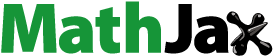
Abstract
Extracellular thermophilic multicopper oxidase i.e. laccase was purified, identified and characterized from Geobacillus stearothermophilus MB600. Fast protein liquid chromatography (FPLC) of lacasse showed a prominent peak at 610 nm. Sodium dodecyl-sulfate polyacrylamide gel electrophoresis (SDS-PAGE) for laccase presented a molecular weight of 59.13 kDa. Analysis via Matrix-Assisted Laser Desorption/Ionization Time-of-Flight (MALDI-TOF) confirmed the presence of laccase similar to in Geobacillus thermoleovorans B23 and Geobacillus sp. LEMMY01. Laccase showed Km 0.532 mM for guaiacol and 0.307 mM for ABTS (2,2′-azino-bis(3-ethylbenzothiazoline-6-sulfonic acid)). Its optimum activity was witnessed at 90°C, at pH 5.0. Laccase catalytic activity was completely inhibited by sodium dodecyl-sulfate (SDS), sodium azide (NaN3), and ethylenediaminetetraacetic acid (EDTA). Among the metal salts against Cu, Ca, Cd, and Li enhanced while Mg inhibited the enzyme activity. It was stable against Cl, I and solvents (Ethanol > Methanol > Dimethyl sulfoxide > Acetonitrile). Thermophilic laccase effectively degraded 93% of Remazol brilliant blue R, 92% Bisphenol A diglycidyle ether without mediator.
1. Introduction
Anthropogenic activities have steadily increased, industrialization and economic growth have increased, and natural resources are being exploited in the continuous hike to a predominantly urbanized civilization. This has led to enormous waste management problems, disposal issues, and the evolution of several pollutants. The degradation of these pollutants is a significant issue that the scientific community is concerned about. These contaminants must be eliminated and degraded to have a sustainable environment [Citation1]. Bioremediation offers a cutting-edge method of transforming dangerous substances into chemical derivatives that are less toxic as an alternative to standard chemical treatments [Citation2]. Bioremediation, which uses microorganisms or enzymes, can be utilized to treat pollutants by decomposing or changing them into alternative forms that are less or non-toxic to the environment. Since enzymes are more efficient at degrading pollutants than microbes are, enzyme-based bioremediation can be used to remove pollutants in a highly effective and targeted manner. Enzyme-based bioremediation has some characteristics that make it appropriate for application, such as the need for mild temperatures and reduced activation energy [Citation3].
The oxidoreductase category of enzymes produced by microorganisms includes a variety of biocatalysts that have been used to treat toxic pollutants. Laccases are one such multicopper oxidase (MCO) enzyme, with the active site comprised of three spectroscopically diverse copper (Cu) ions type-1 (T1), type-2 (T2) and type-3 (T3) [Citation4]. T1 copper present in the third domain imparts a blue colour to the enzyme because of the intense absorption spectra at 600–610 nm due to the covalent linkage of copper and cysteine. T2 “non-blue” copper does not show any spectra of absorption and is coupled with two histidine along with one water molecule. T3 copper atom is made up of a pair of copper atoms and located between the first and third domains, it has an absorption spectrum of 330 nm. T3 copper is surrounded by three histidine residues and hydroxide [Citation5]. T2 and T3 together act as trinuclear clusters where attachment and reduction of oxygen into water molecule occurs. So the active site composition of laccase at least contains one T1 copper, responsible for the substrate oxidation, one T2, and two T3 coppers, which will provide a binding site for molecular oxygen reduction [Citation6].
Laccases are identified as ecofriendly proteins/green biocatalysts in contrast to other oxidases which do not require toxic H2O2 or any mediator for the reduction reaction in contrast to other oxidases and produce water as the sole end product by reducing molecular oxygen. These are monomeric, dimeric, and tetrameric glycoproteins that can oxidize a broad spectrum of inorganic substrates, aromatic and organic compounds [Citation7]. Laccase is mostly utilized to break down chemical contaminants due to its low substrate specificity and monoelectronic oxidation of substrates in a variety of complexes. Laccase can be used effectively to degrade new contaminants, but large-scale applications require reusability, thermostability, and operational stability, necessitating techniques like immobilization and the manufacture or isolation of robust laccase with desirable features [Citation3]. Though the laccases have successfully been isolated and purified from bacteria major focus has been given to fungal laccases in the past two decades. Bacterial laccases exhibit several unique properties (in comparison with fungal laccases) which make them potential agents for industrial applications like stability at high temperatures, high pH, salt tolerance, stability against salt and organic solvents, etc. [Citation8]. These are used in various industries including food, paper, textile processing, and biofuel production [Citation9]. The first bacterial laccase was screened in 1993 from Azospirillum lipoferum from rice rhizosphere. After that laccases were detected in several bacterial species including Bacillus, Streptomyces, Klebsiella, Pseudomonas, Yersinia, Marinomonas, Proteobacterium, Bacillus subtilis, Bordetella compestris, Escherichia coli, Pseudomonas syringae, Streptomyces cyaneus, Streptomyces grizeus, Streptomyces lavendulae, Streptomyces coelicolor and also in Archaea such as Haloferax volcanii [Citation10,Citation11]. Multiple patents have been filed predominately from fungi between 2008 and 2019, which are related to various applications and obtained from multiple sources. The only thermophilic laccase isolated from Brevibacillus has been patented with the ID WO2014167562A1 and is used for the biodegradation of plastics [Citation12].
