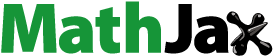
Abstract
The significant biological activity of phenylthiourea derivatives prompted the synthesis of a new phenylthiourea-based heterocyclic ring systems (pyrazole, thiazole, and pyran), and their chemical structures were elucidated by spectral data. The DFT/B3LYP methodology was applied to explore the configuration and energetic features of the FMO’s of the compounds. The derivatives exhibited comparable energy gap, ranging from 2.13 to 2.56 eV, and were arranged in the following order: 5 < 8 < 7 < 6 < 4b < 4a. The antitumor activity of the new phenylthiourea-based derivatives was investigated against diverse cell lines, such as HepG2, HCT-116, MCF-7, PC3, and WI38, using Doxorubicin as a reference drug. The hybrids 5, 6, and 8 revealed strong cytotoxic against HCT-116 (IC50 = 2.29 ± 0.46-9.71 ± 0.34 µM). Furthermore, the molecular docking studies of the compounds indicated that the hybrids 5, 6 and 8 exhibited the greatest docking score values, in accordance to the antitumor activity.
1. Introduction
Due to its ability to form stable hydrogen bonds with biological targets that can have antiviral, anticonvulsant, anti-inflammatory, antibacterial, and anti-tumor actions, thiourea activity is crucial in medicinal chemistry. In fact, the stabilization of ligand–receptor connections and the identification of bioactive sites are made possible by hydrogen bond networks; one such example is shown in Figure [Citation1–5]. Meanwhile, thiourea derivatives with thiazole, pyrazole, and pyran heterocyclic moieties have attracted a lot of interest in the field of medicinal chemistry, as evidenced by numerous works [Citation6–12]. These substances have a wide range of pharmacological properties and have a great deal of potential for use in the drug development process [Citation13,Citation14]. Five-membered heterocycles with a nitrogen and a sulfur atom make up thiazole derivatives, which have aromatic and electron-rich properties [Citation15]. With two neighboring nitrogen atoms, pyrazole compounds have strong anti-inflammatory and analgesic properties [Citation16]. Pyran derivatives, which have an oxygen-fused six-membered ring, exhibit a variety of biological activities [Citation17]. Numerous studies have been conducted on the synthesis and design of thiourea derivatives containing these heterocyclic moieties [Citation18–20]. These heterocyclic moieties’ structural adaptability and synthetic accessibility enable the development of compound libraries with a variety of chemical configurations, which facilitates a better understanding of their biological activities [Citation21]. Promising biological activity, such as anticancer, antibacterial, antiviral, anti-inflammatory, and antioxidant properties, have been shown for thiourea derivatives containing thiazole, pyrazole, and pyran heterocyclic moieties [Citation22–24]. These substances have the capacity to interact with particular biological targets, such as enzymes, receptors, and nucleic acids, resulting in their strong enzyme-related disease inhibitory activity [Citation25–27]. Thiazole, pyrazole, and pyran moieties are incorporated into the thiourea scaffold, which confers special physicochemical and structural features that affect their pharmacological profiles [Citation28,Citation29]. Their interactions with molecular targets make it possible to alter cellular functions and signaling cascades, thus increasing their therapeutic potential [Citation30,Citation31]. This research focuses on the synthesis of thiourea derivatives with thiazole, pyrazole, and pyran heterocyclic moieties as well as their molecular docking studies, anticancer properties, and DFT molecular structure studies. This manuscript aims to stimulate additional research and development on this fascinating topic by giving a thorough sense of the importance and promise of these molecules in medicinal chemistry and drug discovery.
2. Experimental
2.1. Chemistry
Melting points were established using the Gallenkamp electric apparatus. Infrared spectra (IR) were recorded (KBr discs) on a ThermoScientific Nicolet iS10 FTIR spectrometer. Nuclear magnetic resonance spectra (1H NMR: 500 MHz and 13C NMR: 125 MHz) were scanned by a Jeol 500 MHz spectrophotometer. The mass analyses were determined on a Quadrupole GC-MS (DSQII) mass spectrometer at 70 eV. The results of microanalyses (C, H, and N) were obtained using the Carlo Erba EA 1108 elemental analyzer.
2.1.1. Preparation of 1-(4-acetylphenyl)−3-phenylthiourea (2):
The title thiourea compound 2 was prepared as previously described methodology by refluxing 4-aminoacetopheone with phenyl isothiocyanate in dry toluene. Yellow solid, yield = 91%, m.p. = 145-146°C, lit. m.p. = 142-144°C [Citation32].
2.1.2. Synthesis of 1-(4-(3-(dimethylamino)acryloyl)phenyl)−3-phenylthiourea (3):
A mixture of 1-(4-acetylphenyl)−3-phenylthiourea (2) (1.62 g, 6 mmol) and N,N-dimethylformamide-dimethylacetal (0.71 mL, 6 mmol) was refluxed for four hours in 25 mL dioxane. The solid that created upon cooling to 30°C was collected and dried to produce the precursor, 1-(4-(3-(dimethylamino)acryloyl)phenyl)−3-phenylthiourea (3).
Orange solid, yield = 64%, m.p. = 186-187°C. IR (υ/cm-1): 3273, 3228 (N-H), 1673 (C = O). 1H NMR (δ/ppm): 3.11 (s, 3H), 3.20 (s, 3H) [-N(CH3)2], 5.98 (d, J = 12.50 Hz, 1H, COCH = CH-N), 7.01 (t, J = 7.50 Hz, 1H), 7.29 (t, J = 7.50 Hz, 2H), 7.52 (d, J = 8.00 Hz, 2H), 7.67 (d, J = 8.50 Hz, 2H), 7.88 (d, J = 8.50 Hz, 2H), 8.46 (d, J = 12.50 Hz, 1H, COCH = CH-N), 8.93 (s, 1H, N–H), 9.46 (s, 1H, N–H). 13C NMR (δ/ppm): 40.83, 43.47, 91.88, 117.68, 118.96 (2C), 123.07, 129.24, 130.34 (2C), 132.85, 139.15, 143.71, 156.21, 179.37, 187.52. MS m/z (%): 325 (M+, 43.09), 212 (77.30), 183 (53.13), 147 (57.11), 125 (100.00), 87 (58.76), 59 (84.60). Analysis for C18H19N3OS (325.12): Calcd: C, 66.43; H, 5.89; N, 12.91%. Found: C, 66.56; H, 5.84; N, 12.98%.
2.1.3. Synthesis of 1-(4-(1H-pyrazol-5-yl)phenyl)−3-phenylthiourea compounds 4a and 4b:
To a solution of enaminone compound 3 (0.65 g, 2 mmol) in ethanol (25 mL), an equimolar amount of hydrazine hydrate (0.15 mL, 3 mmol) and/or phenyl hydrazine (0.21 mL, 2 mmol) was added. The mixture was refluxed in the presence of one drop of triethylamine for six hours. The solid that formed upon cooling was filtered and dried to afford the conforming pyrazolyl-phenylthiourea compounds 4a and 4b, respectively.
1-(4-(1H-Pyrazol-5-yl)phenyl)−3-phenylthiourea (4a):
Orange solid, yield = 82%, m.p. = 262-263°C. IR (υ/cm-1): 3304, 3267, 3219 (N-H), 1636 (C = N). 1H NMR (δ/ppm): 6.58 (d, J = 5.50 Hz, 1H, pyrazole-H), 7.03 (t, J = 7.50 Hz, 1H), 7.30 (t, J = 7.50 Hz, 2H), 7.47 (d, J = 8.00 Hz, 2H), 7.56 (d, J = 5.50 Hz, 1H, pyrazole-H), 7.70 (d, J = 8.50 Hz, 2H), 7.91 (d, J = 8.50 Hz, 2H), 8.87 (s, 1H, N–H), 9.31 (s, 1H, N–H), 11.94 (s, 1H, N–H). 13C NMR (δ/ppm): 104.63, 118.82, 121.08 (2C), 123.43, 125.38 (2C), 127.28, 129.13 (2C), 137.47, 138.96, 140.89, 141.75, 179.51. MS m/z (%): 294 (M+, 56.83), 281 (18.98), 273 (49.54), 230 (44.97), 209 (76.48), 162 (39.30), 138 (64.56), 109 (100.00), 98 (50.11), 65 (72.31). Analysis for C16H14N4S (294.09): Calcd: C, 65.28; H, 4.79; N, 19.03%. Found: C, 65.13; H, 4.85; N, 19.14%.
