-2020-0011
- Shiriny A, Bayareh M, Ahmadi NA. Nanofluid flow in a microchannel with inclined cross-flow injection. SN Appl Sci. 2019;1:1015. doi:10.1007/s42452-019-1050-y
- Rasool G, Shah HZS, Sajid T, et al. Spectral relaxation methodology for chemical and bioconvection processes for cross nanofluid flowing around an oblique cylinder with a slanted magnetic field effect. Coatings. 2022;12:1560. doi:10.3390/coatings12101560
- Oreyeni T, Ali Shah N, Popoola OA, et al. The significance of exponential space-based heat generation and variable thermophysical properties on the dynamics of casson fluid over a stratified surface with non-uniform thickness. Waves Random Complex Media. 2022;1; doi:10.1080/17455030.2022.2119304
- Priyadharshini P, Archana VM, Ahammad AN, et al. Gradient descent machine learning regression for MHD flow: metallurgy process. Int Commun Heat Mass Transfer. 2022;138:106307. doi:10.1016/j.icheatmasstransfer.2022.106307
- Shah NA, Wakif A, Essam R, et al. Heat transfers thermodynamic activity of a second-grade ternary nanofluid flow over a vertical plate with atangana-baleanu time-fractional integral. Alexandria Eng J. 2022;61:10045–10053. doi:10.1016/j.aej.2022.03.048
- Suhail AKD, Siddiqui MA. Numerical studies on heat and fluid flow of nanofluid in a partially heated vertical annulus. Heat Transfer. 2020;49:1458–1490. doi:10.1002/htj.21672
- Ghasemi SE, Hatami M. Solar radiation effects on MHD stagnation point flow and heat transfer of a nanofluid over a stretching sheet. Case Stud Therm Eng. 2021;25:100898. doi:10.1016/j.csite.2021.100898
- Alfvén H. Existence of electromagnetic-hydrodynamic waves. Nature. 1942;150:405–406. doi:10.1038/150405d0
- Sing K, Pandey AK, Kumar M. Melting heat transfer assessment on magnetic nanofluid flow past a porous stretching cylinder. J Egypt Math Soc. 2021;29:1–14. doi:10.1186/s42787-020-00109-0
- Jha BK, Malgwi BP. Hall and ion-slip effects on MHD mixed convection flow in a vertical microchannel with asymmetric wall heating. Eng Rep. 2020;2:12241. doi:10.1002/eng2.12241
- Mohidul HM. Heat and mass transfer analysis on magneto micropolar fluid flow with heat absorption in induced magnetic field. Fluids. 2021;6:126. doi:10.3390/fluids6030126
- Alam J, Murtaza G, Tzirtzilakis E, et al. Biomagnetic fluid flow and heat transfer study of blood with gold nanoparticles over a stretching sheet in the presence of magnetic dipole. Fluids. 2021;6:113. doi:10.3390/fluids6030113
- Zeeshan A, Shehzad N, Atif M, et al. Electromagnetic flow of SWCNT/MWCNT suspensions in Two ImmiscibleWater- and engine-Oil-based newtonian fluids through porous media. Symmetry (Basel). 2022;406:1–18. doi:10.3390/sym14020406
- Shehzad N, Zeeshan A, Shakeel M, et al. Effects of magnetohydrodynamics flow on multilayer coatings of newtonian and Non-newtonian fluids through porous inclined rotating channel. Coatings. 2022;430:1–25. doi:10.3390/coatings12040430
- Majeed A, Zeeshan A, Shaheen A, et al. Hall current and viscous dissipation impact on MHD mixed convection flow towards a porous exponentially surface with its engineering applications. J Magn. 2022;27:223–231. doi:10.4283/JMAG.2022.27.2.223
- Hdhiri N, Souayeh B, Alfannakh H, et al. Natural convection study with internal heat generation on heat transfer and fluid flow within a differentially heated square cavity filled with different working fluids and porous media. BioNanoScience. 2019;9:702–722. doi:10.1007/s12668-019-00626-y
- Souayeh B, Hdhiri N. Mixed convective heat transfer and heat generation simulation in lid-driven enclosure filled with porous medium. Int J Mod Phys C. 2021;32:2150106. doi:10.1142/S0129183121501060
- Hammami F, Souayeh B, Ben-Cheikh N, et al. Computational analysis of fluid flow due to a two-sided lid driven cavity with a circular cylinder. Comput Fluids. 2017;156:317–328. doi:10.1016/j.compfluid.2017.07.017
- Rasool G, Ali Shah N, El-Zahar ER. Numerical investigation of EMHD nanofluid flows over a convectively heated Riga pattern positioned horizontally in a Darcy-Forchheimer porous medium: application of passive control strategy and generalized transfer laws. Waves Random Complex Media; doi:10.1080/17455030.2022.2074571
- Shah NA, Wakif A, El-Zahar ER, et al. Numerical simulation of a thermally enhanced EMHD flow of a heterogeneous micropolar mixture comprising (60%)-ethylene glycol (EG), (40%)-water (W), and copper oxide nanomaterials (CuO), case studies in thermal engineering. Case Stud Therm Eng. 2022;35:102046. doi:10.1016/j.csite.2022.102046
- Darcy H. Les fontaines publiques De La ville De dijon. Paris: Victor Dalmont; 1856.