Fungal laccase’s primary drawback is that they are unstable at high temperatures (over 50°C). As a result, the investigation of thermophiles is the current focus of laccase production. The goal of the current study is to produce highly thermotolerant enzyme for commercial use by targeting thermophiles i.e. Geobacillus stearothermophilus MB600 originally isolated from hot springs of Gilgit Baltistan. It is possible to rigorously investigate the complex interactions between extraction variables and how they affect the yield or concentration of the target molecule using the single-factor experiment and response surface methodology (RSM). This strategy may result in extraction procedures that are more effective and better use microorganisms for important by products [Citation13,Citation14]. A similar approach was also used to extract laccase. This study also gives insight to understand the behaviour of thermophilic laccase under various conditions which can further play an important role in different biotechnological applications.
2. Materials and methods
2.1. Chemicals
The following chemicals: ABTS (2,2′-azino-bis(3-ethylbenzothiazoline-6-sulfonic acid)), syringaldazine (SGZ), guaiacol, Bisphenol A diglycidyl ether, and Remazol Brilliant Blue R sourced from Merck (Germany). All the chemicals were of analytical grade. Mini-PROTEAN TGX Precast Gels were purchased from BIO-RAD (USA).
2.2. Source of microorganisms and screening media
The G. stearothermophilus MB600 (GenBank Accession No.: MG720004) strain (https://www.ncbi.nlm.nih.gov/nuccore/MG720004.1/) was obtained from Microbiology and Biotechnology research lab at Fatima Jinnah Women University Rawalpindi. Bacterial culture was maintained on Nutrient agar (NA) medium and stored at −20°C. Nutrient agar supplemented with 1% syringaldazine (2, 4 dimethoxy phenol) and 0.2 mM guaiacol was used as a screening medium for primary screening of extracellular laccase production. Fresh bacterial culture (24 h) was streaked on NA plates and incubated at 80°C for 24 h [Citation15].
2.3. Extracellular laccase production and culture conditions
Minimal salt medium (MSM), which contained (g/l) CaCl2 0.01; peptone 5.0; Na2HPO4 2.4; D-glucose 10.0; NH4NO3 0.1; K2HPO4 2.0; MgSO4 0.01, and trace elements (1 ml/l) was used as laccase production medium by Kumar et al. [Citation16]. 200 ml of culture medium in a 500 ml Erlenmeyer flask was sterilized and inoculated with 24 h of fresh active culture of G. stearothermophilus (absorbance = 0.9 at 600 nm) along with guaiacol (0.5 mM) and incubated at 80°C for 72 h, under static conditions. After 72 h, the culture was harvested by centrifugation at 8000 rpm for 15 min at 4°C. The extracellular enzyme solution was used to measure laccase activity [Citation17].
2.4. Laccase assay and protein estimation
Laccase activity was measured by examining the oxidation of guaiacol. The reaction mixture consisted of 50 μl of guaiacol dissolved in 500 μl absolute ethanol and 1.5 ml of the enzyme solution in 1.5 ml 20 mM phosphate buffer (pH 5), followed by incubation at 80°C for 10 min. The oxidation of guaiacol was measured by an increase in absorbance at 470 nm against a blank. An extinction coefficient of 26,600 M−1 cm−1 was used to calculate enzyme activity by Rajeswari and Bhuvaneswari [Citation18]. One unit of enzyme was defined as the amount of enzyme that oxidized 1 μM of guaiacol per minute/ml. The protein concentration of laccase was determined by using the Bradford method [Citation19]. Bovine serum albumin was used as the standard.
where ε470 is 26,600 M−1 cm−1, AE/At is the increase in absorbance per minute, V is the volume of assay solution in the cuvette, ε is the extinction coefficient, v is the volume of the sample, t is the time in minutes.