1-Phenyl-3-(4-(1-phenyl-1H-pyrazol-5-yl)phenyl)thiourea (4b):
Orange solid, yield = 76%, m.p. = 237-238°C. IR (υ/cm-1): 3258, 3221 (N-H), 1641 (C = N). 1H NMR (δ/ppm): 6.61 (d, J = 5.50 Hz, 1H, pyrazole-H), 7.00 (t, J = 7.50 Hz, 1H), 7.28 (t, J = 7.50 Hz, 2H), 7.48 (d, J = 8.00 Hz, 2H), 7.55 (d, J = 5.50 Hz, 1H, pyrazole-H), 7.59-7.70 (m, 7H), 7.89 (d, J = 8.50 Hz, 2H), 8.95 (s, 1H, N–H), 9.30 (s, 1H, N–H). 13C NMR (δ/ppm): 107.29, 119.05, 121.08 (2C), 123.38, 123.96, 125.53 (2C), 126.65, 127.41, 129.01, 129.40 (2C), 138.79, 139.46, 140.77, 141.33, 141.81, 179.60. MS m/z (%): 370 (M+, 31.74), 351 (49.92), 294 (100.00), 260 (26.30), 133 (37.28), 119 (52.50), 82 (50.73), 77 (65.02). Analysis for C22H18N4S (370.13): Calcd: C, 71.33; H, 4.90; N, 15.12%. Found: C, 71.15; H, 4.97; N, 15.24%.
2.1.4. Synthesis of 1-4-7H-thiazolo3,2-apyrimidin-5-yl)phenyl)−3-phenylthiourea (5):
To a solution of enaminone compound 3 (0.65 g, 2 mmol) in methanol (25 mL), an equimolar amount of 2-aminothiazole (0.20 g, 2 mmol) was added. The mixture was refluxed for six hours in methanol and sodium methoxide (0.11 g, 2 mmol). The obtained solid was collected and crystallized from ethanol to give the conforming thiazolopyrimidine-phenylthiourea compound 5.
Brown solid, yield = 57%, m.p. = 255-256°C. IR (υ/cm−1): 3263, 3230 (N-H), 1627 (C = N). 1H NMR (δ/ppm): 4.41 (d, J = 7.00 Hz, 2H, pyrimidine-CH2), 5.27 (t, J = 7.00 Hz, 1H, pyrimidine-H), 5.59 (d, J = 4.00 Hz, 1H, thiazole-H), 6.87 (d, J = 4.00 Hz, 1H, thiazole-H), 7.03 (t, J = 7.50 Hz, 1H), 7.30 (t, J = 7.50 Hz, 2H), 7.48 (d, J = 8.00 Hz, 2H), 7.69 (d, J = 8.50 Hz, 2H), 7.90 (d, J = 8.50 Hz, 2H), 9.01 (s, 1H, N–H), 9.36 (s, 1H, N–H). 13C NMR (δ/ppm): 46.07, 96.95, 98.75, 120.64, 121.33 (2C), 123.52, 125.79 (2C), 128.18, 129.07 (2C), 131.36, 138.25, 138.84, 139.42, 154.29, 179.56. MS m/z (%): 364 (M+, 27.19), 328 (25.13), 111 (100.00), 96 (36.06), 74 (65.71), 51 (50.22). Analysis for C19H16N4S2 (364.08): Calcd: C, 62.61; H, 4.42; N, 15.37%. Found: C, 62.82; H, 4.51; N, 15.24%.
2.1.5. Synthesis of phenylthiourea-based compounds 6, 7, and 8
To a solution of enaminone compound 3 (0.65 g, 2 mmol) in glacial acetic acid (25 mL), benzothiazol-2-yl acetonitrile (0.35 g, 2 mmol), acetyl acetone (0.2 mL, 2 mmol), and/or dimedone (0.28 g, 2 mmol) was added and then subjected to reflux for eight hours. The mixture was poured onto cold water (50 mL), the obtained solid was filtered and crystallized from ethanol to give the conforming phenylthiourea-based heterocyclic compounds 6, 7, and 8, respectively.
1-(4-(4-Cyano-3H-benzo[Citation4,Citation5]thiazolo[Citation3,2-a]pyridin-1-yl)phenyl)−3-phenylthiourea (6):
Reddish brown solid, yield = 66%, m.p. =283-284°C. IR (υ/cm−1): 3268, 3225 (N-H), 2221 (C≡N), 1633 (C = N). 1H NMR (δ/ppm): 4.19 (d, J = 7.00 Hz, 2H, pyridine-CH2), 6.01 (t, J = 7.00 Hz, 1H, pyridine-H), 7.05 (t, J = 7.50 Hz, 1H), 7.27 (t, J = 7.50 Hz, 2H), 7.45-7.64 (m, 8H), 7.91 (d, J = 8.50 Hz, 2H), 9.11 (s, 1H, N–H), 9.35 (s, 1H, N–H). 13C NMR (δ/ppm): 31.96, 79.48, 111.88, 116.53, 120.70 (2C), 121.43 (2C), 122.18, 122.67, 123.18, 124.27, 125.96 (2C), 126.93, 127.83, 129.05 (2C), 131.49, 138.78, 139.56, 142.01, 143.36, 151.30, 179.62. MS m/z (%): 438 (M+, 63.05), 403 (63.41), 368 (87.68), 310 (100.00), 225 (84.60), 200 (94.93), 108 (75.56), 78 (88.96). Analysis for C25H18N4S2 (438.10): Calcd: C, 68.47; H, 4.14; N, 12.78%. Found: C, 68.37; H, 4.10; N, 12.85%.
1-(4-(5-Acetyl-6-methyl-4H-pyran-2-yl)phenyl)−3-phenylthiourea (7):
Red solid, yield = 75%, m.p. = 226-227°C. IR (υ/cm−1): 3276, 3220 (N-H), 1673 (C = O). 1H NMR (δ/ppm): 2.16 (s, 3H, COCH3), 2.37 (s, 3H, CH3), 2.89 (d, J = 7.00 Hz, 2H, pyran-CH2), 5.38 (t, J = 7.00 Hz, 1H, pyran-H), 7.02 (t, J = 7.50 Hz, 1H), 7.28 (t, J = 7.50 Hz, 2H), 7.49 (d, J = 8.00 Hz, 2H), 7.70 (d, J = 8.50 Hz, 2H), 7.88 (d, J = 8.50 Hz, 2H), 9.08 (s, 1H, N–H), 9.39 (s, 1H, N–H). 13C NMR (δ/ppm): 17.08, 22.19, 25.18, 93.28, 112.44, 120.56 (2C), 121.52 (2C), 124.21, 125.81 (2C), 127.04, 128.98 (2C), 138.70, 140.13, 147.37, 156.92, 179.66, 196.30. MS m/z (%): 364 (M+, 28.94), 293 (25.06), 274 (41.81), 247 (70.64), 187 (46.23), 135 (52.45), 115 (54.14), 91 (100.00). Analysis for C21H20N2O2S (364.12): Calcd: C, 69.21; H, 5.53; N, 7.69%. Found: C, 69.34; H, 5.59; N, 7.78%.