- Forchheimer P. Wasserbewegung durch boden. Z Ver D Ing. 1901;45:1782–1800. doi:10.4236/wjm.2017.78019
- Pop I, Ingham DB. Mathematical and computational modeling of viscous fluids and porous media. convective heat transfer. Oxford: Pergamon; 2001.
- Vafai K. Handbook of porous media. 2nd ed New York: Taylor and Francis Group; 2005. doi:10.1201/9780415876384
- Hayat T, Aziz A, Muhammad T, et al. Effects of binary chemical reaction and Arrhenius activation energy in Darcy–Forchheimer three-dimensional flow of nanofluid subject to rotating frame. J Therm Anal Calorim. 2019;136(4):1769–1779. doi:10.1007/s10973-018-7822-6
- Othman MAWA, Muhammad T. Numerical study for Darcy-Forchheimer flow of nanofluid due to a rotating disk with binary chemical reaction and Arrhenius activation energy. Mathematics. 2019;7(10):921–933. doi:10.3390/math7100921
- Ramzan M, Abid N, Lu D, et al. Impact of melting heat transfer in the time dependent squeezing nanofluid flow containing carbon nanotubes in a Darcy-Forchheimer porous media with cattaneo-christov heat flux. Commun Theor Phys. 2020;72:085801. doi:10.1088/1572-9494/ab8a2c
- Ahmad S, Ali K, Rizwan M, et al. Heat and mass transfer attributes of copper-aluminum oxide hybrid nanoparticles flow through a porous medium. Case Stud Therm Eng. 2021;25:100932. doi:10.1016/j.csite.2021.100932
- Kareem SR, Abdulhadi MA. A study of MHD and Darcy-Forchheimer e_ects on third grade flow with cattaneo-christov heat flux. AIP Conf Proc. 2020;2292:020001. doi:10.1063/5.0030516
- Jawad M, Saeed A, Khan A, et al. MHD bioconvection Darcy-Forchheimer flow of casson nanofluid over a rotating disk with entropy optimization. Heat Transfer. 2021;50:2168–2196. doi:10.1002/htj.21973
- Sheremet AM, Pop I. Effect of local heater size and position on natural convection in a tilted nanofluid porous cavity using LTNE and Buongiorno’s models. J Mol Liq. 2018;266:19–28. doi:10.1016/j.molliq.2018.06.065
- Bhatti MM, Arain BM, Zeeshan A, et al. Swimming of gyrotactic microorganism in MHD williamson nanofluid flow between rotating circular plates embedded in porous medium: application of thermal energy storage. J Eng Storage. 2022;45:103511. doi:10.1016/j.est.2021.103511
- Wang CY. Free convection on a vertical stretching surface. Z J Appl Math Mech. 1989;69:418–420. doi:10.1002/zamm.19890691115
- Gorla RS, Sidawi I. Free convection on a vertical stretching surface with suction and blowing. Appl Sci Res. 1994;52:247–257. doi:10.1007/BF00853952
- Makinde DO, Aziz A. Boundary layer flow of a nanofluid past a stretching sheet with a convective boundary condition. Int J Therm Sci. 2011;50:1326–1332. doi:10.1016/j.ijthermalsci.2011.02.019
- Khan AW, Pop I. Boundary-layer flow of a nanofluid past a stretching sheet. Int J Heat Mass Transfer. 2010;53:2477–2483. doi:10.1016/j.ijheatmasstransfer.2010.01.032
- Kuznetsov VA, Nield AD. Natural convective boundary-layer flow of a nanofluid past a vertical plate. Int J Thermal Sci. 2009;07:015. doi:10.1016/j.ijthermalsci.2011.01.003
- Nield AD, Kuznetsov VA. The Cheng-Minkowycz problem for natural convective boundary layer flow in a porous medium saturated by a nanofluid. Int J Heat Mass Transfer. 2009;52:5792–5795. doi:10.1016/j.ijheatmasstransfer.2009.07.024
- Incropera PF, DeWitt PD. Introduction to heat transfer. 3rd ed. New York: Wiley; 1996. p. 453.