2.5. Screening of different nutritional and environmental factors for optimal laccase production
To investigate the optimal conditions for laccase production, one-factor-at-a-time (OFAT) approach was employed on the G. stearothermophilus MB600 strain. To identify the best parameters for maximum laccase production, MSM medium was used. For routine culturing, pH 7, temperature 80°C, and 72 h incubation were used. Effect of different pHs (4–10) on enzyme production was checked by changing the pH of the medium, while the rest of the factors remained constant. Similarly, the effects of temperatures (60°C, 70°C, and 80°C), copper sulphate (0.5, 1, 1.5, 2 mM), substrate concentrations (0.1–0.9 mM), inoculum size (1–5%), and time interval (24, 48 and 72 h) was also checked. Four carbon sources (glucose, dextrose, sucrose, and sodium acetate) and three nitrogen sources (tryptone, peptone, and beef extract) at an initial concentration of 0.5% (w/v) were screened. In addition, the absorbance of liquid culture was measured and tabulated to compare bacterial cell growth and laccase production. An optical density of bacterial growth was determined spectrophotometrically at λ = 600 nm. The results were analysed and illustrated graphically by Origin pro (8.5) For every experiment, three independent experiments were set, and the reading is presented as the mean of three [Citation20].
2.6. Optimization of laccase production using central composite design
The effect of various parameters was studied to predict a precise set of environmental conditions for optimum laccase production. Process parameters affecting laccase production were investigated and validated using Response Surface Methodology (RSM). Four factors, i.e. pH, temperature, incubation period, and substrate concentration, were analysed for the optimization of enzyme production. A central composite design (CCD) with a second-order (quadratic) model was employed to assess the interaction among these significant factors (pH, temperature, substrate concentration, and incubation period) as well as to determine their optimal levels for the production of MB600 laccase. A set of 17 experiments was conducted. The lowest and maximum design factor levels for each parameter were coded. The most effective model was chosen by computing iterative F tests, lack-of-fit tests, and other accuracy measurements. The optimum levels of selected variables were obtained by solving the regression equation and analysing the response surface contours and surface plots. An analysis of variance (ANOVA, p > .05) was used to determine the statistical significance of the developed model. The coefficient of determination (R2), adjusted coefficient of determination (R2-adj) were used to assess the model’s quality. The data was analysed and graphics were created using Design-Expert 13 software [Citation21,Citation22].
2.7. Characterization of laccase
The optimal pH for laccase activity was evaluated by changing the reactions pH with the following buffer systems: for pH 4–6 (50 mM sodium acetate buffer), for pH 7–8 (10 mM sodium phosphate buffer) and for pH 9–10 (50 mM Glycine buffer). The effect of pH on the laccase stability was determined by incubating the enzyme solution with different buffers (aforementioned buffer for pH 4.0–10) at 75°C for 24 h and the relative activity was calculated as per standard method assay Rajeswari and Bhuvaneswari [Citation18]. The effect of temperature on laccase activity was determined by performing the enzyme assay at temperatures 70°C, 80°C, 90°C, 100°C, 110°C and 120°C under standard conditions (20 mM phosphate buffer, pH 6.5). The stability of the enzyme at various temperatures was investigated by preincubating the laccase at different temperatures i.e. 70°C, 80°C, 90°C, 100°C, 110°C and 120°C up to 120 min, followed by determination of the relative activity percentage [Citation20]. Similarly, the effect of metal salts including (CuSO4, CaCl2, CdSO4, MnSO4, LiCl) and inhibitors sodium dodecyl-sulfate (SDS), sodium azide (NaN3), and ethylene diaminetetraacetic acid (EDTA) at (1, 10 and 100 mM) on laccase activity was investigated by incorporating them into assay mixture prior to determination of activity [Citation23].
2.8. Laccase extraction and purification
Cell-free enzyme solution obtained after the centrifugation of 72 h incubated bacterial culture at 10,000 rpm for 20 min at 4°C was used as a source of laccase enzyme. The enzyme solution was collected and precipitated with 60% ammonium sulfate for 24 h with constant stirring at 4°C. The precipitated sample was then centrifuged at 12,000 rpm at 4°C, the enzyme solution was discarded while the pellet was collected and dissolved in 20 mM sodium phosphate buffer pH 6.4. It was finally dialysed using dialysis sacks with an average width of 25 mm and pour size of 12 kDa, against the same buffer for 24 h at 4°C. The dark brown dialysate was then concentrated by washing thrice with 20 mM sodium phosphate buffer (pH 6.4) using 30 kDa vivaspin and centrifuged at 4000 rpm for 8 min. This light brown colour protein was then subjected to a fast protein liquid chromatography FPLC AKTA purifier system. HiTrap DEAE FF 5 ml column was used for the purification of laccase [Citation24]. A 500 ml of 20 mM sodium phosphate buffer (pH 6.75–7) as a binding buffer, while 500 ml of 20 mM sodium phosphate buffer with 1 M ammonium sulphate was used as an elution buffer. For the elution buffer, pH was maintained within a range of 6.5–7. The protein of interest was collected into fraction collector units of the FPLC system. The protein samples were taken from the collection units and assayed for protein content by using Nanodrop [Citation25].