1-(4-(7,7-Dimethyl-5-oxo-5,6,7,8-tetrahydro-4H-chromen-2-yl)phenyl)−3-phenylthiourea (8):
Red solid, yield = 71%, m.p. = 270-271°C. IR (υ/cm−1): 3270, 3223 (N-H), 1667 (C = O). 1H NMR (δ/ppm): 0.96 (s, 3H, CH3), 1.01 (s, 3H, CH3), 1.93 (s, 2H, CH2), 2.08 (s, 2H, CH2), 2.94 (d, J = 7.00 Hz, 2H, pyran-CH2), 5.33 (t, J = 7.00 Hz, 1H, pyran-H), 7.01 (t, J = 7.50 Hz, 1H), 7.27 (t, J = 7.50 Hz, 2H), 7.48 (d, J = 8.00 Hz, 2H), 7.65 (d, J = 8.50 Hz, 2H), 7.87 (d, J = 8.50 Hz, 2H), 9.06 (s, 1H, N–H), 9.29 (s, 1H, N–H). 13C NMR (δ/ppm): 21.87, 27.63, 27.85, 32.93, 38.17, 47.11, 93.50, 113.67, 120.60 (2C), 121.47 (2C), 124.15, 125.72 (2C), 126.98, 129.03 (2C), 138.83, 140.22, 147.45, 157.08, 179.58, 187.41. MS m/z (%): 404 (M+, 30.47), 360 (100), 341 (50.74), 307 (93.61), 241 (68.61), 93 (76.12), 51 (69.22). Analysis for C24H24N2O2S (404.16): Calcd: C, 71.26; H, 5.98; N, 6.93%. Found: C, 71.10; H, 5.90; N, 6.81%.
2.2. Computational calculations
The synthesized phenylthiourea derivatives geometrical optimization were performed using Gaussian 09W software [Citation33] via DFT/B3LYP/6-311++G(d,p) approach [Citation34–36]. The GaussView program has been utilized in investigation of optimized structures characteristics, electronic and FMO’s [Citation37]. The Fukui indices were determined via the Materials Studio package/DMol3 module [Citation38] at B3LYP and DNP (ver. 3.5) basis set [Citation39].
2.3. In vitro cytotoxicity evaluation
The produced phenylthiourea-based derivatives were investigated using the MTT method on human colorectal carcinoma (HCT-116), breast cancer (MCF-7), prostate cancer (PC3), liver carcinoma (HepG2), and normal fibroblast cells (WI38) in order to determine their in vitro cytotoxic effectiveness [Citation40,Citation41] For Doxorubicin (Dox), the studied hybrids 4–8 revealed respectable IC50 standards (mean ± SD, µM) [Citation42,Citation43].
2.4. Molecular docking
The optimized structure of the synthesized molecules obtained from DFT calculations were subjected to docking studies carried out using the program M.O.E. Citation2015.10. [Citation44]. In the meantime, a protein structure file (PDB ID: 2VCJ) was downloaded from the RSCB Protein Data Bank. Hydrogen was added, solvent, and co-crystal ligand were removed, and polar hydrogen was then added.
3. Results and discussion
3.1. Synthesis of phenylthiourea-based heterocycles
The title compound, 1-(4-acetylphenyl)−3-phenylthiourea (2) [Citation32] was converted into its corresponding enaminone 3 by refluxing with dimethylformamide-dimethylacetal (DMF-DMA) reagent in dioxane (Scheme 1). The reaction of enaminone compound 3 with different nitrogen binucleophiles (namely; hydrazine hydrate and phenyl hydrazine) was investigated. The reaction has been achieved by refluxing in ethanol and triethylamine to produce the conforming phenylthiourea-pyrazole compounds 4a and 4b. The structures of compounds 4a and 4b were secured based on their spectral data. The IR spectrum of pyrazole compound 4a clearly identified the absorptions of N-H functions at 3304, 3267, and 3219 cm−1 in addition to the absorption of the pyrazole-C = N function at 1636 cm−1. The 1H NMR spectrum exhibited two doublet signals at δ 6.58 and 7.56 ppm for the protons of the pyrazole ring system. The aromatic protons were detected as triplet and doublet signals in the range from δ 7.03–7.91 ppm. The protons of N-H groups were observed at δ 8.87, 9.31, and 11.94 ppm as singlet signals. In the mass analysis, the molecular ion peak was detected at m/z = 294 (56.83%) to indicate the molecular formula C16H14N4S for compound 4a.
The reaction of enaminone compound 3 with 2-aminothiazole was performed in boiling methanol and sodium methoxide to afford the corresponding thiazolopyrimidine-phenylthiourea compound 5 (Scheme 2). The reaction of enaminone compound 3 with several types of carbon-nucleophiles has also been investigated. Thus, in refluxing acetic acid, the reaction of compound 3 with benzothiazol-2-yl acetonitrile produced the corresponding benzothiazolo[Citation3,2-a]pyridine-phenylthiourea compound 6. The structures of compounds 5 and 6 find support from their agreeable elemental and spectral analyses. The IR spectrum of compound 6 displayed absorptions at 3268 and 3225 cm−1 assigned for the two N-H groups. At its expected frequency, the nitrile group was observed at 2221 cm−1. The 1H NMR spectrum showed doublet and triplet signals at δ 4.19 and 6.01 ppm assigned for protons of the pyridine ring system ( = CH-CH2-). The aromatic protons were observed as triplet, multiplet, and doublet signals (δ 7.05–7.91 ppm). The two singlet signals at δ 9.11 and 9.35 ppm were assigned to the protons of two N-H groups.
Scheme 2. Synthesis of phenylthiourea linked with thiazolopyrimidine and thiazolopyridine compounds 5 and 6.

The enaminone compound 3 was reacted with acetyl acetone in glacial acetic acid to produce the corresponding 1-(4-(5-acetyl-6-methyl-4H-pyran-2-yl)phenyl)−3-phenylthiourea-based pyran compound 7 (Scheme 3). Finally, the targeting tetrahydrochromene-phenylthiourea compound 8 was obtained from the reaction of dimedone in glacial acetic acid with enaminone compound 3. The structures of the latter products (7 and 8) were inferred from microanalysis and spectral data. The IR spectrum of phenylthiourea-pyran compound 7 indicated the absorption frequencies of the two N-H groups at 3276 and 3220 cm−1 while the absorption of the carbonyl function was recorded at 1673 cm−1. The 1H NMR spectrum exhibited the characteristic singlet signals to the protons of methyl groups at δ 2.16 (-COCH3) and 2.37 ppm (pyran-CH3). The doublet and triplet signals that were recorded at δ 2.89 and 5.38 ppm were assigned for the protons of the pyran ring system ( = CH-CH2-). The aromatic protons appeared as triplet and doublet signals (δ 7.02–7.88 ppm). The N-H protons resonated at δ 9.08 and 9.39 ppm.