2.9. Electrophoresis
The eluted protein sample from the column was subjected to SDS-PAGE using a standard molecular mass marker of 10–180 kDa [Citation26]. SDS-Page for two groups of column fractions was performed.
2.10. In-gel tryptic digestion and MALDI-TOF MS/MS for protein identification
Two protein bands of interest were cut from SDS gel and picked in a 0.2 ml microcentrifuge tube. Trypsin digestion of excised protein was done by the method [Citation27]. The peptide mass fingerprinting was performed on a MALDI-TOF-TOF MS analyzer (ABSCIEX TOF/TOF 5800, Applied Biosystems, USA) and the protein identification (ID) was obtained using result-dependent analysis (RDA) by ProteinPilot™ software (Version 3.2, USA). The resulting peptide mass finger-printings (PMFs) were used to identify both proteins by searching the SWISS-PROT and NCBI-nr databases using the Mascot 2.0 search engine with fragment mass tolerance of ±0.3 Da [Citation28].
2.11. Kinetic parameters (Km and Vmax values) of G. stearothermophilus laccase
The kinetic constant of laccase enzyme was investigated using (ABTS) and guaiacol as a substrate, in 0.1 M sodium acetate buffer (pH 4) and 20 mM sodium phosphate buffer (pH 6.5). Km and Vmax were calculated according to the method described by Lineweaver and Burk [Citation29].
2.12. Docking and protein–protein interaction of laccase
Docking was performed between ligand (guaiacol and ABTS) and protein (laccase) using CB Dock a web server to perform the blind docking by the method of Liu et al. [Citation30]. Protein–protein interaction (PPI) of laccase was performed using STRING Data Base (11.5 version). Geobacillus genomosp. 3 was used as a model organism.
2.13. Phylogenetic analysis
Evolutionary studies were conducted in MEGA X by using the JTT model [Citation31].
2.14. Synthetic dye decolourization and endocrine disruptor degradation by G. stearothermophilus laccase
Two pollutants, i.e. Reactive blue 19 an anthraquinone dye and Bisphenol A diglycidyle ether (BADGE), were used to study their degradation by laccase. The reaction mixture containing dye solution [Remazol brilliant blue R (25 mg/1)], was incubated with 1 ml of laccase in 50 mM sodium acetate phosphate buffer (4.5) at 75°C [Citation32]. Degradation of BADGE was carried out with laccase, enzymatic treatment was carried out in a 10 ml reaction mixture containing 1 ml of enzyme solution and 9 ml of 1 M sodium acetate buffer (4.5) with a specific concentration of BADGE (10 mg/l) at 80°C [Citation33]. Per cent decolourization was checked by using T80-UV/Vis Spectrometer (PG instrument Ltd.) at the wavelength of 592 nm for RBBR and at 245 nm for BADGE as per the formula:
where I is the initial absorbance and F is the final absorbance.
3. Results
3.1. Screening of G. stearothermophilus for laccase activity
A qualitative screening of laccase was conducted. Notably, when G. stearothermophilus MB600 colonies were streaked over nutrient agar (NA) plates with syringaldazine as a supplement, noticeable visual modifications were found in the colonies. The colonies’ initial off-white colour changed into an orange, which confirmed the laccase’s presence (as seen in Figure ).
3.2. Effect of the incubation period, temperature, carbon, and nitrogen source on laccase production and biomass of G. stearothermophilus MB600
The optimum time course for the production of extracellular laccase from G. stearothermophilus MB600 was 72 h. It was observed that the incubation period showed a direct relationship between bacterial biomass and enzyme production. The optimum temperature for laccase production was 70°C. However, no increase in enzyme production was observed by increasing temperature (Figure ). For carbon sources, laccase production from thermophiles was enhanced in the presence of sucrose only while glucose and dextrose did not have any effect. However, in the case of nitrogen source, peptone and tryptone acted as enhancers but beef extract did not affect laccase production (Supplementary Table S1).
3.3. Effect of CuSO4 on laccase production and biomass
Laccase production was not noticed at 0.5 mM concentration of CuSO4 but an increase in bacterial biomass was observed. The optimum concentration for laccase production was 2 mM, however, the bacterial biomass was decreased which showed an inverse relation between CuSO4 concentration and bacterial biomass and direct relation between enzyme production and CuSO4 concentration (Supplementary Table S1).
3.4. Effect of pH, substrate concentration and inoculum size on laccase production
The enzyme production was maximum at pH 6 and in the presence of 0.5 mM substrate. The enzyme production from MB600 was optimum when the inoculum size was CFU 6 × 107 cells/ml (Figures and ).
3.5. Effect of temperature and pH on laccase activity
The effect of temperature on enzyme activity revealed that the enzyme was active at high temperature while the optimum activity was achieved at 90°C and the enzyme was stable for 60 min at 80°C. The optimum pH for laccase activity was pH 5 (Figure ).
3.6. Effect of solvents on laccase activity
The effect of four solvents was determined at different concentrations of 10%, 20% and 30% to check how these chemicals influence the enzymatic activity. G. stearothermophilus MB600 laccase retained maximum activity for ethanol while minimum activity was observed for acetonitrile. The trend followed by MB600 laccase showing maximum to minimum activity when treated with different solvents was Ethanol > Methanol > DMSO > Acetonitrile (Figure ).
3.7. Statistical optimization of laccase production
To investigate the effect of independent variables incubation period, temperature, and substrate concentration on laccase production, three-dimensional response surface plots were created (Figure ). By keeping the time period constant, the influence of temperature and pH on laccase production was evaluated, demonstrating that laccase concentration increased as the temperature climbed (80°C). However, a rise in pH at the greatest temperature did not affect laccase production. The 3D response surface plot between time duration and pH revealed that maximal enzyme production occurred with the longest treatment time and pH 7 examined. At the centre pH value, the interaction between time period and temperature exhibited maximum laccase production at the highest levels of both time and temperature (Figure ). Summary of descriptive statistics of the factors which were used for the optimization of enzyme production (Table ). The analysis of variance showed the Model F-value of 4.60 and the observed p-value was 0.04. All these values indicated that the applied model is significant (Table 2).
Figure 6. Enzyme production summary by 3D response surface plot for different independent variables i.e. actual factors X1 = A (Incubation period), X2 = B (Temperature) Actual factors C (Substrate concentration) = 0.5, D (pH) = 7.

Table 1. Central composite design (CCD) matrix.
Table 2. Analysis of variance (ANOVA) for reduced linear model.
3.8. Effect of metal salts, and halide salts and inhibitors on laccase activity
The effect of three concentrations (1, 10 and 100 mM) of metal salts on laccase activity was monitored. Copper salt played a major role in stimulating the enzyme activity at all three concentrations, whereas enzyme activity was completely inhibited by Cd2+ and Li1+. On the other hand, Ca2+ and Mn2+ did not play any role in activating the enzyme at low concentrations (1 and 10 mM) but at high concentrations (100 mM) these salts, acted as enhancers. Among halide salts only chloride and iodide showed activity, while laccase did not show any activity in the presence of Br, and F. Inhibition percentage of three inhibitors SDS, NaN3, and EDTA, was studied. All of these compounds inhibited the enzyme activity. The highest inhibition percentage was recorded for SDS at 96% (100 mM). The % inhibition trend was as follows
3.9. Protein estimation
The laccase enzyme showed 18.65 nmoles/µl protein concentration (Supplementary Figure S1).
3.10. Purification of laccase
It was observed that protein from G. stearothermophilus MB600 showed two peaks one at 280 nm wavelength and the other at 610 nm that confirmed the presence of laccase (Figure ). The protein after purification showed a protein concentration of 7.17 mg/ml. The purification yield of laccase at each step was also calculated (Table ).
Table 3. Purification of laccase from extracellular G. stearothermophilus.
Table 4. The peptide sequence of laccase identified by MALDI-TOF.
Table 5. Proteins matching the same set of peptides.
3.11. Gel electrophoresis
The molecular mass of multicopper oxidase from G. stearothermophilus MB600 was determined using SDS-PAGE and it ranged from ∼55 and 70 kD, confirming the presence of laccase once dyed with Instant blue Coomassie dye for 2 h (Figure ).
3.12. In-gel tryptic digestion and MALDI-TOF MS/MS for protein identification/phylogenetic analysis
The proteome analysis of G. stearothermophilus MB600 laccase was performed using the MALDI-TOF MS/MS system. The mascot analysis, result showed various protein entries with peptide sequences that match the protein of interest. Based on the peptide sequences that match those found in the MALDI-TOF MS/MS analysis, the protein was identified as Multicopper oxidase similar to the one found in Geobacillus thermoleovorans B23 and Geobacillus sp. LEMMY01 with a score of 174 which shows how well the theoretical data (protein sequences in the database) and actual data (peptide sequences acquired from MS/MS) matched as shown in (Tables 4 and 5). Phylogenetic analysis of the identified laccase sequence was performed by performing a Blast of this specific sequence and the top 10 closest matched sequences. The matched sequence was retrieved from Uniprot for phylogenetic analysis. The laccase identified from thermophiles was closely related to G. thermoleovorans B23 laccase (Supplementary Figure S2).