3.2. Molecular modeling
The DFT geometrical optimization of the investigated phenyl thiourea derivatives 4–8 afforded very similar V-shape structures in which the phenyl thiourea moiety has almost identical configuration. For example, the terminal phenyl ring (Ph1) was slanted on the thione group plane by 26.0°, as the dihedral angles CS(thr)-N1(thr)-C1(Ph1)-C2(Ph1) and SC(thr)-CS(thr)-N1(thr)-C1(Ph1) were 22.7-27.5° and 153.0-154.5° ranges, respectively (Figure ). Similarly, the other phenyl thiourea ring (Ph2) behaved in the same manner where the corresponding dihedral angles, CS(thr)-N2(thr)-C1(Ph2)-C2(Ph2) and SC(thr)-CS(thr)-N2(thr)-C1(Ph2), displayed values of 156.1-159.7° and 152.3-154.5°, respectively (Tables S1). On contrary, the pyrazole derivatives 4a-b dihedral angle designated that the pyrazole ring was planar and sloped on the adjacent phenyl ring where the C3(Ph2)-C4(Ph2)-C5(Pyz)-N1(Pyz) was 23.8° and 38.2°, respectively, as well as the phenyl pyrazole substituent was situated out the pyrazole plane as C4(Ph2)-C5(Pyz)-N1(Pyz)-C1(PhPyz) = 10.4° and C5(Pyz)-N1(Pyz)-C1(PhPyz)-C2(PhPyz) = 34.8°. Both of thiazolopyrimidine 5 and thiazolopyridine 6 derivatives exhibited more deviation from planarity where the pyrimidine and pyridine substituents were tilted on the phenyl ring as, e.g. the dihedral angle C3(Ph2)-C4(Ph2)-C5(TzPym)-C6(TzPym) = −45.4° and C3(Ph2)-C4(Ph2)-C1(TzPyn)-N10(TzPyn) = 42.5°, respectively. Moreover, the pyranyl nucleus along with its methyl and acetyl substituents in 7 has planar structure and was approximately in plane with the phenyl nucleus where C3(Ph2)-C4(Ph2)-C2(Pyrn)-O1(Pyrn) = −2.1° and C4(Pyrn)-C5(Pyrn)-CO(Act)-OC(Act) = 179.9°. Also, the chromenyl nucleus has a bent configuration in which the C7-atom is shifted down the plane of the other atoms, where O(Oxo)-C5(Chrm)-C6(Chrm)-C7(Chrm) = −150.8°, while the whole chromenyl moiety is tilted on the phenyl ring as C3(Ph2)-C4(Ph2)-C2(Chrm)-O1(Chrm) =3.8° (Figure ) (Tables S1). Moreover, the other structural parameters, length and angle of bonds, offered remarkable resemblance with those gained from the single crystal x-ray of correspondent compounds [Citation45–47], where the realized lengths have been lengthier than the equivalent x-ray by a maximum of 0.13 Å, RMSD = 0.04, while the angle deviations reached a maximum of 14.8°, RMSD = 3.3-5.4. Such divergences may be caused by, in calculations, only one gaseous molecule was studied and thus no intermolecular columbic interactions were observed, whereas in solid crystal lattice, the molecules interacted with each other [Citation48] (Tables S2-S3).
The HOMO and LUMO exhibited significant role in molecule’s ability for electrons donation and acceptance [Citation49] along with the bioactivity which influenced mainly by the HOMO–LUMO energy gap [Citation50–52]. The 3D graphic representation of FMO, depicted in Figure , revealed that all the studied derivatives have similar LUMO configuration in which it was mainly made up of the entire molecule π*-orbitals. Whereas, the HOMO plots disclosed altered configurations. The 4a-b derivatives have similar HOMO structure that were constructed principally from the non-bonding electrons of the thiourea sulfur atom along with the π-orbitals of the whole molecule, while in 5 and 6 derivatives, it was formed from the π-orbital of thiazolopyrimidine and thiazoloptyridine fragments, respectively, with minimal involvement of the heteroatoms lone pair of electrons. Furthermore, the HOMO plots of derivatives 7 and 8 presented close configuration in which it was built of the thiourea sulfur atom non-bonding electrons with participation of the π-orbitals the phenyl thiourea rings along with minor involvement of the pyranyl and chromenyl nucleus, respectively. Table presents the energy of HOMO (EH) and LUMO (EL) where the data cleared that the explored derivatives have comparable values of EH, −5.29 to −5.68 eV, while the EL values were less diverse, −3.09 to −3.31 eV. Although the compounds 5 and 4b presented the lowest values of EH and EL, respectively, the studied hybrids exhibited small and comparable energy gap (ΔEH-L), 2.13-2.56 eV, and may be arranged as 5 < 8 < 7 < 6 < 4b < 4a. Correspondingly, exploiting the obtained energies, several chemical reactivity parameters have been calculated, i.e. electronegativity (χ), global hardness (η), softness (δ), electrophilicity (ω), electron-donating power (ω-) and electron-accepting power (ω+) as follows [Citation50].
As displayed in Table , the derivative 5 has the smallest value of electronegativity (χ), global hardness (η) and highest softness (δ), thus it would be utmost chemically reactive, least kinetically stable and softest derivative. The electrophilicity index (ω), as a measure of stabilization energy owing to receiving extra electronic charge from the environment, were calculated, 7.48-8.62 eV, and so they were strong electrophile as their ω > 1.5 eV [Citation53,Citation54], according to the order 4b < 4a < 5 < 6 < 7 < 8. Similarly, the electron donating (ω+) and acceptance (ω-) powers, measure the ability to give and receive electrons, of the investigated derivatives were 9.83-10.99 and 5.45-6.53 eV, respectively, which cleared that they have higher tendency to accept than donation, as lesser values imply better transaction [Citation53,Citation54] (Table ).
Table 1. The FMO’s energies and chemical reactivity parameters of investigated compounds (eV).
The molecule’s charge transfer and electronegativity can be explained by the Mulliken’s atomic charges [Citation55] (Table S4). The Mulliken’s charges of the studied phenylthiourea compounds disclosed that the thiourea sulfur and nitrogen atoms, SC(thr), N1(thr) and N2(thr), have negative charges where the SC(thr) acquired higher charge than nitrogen’s, −0.747 – −0.779, where, the N1(thr) charge were slightly less than those of N2(thr), −0.239 – −0.291 and −0.265 – −0.357, respectively. In derivative 4a, the pyrazolyl nitrogen’s, N1(Pyz) and N2(Pyz), have been negatively charged, −0.086 and −0.288, respectively, whereas in 4b, the N1(Pyz) turned to be positive, 0.216, which may be ascribed to the participation of its lone pair of electrons in resonance of the adjacent phenyl ring. On contrary, the thiazolopyrimidine and thiazolopyridine derivatives 5 and 6 data displayed that their sulfur atoms, S1(TzPym) and S5(TzPyn), have negative charge but the former was much lower than the latter, −0.064 and −0.457, respectively. However, their nitrogen atoms have positive values, where in 5, the N4(TzPym) and N8(TzPym) were lower in charge, 0.402 and 0.163, respectively, than the N10(TzPyn) in 6, 0.669. On the other hand, the oxygen atoms of pyranyl and chromenyl nucleus have negative charge, −0.127 and −0.142, and so their acetyl and oxo substituents with higher values, −0.327 and −0.309, respectively (Table S4).
Additionally, the Fukui’s indices were determined to differentiate the susceptible sites for nucleophilic () and electrophilic (
) attacks [Citation56–59]. However, Fukui's indices occasionally revealed inaccurate nucleophilic and electrophilic attack active sites, therefore, the local relative electrophilicity (
) and nucleophilicity (
) descriptors were calculated and compared with the corresponding Fukui's indices [Citation60–62], where δ is global softness,
and
. The electrophilic attack indices (
) data of all derivatives showed that the thiourea sulfur, SC(thr), was the most susceptible site in all derivatives except in 5 where it appeared in the second place after the sulfur atom of thiazolopyrimidine, S1(TzPym). On contrary, the relative electrophilicity descriptors data,
, presented entirely changed order than that found in Fukui’s indices where the thiourea sulfur atom, SC(thr), occupied the first position in 4a and 7 derivatives while it appeared in the 3rd and 2nd positions in 4b and 8, respectively (Table S5). Also, the Fukui’s indices (
) indicated that the thiourea sulfur atom, SC(thr), was the utmost liable site in all derivatives while its carbon atom, CS(thr), occupied the 2nd place in both 4b and 5, and the 3rd in 4a and 6. In contrast, the relative nucleophilicity descriptors data,
, offered diverse patterns for the highly vulnerable atoms. Such as the phenyl carbon, C1(Ph1), was the largely active in 4b and 5 whereas it resided in the 2nd position after pyrazole and phenyl carbon atoms, C5(Pyz) and C4(Ph2), in both of 4a and 6, respectively (Table S5).
Moreover, the molecular polarizability (αtotal), hyperpolarizabilities (βtotal), and dipole moment (μ), were calculated [Citation63–65] as a measure for the molecule’s softness and electron density distribution that chiefly influence the intermolecular interactions [Citation66], as well as, optical nonlinearity and response [Citation67–70].