3.13. Docking
The 3D structure of laccase was predicted from Alpha Fold and the 3D structure of ABTS and guaiacol were retrieved from the Zinc database. The molecular docking was performed by CB- Dock online docking server. CB Dock performed the docking and improved the accuracy of the procedure by using a curvature-based cavity detection approach (CurPocket) for the prediction of the binding site of the target protein and the binding poses of query ligands were performed by using AutoDock Vina. For ABTS the first binding mode was selected, which showed and a Vina score of (−7.1) and cavity size of 1348. For Guaiacol the top Vina score was −5.5 and the cavity size was 263 (Figure ). Docking helped to predict the binding interaction of laccase with two of its substrates i.e. ABTS and Guaiacol. The complete 3D view of docking for both ligands is shown in Figure .
3.14. Enzyme kinetic
Laccase from G. stearothermophilus MB600 followed the Michaelis Menten equation and showed higher affinity for ABTS as compared to guaiacol with Km values of 0.3077 and 0.532 (Supplementary Figure 3).
3.15. Synthetic dye decolourization and endocrine gland disruptor degradation by G. stearothermophilus laccase
The anthraquinonic dye i.e. Ramazol brilliant blue (RBBR), and Bisphenol A diglycidyle ether (BADGE) were successfully degraded by G. stearothermophilus MB600 laccase. 93% of the decolourization percentage was observed after enzymatic treatment within 2 h of the incubation period of reaction time. BADGE when treated with laccase from G. stearothermophilus MB600, showed a 92% degradation rate after 30 min of incubation period.
4. Discussion
Laccases are very appealing macromolecules that are regarded as green catalysts [Citation34]. A wide range of biotechnological applications necessitates the development of innovative sources of highly active and stable laccases. The current study also revolved around finding a novel and highly stable laccase enzyme from thermophiles, i.e. G. stearothermophilus MB600. Laccase production as a byproduct can vary depending on parameters such as nutritional availability, pH, temperature, incubation time, and the presence of substrates that trigger laccase production for higher yield and long-term use. The operating conditions of thermophilic laccase production were optimized on low-cost media by a single factor and response surface method (RSM). The Response Surface Methodology (RSM) based on Central Composite Design (CCD) was used to evaluate and optimize the production of laccase from thermophilic bacteria. The results were in good agreement with the ones predicted by the model, thereby providing statistical authenticity and validating the model for laccase production from thermophilic bacteria.
Sucrose was the primary carbon source used by G. stearothermophilus MB600 for effective enzyme production, whereas glucose and dextrose maintained bacterial growth but did not improve enzyme yield. Peptone and tryptone enhanced thermophilic bacterial growth substantially, whereas beef extract had no effect. Notably, nitrogen supplies had a larger impact on enzyme synthesis regulation than carbon sources [Citation35]. Irpex lacteus laccase production was also boosted by peptone and malt extract. This could be because complex nitrogen sources are favoured more since they are broken down to produce nucleic acids, proteins, and amino acids, which may offer all needed nutrients to the microbial system for enzyme creation [Citation36]. Copper sulphate enhanced the enzyme production of G. stearothermophilus MB600. Even in the absence of typical aromatic mediators, copper sulphate greatly increased the production of extracellular laccase from Bacillus species (51.95 U/l). In many studies, it is used as the sole inducer and an important culture component for laccase production [Citation37]. Researchers have seen an increase in laccase synthesis by fungi when copper or different aromatic compounds are introduced to the medium [Citation38]. For laccase from G. stearothermophilus MB600, optimum laccase production was observed for a 2.5 ml (6 × 107 cells/ml) inoculum size. The optimal pH of laccase largely depends on the type of substrate used, here the enzyme was active at pH 5, 6, 7, and 8 with an optimum pH of 5, similar to the laccase from Bacillus sp. strain WT [Citation32]. Beauveria pseudobassiana laccase activity in the production medium peaked at 21.47 0.18 U/ml at pH 6.0 [Citation39], which is slightly closer to thermophilic laccase, presumably due to the organism’s predisposition for slightly acidic conditions for both laccase production and metabolism. This pH promotes three-dimensional enzyme structure and promotes nutrition absorption, organism growth, and enzyme production via signalling pathways. Notably, B. subtilis had the maximum laccase activity at pH 6.5 [Citation40], but Trichoderma harzianum needed pH 5.0 to thrive and produce laccase [Citation41]. pH 5.0 was also found to be optimum for laccase synthesis in Ganoderma leucocontextum [Citation42]. Laccase was completely inactive at pH 11, as at higher pH, ionization of critical amino acids (Asp and Glu) causes the decrease in the oxidation rate of different substrates. This might be due to the binding of the hydroxide anion to the trinuclear copper of laccase, which causes the interruption of electron transfer internally from T1 to the trinuclear centre and the ionization of pI [Citation25]. The temperature has an important environmental effect on the conformation and stability of enzymes. In general, the optimal temperature for laccase activity varies from strain to strain. The Geobacillus laccase was active at different temperatures (70°C, 80°C, 90°C, 100°C, and 110°C), with optimum activity at 90°C. A further increase in temperature decreased the enzyme activity, which might be due to protein denaturation. Similar results were reported from Bacillus tequilensis SN4 (SN4LAC) laccase [Citation43]. The stability range for most fungal laccases is 50–70°C [Citation44]. White rot fungus Trametes orientalis Laccase (Tolacc-T) also showed the highest activity at 80°C. The enzyme appears to have achieved a value of 47.11% at 100°C [Citation44]. Geobacillus laccase’s strong temperature-dependent activity could be attributed to the higher temperature environment of Pakistan, where the bacterial strain MB600 was obtained. These findings suggested that using this laccase could enable greater process temperatures, higher reaction speeds, and decreased chances of enzyme inactivation [Citation45]. So far, ABTS was mostly used as a substrate for most of the bacterial laccase but the present study reported the first thermophilic bacterial laccase where guaiacol was used as a substrate for enzyme characterization. laccase enzyme was completely inhibited by SDS and EDTA and NaN3 similar results were reported for laccase from Bacillus sp. ADR and B. subtilis LP2 [Citation46].
Laccase was stable against different organic solvents (ethanol, methanol, acetonitrile, and DMSO), halide ions, and metal ions. G. stearothermophilus laccase showed high stability in ethanol, methanol, and then acetonitrile while least stable in DMSO. Cerrena sp. HYB07, Cynaobacteria, and Ganoderma fornicatum where laccase was stable in all these organic solvents also showed the same results [Citation25,Citation47]. This may be due to the high protein conformational stiffness detected in the existence of organic solvents. Similar results were observed with solvents like DMSO, methanol, 2-propanol, acetonitrile, and acetone, the laccase activity from Streptomyces sviceus dropped to 20–40% [Citation48]. The pattern of relative activity against halide ions (100 mM) was I−1 > Cl−1, while a decrease was observed at 500 mM. The enzyme showed no activity for the rest of the halides not even at low concentrations. Zhang et al. [Citation49] reported a decrease in Bacillus vallismortis laccase activity in the presence of NaCI, retaining 41.02% and 18.61% of its original activity in the presence of 500 mM concentration of NaCI. Enzyme purification was performed by using the anion exchange chromatography technique. Diethylaminoethyl Sepharose (DEAE sepharose) column, a weak anion exchange column, where DEAE covalently binds to sepharose was used and the separation of laccase enzyme was done by using the FPLC AKTA purifier system. Sahay et al. [Citation50] conducted the purification of laccase secreted by Labarrus lividus using the same column. The thermostable laccase from Bacillus sp. had also been purified by anion exchange chromatography [Citation51]. After purification, the molecular characterization of laccase was performed by SDS-PAGE. The SDS-PAGE results showed that thermophilic laccase has a molecular weight of 59.13 kDa. Yao et al. [Citation52] reported the identification of a similar laccase with a molecular weight between 58.4 and 74.0 kDa when characterized through SDS-PAGE. MALDI-TOF database findings revealed that thermophilic laccase is a member of the multicopper oxidase protein family with a molecular weight of 59.13 kDa, which has the similar amino acid sequence of multicopper oxidase protein present in G. thermoleovorans B23 and Geobacillus sp. LEMMY01.
Enzyme kinetics study revealed that the thermophilic laccase has more affinity for ABTS as substrate as compared to guaiacol. The new blind docking tool CB dock focuses on enhancing the docking accuracy and was used to predict binding regions of a given protein i.e. laccase with its substrates (ABTS and guaiacol). CD dock used a curvature-based cavity recognition method by calculating the centers, and sizes and finally performing docking using Autodock Vina. It improved protein–ligand binding affinity prediction by using a curvature-dependent surface-area model. The Vina score is expressed in kcal/mol, and the lower the value, the stronger the anticipated binding interaction. The experimental and in silico analyses revealed the maximum activity of MB600 laccase and lowest binding energy with ABTS. Myrothecium verrucaria ITCC-8447 laccase also showed the same lowest binding energy with ABTS (−5.64), however, it showed binding energy of (−4.42) with guaiacol [Citation53]. Similarly, laccase from Stropharia sp ITCC-8422 also showed a similar result with the least binding energy for ABTS [Citation54]. A similar approach was used by Liu et al. [Citation30]. This is the very first reported study using CB dock, to perform the docking for the prediction of thermophilic laccase interaction with guaiacol and ABTS. CB-Dock’s automatic binding site identification, conformational flexibility consideration, thorough interaction modelling, solvation effects, and user-friendly workflow make it a more effective tool than traditional docking approaches. While traditional docking approaches such as AutoDock Vina have proved useful in docking research, CB-Dock’s unique capabilities can provide more accurate and nuanced insights, particularly in cases requiring precise binding interactions. Dye degradation potential of MB600 laccase revealed that it can decolorize 94% of dye in 2 h, Si et al. [Citation55] reported Ganoderma australe laccase breakdown 57.94% of RBBR dye. following a 72-h incubation period at 55°C and pH 6. Alkali-resistant and metal-tolerant laccase from Trametes pubescens showed a 66.57% dye decolourization [Citation56].