.
The dipole moments (μ) of the examined derivatives were varied from 1.57 D, for 5, to 5.65 D, for 7, which higher than the dipole of urea as reference material [Citation71] by 1.14-4.11 times (Table ). Additionally, the explored hybrids polarizability (αtotal) presented comparable values, as 4a and 6 displayed the lowest and highest values, 2.08 × 10−23 and 3.24 × 10−23 esu, respectively. However, their first-order hyperpolarizability revealed that the lowest and highest value was observed for the compounds 5 and 6, βtotal = 0.87 and 3.19 × 10−30 esu, respectively. Comparing with the urea’s value [Citation71], the studied hybrids presented greater hyperpolarizability than urea by 2.33-8.54 times and may be ordered as 5 < 4a < 4b < 7 < 8 < 6 (Table ).
Table 2. The computed dipole moment (μ), polarizability (αtotal), polarizability anisotropy (Δα) and first-order hyperpolarizability (βtotal) of investigated compounds.
3.3. In vitro anticancer activity
Using the MTT methodology, the in vitro cytotoxicity of the synthesized phenylthiourea-based pyrazole, thiazole, and/or pyran compounds was evaluated against different cancer cell lines, HepG2, HCT-116, MCF-7, PC3, and WI38 [Citation72]. Table displays the cytotoxic results as IC50 values (μM) using Doxorubicin (Dox) as a reference drug. In general, the results demonstrated that the produced phenylthiourea-based compounds exhibited reasonable cytotoxic efficiency against the examined cell lines. The reactivity of the synthesized phenylthiourea-based compounds on the examined cell lines is as follows: HCT-116 > HepG2 > MCF-7 >PC3. In the HCT-116 cell line, the phenylthiourea-based derivatives 5, 6, and 8 that contain thiazolopyrimidine, benzothiazolyl-pyridine, and substituted tetrahydrochromene moieties, respectively, presented potent cytotoxic effectiveness (IC50 = 2.29 ± 0.46, 9.71 ± 0.34, and 7.36 ± 0.25 μM). The reference drug displayed an IC50 of 2.42 ± 0.02 μM against HCT-116 cell line. Meanwhile, the phenylthiourea-based pyran derivative 7, which has an acetyl group in position-5, showed appropriate effectiveness (IC50 = 12.41 ± 0.08 μM). But the phenylthiourea-based pyrazole derivative 4a and its corresponding phenyl pyrazole derivative 4b offered weakest effectiveness through IC50 (20.19 ± 0.03 and 17.85 ± 0.15 μM), respectively. Also, the compounds 5 and 8 disclosed appropriate effectiveness against the HepG2 cell line (IC50 = 11.52 ± 0.48 and 14.09 ± 0.73 μM, individually) in comparison to Dox (IC50 = 4.02 ± 0.33 μM). Furthermore, the phenylthiourea-based hybrids 5, 6, and 8 revealed good effectiveness against the normal cell line WI38 (IC50 = 57.26 ± 0.65, 48.26 ± 0.17, and 52.16 ± 0.39 μM, respectively).
Table 3. In vitro cytotoxic effectiveness of phenylthiourea-based hybrids.
Inspired by the Structural-activity relationship (SAR), the cytotoxic activity of phenylthiourea-based compounds with pyrazole, thiazole, and pyran derivatives [Citation6,Citation73,Citation74]. The investigation of the structure–activity relationship (SAR) identifies the critical elements that influence their cytotoxicity. Alkyl, phenyl, or halogen group substitutions on the phenylthiourea core affect the compound's lipophilicity and interactions with target biomolecules [Citation75–77]. Another important factor is the heterocyclic ring system's makeup. N-alkylpyrazole has a weak cytotoxic activity in pyrazole derivatives, supporting the lowest IC50 values for both 4a and 4b derivatives [Citation78]. Meanwhile, thiazole hybrids such thiazolopyrimidines exhibit greater cytotoxic potency [Citation79] that can explain the eminent activities for derivatives 5 and 6 with IC50 values = 2.29 ± 0.46 and 9.71 ± 0.34 μM. Moreover, pyran derivatives with a lipophilic electron donating group at C2 also display increased cytotoxic activity [Citation80] that can be appeared in derivative 8 with IC50 value = 7.36 ± 0.25 μM. Moreover, the existence of electron with drawing group that was appeared on the pyran ring in the phenylthiourea-based hybrid 7 may be that causes the decreasing of the cytotoxic effectiveness that appeared against most of the investigated cell lines.
Ultimately, the Multiple Linear Regression methodology (MLR) [Citation81], built-in OriginPro program [Citation82], was employed to explore the relationship between the anticancer activity (IC50), as dependent variable, and the quantum chemical calculation parameters (EH, EL, ΔEH-L and δ), as independent variables (Table ). The data presented that the HOMO–LUMO gap and softness were the most effective parameters as their coefficients have negative sign with exponential values 103 which denotes that the increase in such parameters will lead to decrease in the activity except in the case of WI38 cell line where they have positive sign. Moreover, the cell lines showed good regression coefficients, R2 = 0.9342-0.9891, with standard deviation, SD = 1.62-4.96.
Table 4. The MLR coefficient of quantum chemical descriptors.
3.4. Molecular docking
In spired the important role of PDB (ID:2VCJ), where PDB: 2VCJ is one of a remarkable representative for Heat shock protein 90 (Hsp90) whose, inhibitors that showed a promising activity that bind to the N-terminal ATP binding pocket to disrupt the recurrence of chaperone protein in cancer treatment [Citation83]. The synthesized phenylthiourea-based hybrids were screened against the chosen protein by applying the docking technique. As shown in Table , the H-bonds bindings were suggested between all derivatives and amino acid remains. Also, there were residues Leu 107, Lys 58, Gly 97, Gly 137, Asp 102, Asp 93, Lys 58, Asn 51, and Asp 54 around the active pocket [Citation84]. Among the six phenylthiourea-based hybrids, the hybrids 5, 6 and 8 exhibited the greatest docking score (S = −7.5343, −7.3079 and −7.1617 kcal/mol, respectively) which are comparable to that showed for the Doxorubicin, reference drug (S = −7.3183 kcal/mol). The binding results may be attributed to the formation different types of H-bonds (donor and/or acceptor), where, for example, the nitrogen atoms N16 and N19 in addition to the S18 of the thiourea moiety of hybrid 5 were involved in acceptor H-bond formation with Gly 97 and Lys 58 residues, respectively. Meanwhile, in hybrid 6, the H-donor formation was observed between the thiourea N23 and S22 with Asp 39 and Gly 137 residues, respectively (Figure ).
Table 5. Docking outcomes of the prepared phenylthiourea-based compounds.
On contrary, the phenylthiourea-based pyrazole hybrids 4a and 4b and phenylthiourea pyran-based compounds hybrid 7 displayed the lowest binding energy values (S = −6.5585, −6.7287, and −7.0442 kcal/mol, respectively) which resulted from different types of interactions (H-donor, H-acceptor, and Pi-cation). For example, in hybrid 4a, the two nitrogen atoms N9 and N16 were participated in H-donors binding with Leu 107 while the terminal phenyl ring involved in Pi-cation interaction with Lys 58 with bond lengths 3.43, 3.48 and 3.99 Å, respectively (Figure S1). Meanwhile in hybrid 4b, there is only one H-donor was arisen between thiourea N21 and Asp 102 residue (3.10 Å) whereas the thiourea N15 and S17 were attached with Gly 97 and Lys 58 residues through bond lengths 2.97 and 3.27 Å, respectively, as it was appeared in the phenylthiourea-based pyran 7 (Figure S2 and Figure S3). Moreover, Doxorubicin was displayed acceptable binding (S = −7.3183 kcal/mol), where the tetracyclic ring C14 and hydroxyl O33 bonded with Asp 54 through two H-donors, while the carbonyl O29 formed H-acceptor with Lys 59 (Figure S4).
4. Conclusion
The main target of our research involves the synthesis of some new pyrazole, thiazole, and/or pyran ring systems linked with phenylthiourea moiety. The synthetic strategy is based on the reaction of 1-(4-(3-(dimethylamino)acryloyl)phenyl)−3-phenylthiourea with different types of nitrogen and/or carbon nucleophiles as hydrazine, phenyl hydrazine, 2-aminothiazole, 2-benzothiazolyl acetonitrile, acetyl acetone and/or dimedone. The chemical structures are secured by the spectral analyses as IR, 1H NMR, 13C NMR and mass analysis. The DFT/B3LYP investigations illustrated the FMO’s composition which characterized by low HOMO–LUMO energy gap (ΔEH-L). The MLR correlation of the DFT calculated parameters with the anticancer activity indicated that the ΔEH-L and softness were the most effective parameters and exhibited good regression coefficients, R2 = 0.9342-0.9891, and standard deviation, SD = 1.62-4.96. In order to compare the newly created phenylthiourea-based pyrazole, thiazole, and/or pyran derivatives to the standard reference doxorubicin, they were tested for their anticancer activity against a variety of cell lines, as well as HepG2, HCT-116, MCF-7, PC3, and WI38. When used on HCT-116, the synthesized hybrids 5, 6, and 8 shown substantial cytotoxic effectiveness over IC50 (2.29 ± 0.46, 9.71 ± 0.34, and 7.36 ± 0.25 µM, respectively). As the synthesized hybrids based on phenylthiourea were displayed proper anticancer effectiveness, the molecular docking stimulation was carried out by choosing a PDB: 2VCJ regarding to their interesting role in the heat shock protein 90 (Hsp90) inhibitors which revealed a good reactivity from their binding with the N-terminal ATP binding pocket to disturb the reappearance of chaperone protein in cancer therapy. The synthesized hybrids based on phenylthiourea were simulated against the chosen protein over the molecular docking process. The docking results showed proper bindings over a reasonable scores that recommended a future work for a biochemical assay on the protein 90 (Hsp90).
Competing interests
The authors declare that they have no competing interests.
Availability of data and materials
All relevant data are within the manuscript and available from the corresponding author upon request.
Supplemental Material
Download MS Word (6.8 MB)Disclosure statement
No potential conflict of interest was reported by the author(s).
References
- Özgeriş B. Synthesis of substituted phenethylamine-based thioureas and their antimicrobial and antioxidant properties. Russ J Org Chem. 2021;57:422–429. doi:10.1134/S1070428021030143
- Ravichandran V, Shalini S, Kumar KS, et al. Design, synthesis and evaluation of thiourea derivatives as antimicrobial and antiviral agents. Lett Drug Des Discov. 2019;16(6):618–624. doi:10.2174/1570180815666180801120440
- Keri RS, Budagumpi S, Balappa Somappa S. Synthetic and natural coumarins as potent anticonvulsant agents: A review with structure–activity relationship. J Clin Pharm Therap. 2022;47(7):915–931. doi:10.1111/jcpt.13644
- Calixto SD, Simão TLBV, Palmeira-Mello MV, et al. Antimycobacterial and anti-inflammatory activities of thiourea derivatives focusing on treatment approaches for severe pulmonary tuberculosis. Bioorg Med Chem. 2022;53:116506. doi:10.1016/j.bmc.2021.116506
- Elmorsy MR, Abdel-Latif E, Gaffer HE, et al. Theoretical studies, anticancer activity, and photovoltaic performance of newly synthesized carbazole-based dyes. J Mol Struct. 2022;1255:132404. doi:10.1016/j.molstruc.2022.132404
- Havrylyuk D, Roman O, Lesyk R. Synthetic approaches, structure activity relationship and biological applications for pharmacologically attractive pyrazole/pyrazoline–thiazolidine-based hybrids. Eur J Med.Chem. 2016;113:145–166. doi:10.1016/j.ejmech.2016.02.030
- Gümüş M, Yakan M, Koca İ. Recent advances of thiazole hybrids in biological applications. Future Med Chem. 2019;11(16):1979–1998. doi:10.4155/fmc-2018-0196
- Becerra D, Abonia R, Castillo J-C. Recent applications of the multicomponent synthesis for bioactive pyrazole derivatives. Molecules. 2022;27(15):4723. doi:10.3390/molecules27154723
- Mohareb RM, Al-Omran F, Azzam RA. Heterocyclic ring extension of estrone: synthesis and cytotoxicity of fused pyran, pyrimidine and thiazole derivatives. Steroids. 2014;84:46–56. doi:10.1016/j.steroids.2014.03.012
- Pasricha S, Mittal K, Gahlot P, et al. Multicomponent synthetic strategies and perspectives for synthesis of linked or fused coumarin heterocycles. J Iran Chem Soc. 2022;19(10):4035–4092. doi:10.1007/s13738-022-02603-x
- Elmorsy MR, Mahmoud SE, Fadda AA, et al. Synthesis, biological evaluation and molecular docking of new triphenylamine-linked pyridine, thiazole and pyrazole analogues as anticancer agents. BMC Chem. 2022;16(1):88. doi:10.1186/s13065-022-00879-x
- Elmorsy MR, Eltoukhi M, Fadda AA, et al. Synthesis of New carbazole–thiazole analogues and evaluating their anticancer activity. Polycycl Arom Compds. 2022: 1–17. doi:10.1080/10406638.2022.2144909
- Borah B, Chowhan LR. Ultrasound-assisted transition-metal-free catalysis: a sustainable route towards the synthesis of bioactive heterocycles. RSC Adv. 2022;12(22):14022–14051. doi:10.1039/D2RA02063G
- Karrouchi K, Radi S, Ramli Y, et al. Synthesis and pharmacological activities of pyrazole derivatives: A review. Molecules. 2018;23(1):134. doi:10.3390/molecules23010134
- Mak JY, Xu W, Fairlie DP. Thiazoles in peptides and peptidomimetics. Top Heterocycl Chem. 2017;48:235–266. doi:10.1007/7081_2015_176
- Bennani FE, Doudach L, Cherrah Y, et al. Overview of recent developments of pyrazole derivatives as an anticancer agent in different cell line. Bioorg Chem. 2020;97:103470. doi:10.1016/j.bioorg.2019.103470
- Yahiaoui AA, Ghichi N, Hannachi D, et al. Synthesis, XRD/HSA-interactions, biological activity, optical and nonlinear optical responses studies of new pyran derivative. J Mol Struct. 2022;1263:133161. doi:10.1016/j.molstruc.2022.133161
- Zahra U, Saeed A, Fattah TA, et al. Recent trends in chemistry, structure, and various applications of 1-acyl-3-substituted thioureas: a detailed review. RSC Adv. 2022;12(20):12710–12745. doi:10.1039/D2RA01781D
- Keri RS, Patil MR, Patil SA, et al. A comprehensive review in current developments of benzothiazole-based molecules in medicinal chemistry. Eur J Med Chem. 2015;89:207–251. doi:10.1016/j.ejmech.2014.10.059
- Bansal R, Malhotra A. Therapeutic progression of quinazolines as targeted chemotherapeutic agents. Eur J Med Chem. 2021;211:113016. doi:10.1016/j.ejmech.2020.113016
- Guillemard L, Kaplaneris N, Ackermann L, et al. Late-stage C–H functionalization offers new opportunities in drug discovery. Nature Rev Chem. 2021;5(8):522–545. doi:10.1038/s41570-021-00300-6
- Mustafa G, Zia-ur-Rehman M, Sumrra SH, et al. A critical review on recent trends on pharmacological applications of pyrazolone endowed derivatives. J Mol Struct. 2022;1262:133044. doi:10.1016/j.molstruc.2022.133044
- Mishra I, Mishra R, Mujwar S, et al. A retrospect on antimicrobial potential of thiazole scaffold. J Heterocycl Chem. 2020;57(6):2304–2329. doi:10.1002/jhet.3970
- Shukla PK, Verma A, Mishra P. Significance of nitrogen heterocyclic nuclei in the search of pharmacological active compounds. In: Shukla RP, Mishra RS, Tripathi AD, et al., editors. New Persp. Agric. Hum. Health. New Delhi: Bharti Publication; 2017. p. 100–126.
- Scotti L, Mendonca Junior F, Ishiki H, et al. Docking studies for multi-target drugs. Curr Drug Targ. 2017;18(5):592–604. doi:10.2174/1389450116666150825111818
- Azam F, Mohamed N, Alhussen F. Molecular interaction studies of green tea catechins as multitarget drug candidates for the treatment of Parkinson’s disease: computational and structural insights. Network: Comput Neural Syst. 2015;26(3-4):97–115. doi:10.3109/0954898X.2016.1146416
- Yang CT, Devarie-Baez NO, Hamsath A, et al. S-persulfidation: chemistry, chemical biology, and significance in health and disease. Antioxid Redox Signal. 2020;33(15):1092–1114. doi:10.1089/ars.2019.7889
- Kerru N, Gummidi L, Maddila S, et al. A review on recent advances in nitrogen-containing molecules and their biological applications. Molecules. 2020;25(8):1909. doi:10.3390/molecules25081909
- Kerru N, Singh P, Koorbanally N, et al. Recent advances (2015–2016) in anticancer hybrids. Eur J Med Chem. 2017;142:179–212. doi:10.1016/j.ejmech.2017.07.033
- Haghighijoo Z, Zamani L, Moosavi F, et al. Therapeutic potential of quinazoline derivatives for Alzheimer's disease: A comprehensive review. Eur J Med Chem. 2022;227:113949. doi:10.1016/j.ejmech.2021.113949
- Aghazadeh Tabrizi M, Baraldi PG, Borea PA, et al. Medicinal chemistry, pharmacology, and potential therapeutic benefits of cannabinoid CB2receptor agonists. Chem Rev. 2016;116(2):519–560. doi:10.1021/acs.chemrev.5b00411
- Sonmez F, Sevmezler S, Atahan A, et al. Evaluation of new chalcone derivatives as polyphenol oxidase inhibitors. Bioorg Med Chem Lett. 2011;21(24):7479–7482. doi:10.1016/j.bmcl.2011.09.130
- Frisch M, Trucks G, Schlegel H, et al. Gaussian 09W. Revision A. 1 ed. Wallingford, CT, USA: Gaussian. Inc.; 2009.
- Becke AD. Density-functional thermochemistry. III. The role of exact exchange. J Chem Phys. 1993;98(7):5648–5652. doi:10.1063/1.464913
- Lee C, Yang W, Parr RG. Development of the colle-salvetti correlation-energy formula into a functional of the electron density. Phys Rev. 1988;37(2):785–789. doi:10.1103/PhysRevB.37.785
- Perdew JP, Wang Y. Pair-distribution function and its coupling-constant average for the spin-polarized electron gas. Phys Rev. 1992;46(20):12947–12954. doi:10.1103/PhysRevB.46.12947
- Dennington R, Keith T, Millam J. GaussView, version 5. Semichem Inc, Shawnee Mission, KS2009.
- BIOVIA DS. Materials Studio. Dassault Systèmes, San Diego 2017.
- Delley B. Ground-State enthalpies: evaluation of electronic structure approaches with emphasis on the density functional method. J Phys Chem. 2006;110(50):13632–9. doi:10.1021/jp0653611
- Alqahtani AM, Bayazeed AA. Synthesis and antiproliferative activity studies of new functionalized pyridine linked thiazole derivatives. Arab J Chem. 2021;14(1):102914. doi:10.1016/j.arabjc.2020.11.020
- Gerlier D, Thomasset N. Use of MTT colorimetric assay to measure cell activation. J Immunol Methods. 1986;94(1-2):57–63. doi:10.1016/0022-1759(86)90215-2
- Mor S, Khatri M, Sindhu S. Recent progress in anticancer agents incorporating pyrazole scaffold. Mini Rev Med Chem. 2022;22(1):115–163. doi:10.2174/1389557521666210325115218
- Alam MJ, Alam O, Naim MJ, et al. Recent advancement in drug design and discovery of pyrazole biomolecules as cancer and inflammation therapeutics. Molecules. 2022;27(24):8708. doi:10.3390/molecules27248708
- Zhang Y, Zhang X, Qiao L, et al. Synthesis, structures, drug-likeness, in vitro evaluation and in silico docking on novel N-benzoyl-N′-phenyl thiourea derivatives. J Mol Struct. 2019;1176:335–345. doi:10.1016/j.molstruc.2018.08.069
- Kirby IL, Pitak MB, Wilson C, et al. Electron density distribution studies as a tool to explore the behaviour of thiourea-based anion receptors. Cryst Eng Comm. 2015;17(14):2815–2826. doi:10.1039/C5CE00213C
- Bernhardt PV, Wentrup C. Structures of 4-iminopyrido[1,2-a]pyrimidines, pyrido[1,2-a]pyrimidin-4-ones, pyridopyrimidinium olates, and thiazolo[3,2-a]pyrimidine analogues. Aust J Chem. 2012;65(4):371–375. doi:10.1071/CH12040
- Inglebert SA, Kamalraja J, Sethusankar K, et al. 4-(4-Bromophenyl)-7,7-dimethyl-2-methylamino-3-nitro-7,8-dihydro-4H-chromen-5(6H)-one including an unknown solvate. Acta Crystallogr Sect Sect E: Struct Rep Online. 2014;70(5):579––5780. doi:10.1107/S1600536814007983
- Sajan D, Joseph L, Vijayan N, et al. Natural bond orbital analysis, electronic structure, non-linear properties and vibrational spectral analysis of L-histidinium bromide monohydrate: A density functional theory. Spectrochim Acta Part A: Molecular and Biomol Spectrosc. 2011;81(1):85–98. doi:10.1016/j.saa.2011.05.052
- Bulat FA, Chamorro E, Fuentealba P, et al. Condensation of frontier molecular orbital fukui functions. J Phys Chem. 2004;108(2):342–349. doi:10.1021/jp036416r
- Xavier S, Periandy S, Ramalingam S. NBO, conformational, NLO, NBO, conformational, NLO, HOMO–LUMO, NMR and electronic spectral study on 1-phenyl-1-propanol by quantum computational methods. Spectrochim Acta Part A: Molecular and Biomol Spectrosc. 2015;137:306–320. doi:10.1016/j.saa.2014.08.039
- Makhlouf MM, Radwan AS, Ghazal B. Experimental and DFT insights into molecular structure and optical properties of new chalcones as promising photosensitizers towards solar cell applications. Appl Surf Sci. 2018;452:337–351. doi:10.1016/j.apsusc.2018.05.007
- Bouchoucha A, Zaater S, Bouacida S, et al. Synthesis and characterization of new complexes of nickel (II), palladium (II) and platinum(II) with derived sulfonamide ligand: structure, DFT study, antibacterial and cytotoxicity activities. J Mol Struct. 2018;1161:345–355. doi:10.1016/j.molstruc.2018.02.057
- Afolabi SO, Semire B, Akiode OK, et al. Quantum study on the optoelectronic properties and chemical reactivity of phenoxazine-based organic photosensitizer for solar cell purposes. Theor Chem Acc. 2022;141(4):1–14. doi:10.1007/s00214-022-02882-w
- Domingo LR, Ríos-Gutiérrez M, Pérez P. Applications of the conceptual density functional theory indices to organic chemistry reactivity. Molecules. 2016;21(6):748. doi:10.3390/molecules21060748
- Bhagyasree JB, Varghese HT, Panicker CY, et al. Vibrational spectroscopic (FT-IR, FT-Raman, 1H NMR and UV) investigations and computational study of 5-nitro-2-(4-nitrobenzyl) benzoxazole. Spectrochim. Acta Part A: Molecular and Biomol Spectrosc. 2013;102:99–113. doi:10.1016/j.saa.2012.09.032
- Olasunkanmi LO, Obot IB, Ebenso EE. Adsorption and corrosion inhibition properties of N-{n-[1-R-5-(quinoxalin-6-yl)-4,5-dihydropyrazol-3-yl]phenyl}methanesulfonamides on mild steel in 1 M HCl: experimental and theoretical studies. RSC Adv. 2016;6(90):86782–86797. doi:10.1039/C6RA11373G
- El Adnani Z, Mcharfi M, Sfaira M, et al. DFT theoretical study of 7-R-3methylquinoxalin-2(1H)-thiones (RH; CH3; Cl) as corrosion inhibitors in hydrochloric acid. Corros Sci. 2013;68:223–230. doi:10.1016/j.corsci.2012.11.020
- Mi H, Xiao G, Chen X. Theoretical evaluation of corrosion inhibition performance of three antipyrine compounds. Comput Theor Chem. 2015;1072:7–14. doi:10.1016/j.comptc.2015.08.023
- Messali M, Larouj M, Lgaz H, et al. A new schiff base derivative as an effective corrosion inhibitor for mild steel in acidic media: experimental and computer simulations studies. J Mol Struct. 2018;1168:39–48. doi:10.1016/j.molstruc.2018.05.018
- Roy R, Krishnamurti S, Geerlings P, et al. Local softness and hardness based reactivity descriptors for predicting intra- and intermolecular reactivity sequences: carbonyl compounds. J Phys Chem. 1998;102(21):3746–3755. doi:10.1021/jp973450v
- Roy R, de Proft F, Geerlings P. Site of protonation in aniline and substituted anilines in the gas phase: a study via the local hard and soft acids and bases concept. J Phys Chem. 1998;102(35):7035–7040. doi:10.1021/jp9815661
- Roy RK, Pal S, Hirao K. On non-negativity of fukui function indices. J Chem Phys. 1999;110(17):8236–8245. doi:10.1063/1.478792
- Sun Y, Chen X, Sun L, et al. Nanoring structure and optical properties of Ga8As8. Chem Phys Lett. 2003;381(3-4):397–403. doi:10.1016/j.cplett.2003.09.115
- Abraham JP, Sajan D, Joe IH, et al. Molecular structure, spectroscopic studies and first-order molecular hyperpolarizabilities of p-amino acetanilide. Spectrochem Acta Part A: Mol Biomol Spectrosc. 2008;71(2):355–367. doi:10.1016/j.saa.2008.01.010
- Karamanis P, Pouchan C, Maroulis G. Structure, stability, dipole polarizability and differential polarizability in small gallium arsenide clusters from all-electronab initioand density-functional-theory calculations. Phys Rev. 2008;77(1):013201. doi:10.1103/PhysRevA.77.013201
- Aziz M, Ejaz SA, Tamam N, et al. Management of validation of HPLC method for determination of acetylsalicylic acid impurities in a new pharmaceutical product. Sci Rep. 2022;12(1):1–17. doi:10.1038/s41598-021-99269-x
- Shi Y. Particle swarm optimization: developments, applications and resources. Proceedings of the 2001 congress on evolutionary computation (IEEE Cat No 01TH8546); 2001: IEEE.
- Prasad PN, Williams DJ. Introduction to nonlinear optical effects in molecules and polymers: Wiley New York; 1991.
- Williams DJ. Organic polymeric and Non-polymeric materials with large optical nonlinearities. Angewandte Chemie International Edition in English. 1984;23(9):690–703. doi:10.1002/anie.198406901
- Khan MU, Khalid M, Shafiq I, et al. Theoretical investigation of nonlinear optical behavior for rod and T-shaped phenothiazine based D-π-A organic compounds and their derivatives. J Saudi Chem Soc. 2021;25(10):101339. doi:10.1016/j.jscs.2021.101339
- Ahmed AB, Feki H, Abid Y, et al. Structural, vibrational and theoretical studies of l-histidine bromide. J Mol Struct. 2008;888(1-3):180–186. doi:10.1016/j.molstruc.2007.11.056
- Abd El-Meguid E, Awad H, Anwar M. Synthesis of new 1,3,4-oxadiazole-benzimidazole derivatives as potential antioxidants and breast cancer inhibitors with apoptosis inducing activity. Russ J Gen Chem. 2019;89(2):348–356. doi:10.1134/S1070363219020282
- Kapoor JK, Prakash R, Kumar A, et al. Selective synthesis of 3-(α,α-dibromoacetyl)-4-hydroxy-6-methyl-2H-pyran-2-one as an excellent precursor for the synthesis of 2-substituted 4-(4-hydroxy-6-methyl-2H-2-oxopyran-3-yl)thiazoles as antimicrobial and antifungal agents. J Heterocycl Chem. 2018;55(4):899–906. doi:10.1002/jhet.3116
- Mohareb RM, Abdo NYM, Ibrahim RA, et al. Anti-proliferative, c-Met inhibitions, and PAINS evaluations of New thiophene,thiazole, coumarin, pyran, and pyridine derivatives. Anti-Cancer Agents in Medicinal Chemistry (Form. Curr Med Chem Anti-Cancer Agents. 2022;22(11):2125–2141. doi:10.2174/1871520621666211103104408
- Ribeiro GH, Costa AR, de Souza AR, et al. An overview on the anticancer activity of Ru(II)/acylthiourea complexes. Coord Chem Rev. 2023;488:215161. doi:10.1016/j.ccr.2023.215161
- Malacarne MC, Gariboldi MB, Caruso E. Bodipys in PDT: A journey through the most interesting molecules produced in the last 10 years. Int J Mol Sci. 2022;23(17):10198. doi:10.3390/ijms231710198
- War JA, Srivastava SK, Srivastava SD. Design, synthesis and molecular docking studies of some morpholine linked thiazolidinone hybrid molecules. Eur J Chem. 2016;7(3):271–279. doi:10.5155/eurjchem.7.3.271-279.1427
- Mizuhara T, Kato T, Hirai A, et al. Structure–activity relationship study of phenylpyrazole derivatives as a novel class of anti-HIV agents. Bioorg Med Chem Lett. 2013;23(16):4557–4561. doi:10.1016/j.bmcl.2013.06.026
- Nemr MT, Teleb M, AboulMagd AM, et al. Design, synthesis and chemoinformatic studies of new thiazolopyrimidine derivatives as potent anticancer agents via phosphodiesterase-5 inhibition and apoptotic inducing activity. J Mol Struct. 2023;1272:134216. doi:10.1016/j.molstruc.2022.134216
- Fouda AM, El-Nassag MA, Elhenawy AA, et al. Synthesis of 1,4-dihydropyrano[2,3-c]pyrazole derivatives and exploring molecular and cytotoxic properties based on DFT and molecular docking studies. J Mol Struct. 2022;1249:131555. doi:10.1016/j.molstruc.2021.131555
- Worachartcheewan A, Nantasenamat C, Isarankura-Na-Ayudhya C, et al. Predicting the free radical scavenging activity of curcumin derivatives. Chem. Intellig Lab Syst. 2011;109(2):207–216. doi:10.1016/j.chemolab.2011.09.010
- OriginLab. OriginPro 2018 ed: OriginLab Corporation Northampton, MA; 2018.
- Brough PA, Aherne W, Barril X, et al. 4,5-diarylisoxazole hsp90 chaperone inhibitors: potential therapeutic agents for the treatment of cancer. J Med Chem. 2008;51(2):196–218. doi:10.1021/jm701018h
- Koca I, Özgür A, Er M, et al. Design and synthesis of pyrimidinyl acyl thioureas as novel Hsp90 inhibitors in invasive ductal breast cancer and its bone metastasis. Eur J Med Chem. 2016;122:280–290. doi:10.1016/j.ejmech.2016.06.032