5. Conclusion
G. stearothermophilus MB600 laccase was active and stable at a narrow pH range of 5–8 with an optimum temperature of 90°C. Therefore, confirming good thermo and alkali-stable properties. In conclusion, the development of a high-temperature stable laccase isolated from thermophilic bacteria is the key contribution of our work. The novel features of the resulting enzyme have the potential to revolutionize industrial processes, improve bioprocessing efficiency, help clean up the environment, save resources, provide new biological insights, and broaden the range of biocatalysts that are available for various applications. In addition to advancing our knowledge of extremophilic enzymes, this research lays the path for creative and long-lasting solutions across a variety of industries
Supplemental Material
Download MS Word (1.8 MB)Disclosure statement
No potential conflict of interest was reported by the author(s).
References
- Bugg TD, Williamson JJ, Rashid GM. Bacterial enzymes for lignin depolymerisation: new biocatalysts for generation of renewable chemicals from biomass. Curr Opin Chem Biol. 2020;55:26–33. doi:10.1016/j.cbpa.2019.11.007
- Sutaoney P, Pandya S, Gajarlwar D, et al. Feasibility and potential of laccase-based enzyme in wastewater treatment through sustainable approach: a review. Environ Sci Pollut Res. 2022;29(57):86499–86527. doi:10.1007/s11356-022-21565-4
- Dong CD, Tiwari A, Anisha GS, et al. Laccase: a potential biocatalyst for pollutant degradation. Environ Pollut. 2023: 120999. doi:10.1016/j.envpol.2023.120999
- Reiss R, Ihssen J, Richter M, et al. Laccase versus laccase-like multi-copper oxidase: a comparative study of similar enzymes with diverse substrate spectra. PLoS One. 2013;8(6):e65633. doi:10.1371/journal.pone.0065633
- Giardina P, Faraco V, Pezzella C, et al. Laccases: a never-ending story. Cell Mol Life Sci. 2010;67(3):369–385. doi:10.1007/s00018-009-0169-1
- Santhanam N, Vivanco JM, Decker SR, et al. Expression of industrially relevant laccases: prokaryotic style. Trends Biotechnol. 2011;29(10):480–489. doi:10.1016/j.tibtech.2011.04.005
- Mehandia S, Sharma SC, Arya SK. Isolation and characterization of an alkali and thermostable laccase from a novel Alcaligenes faecalis and its application in decolorization of synthetic dyes. Biotechnol. 2020;Rep 25:e00413.
- Guan ZB, Luo Q, Wang HR, et al. Bacterial laccases: promising biological green tools for industrial applications. Cell Mol Life Sci. 2018;75(19):3569–3592. doi:10.1007/s00018-018-2883-z
- Atalah J, Caceres-Moreno P, Espina G, et al. Thermophiles and the applications of their enzymes as new biocatalysts. Bioresource Technol. 2019;280:478–488. doi:10.1016/j.biortech.2019.02.008
- Uthandi S, Saad B, Humbard MA, et al. Lcca, an archaeal laccase secreted as a highly stable glycoprotein into the extracellular medium by Haloferax volcanii. Appl Environ Microbiol. 2010;76(3):733–743. doi:10.1128/AEM.01757-09
- Liu Y, Huang L, Guo W, et al. Cloning, expression, and characterization of a thermostable and pH-stable laccase from Klebsiella pneumoniae and its application to dye decolorization. Process Biochem. 2017;53:125–134. doi:10.1016/j.procbio.2016.11.015
- Zerva A, Simic S, Topakas E, et al. Applications of microbial laccases: patent review of the past decade (2009–2019). Catalysts. 2019;9(12):1023. doi:10.3390/catal9121023
- Yan Y, Zhou H, Wu C, et al. Ultrasound-assisted aqueous two-phase extraction of synephrine, naringin, and neohesperidin from Citrus aurantium L. fruitlets. Prep Biochem Biotechnol. 2021;51(8):780–791. doi:10.1080/10826068.2020.1858427
- Jiang B, Chen P, Guo J, et al. Structural characteristics and biological activity of lactic acid bacteria exopolysaccharides separated by ethanol/(NH4) 2SO4 ATPS. Int J Biol Macromol. 2023: 125451. doi:10.1016/j.ijbiomac.2023.125451
- Bhavsar S, Dudhagara P, Tank S. R software package based statistical optimization of process components to simultaneously enhance the bacterial growth, laccase production and textile dye decolorization with cytotoxicity study. Plos One. 2018;35:e0195795.
- Kumar A, Singh AK, Ahmad S, et al. Optimization of laccase production by Bacillus sp. strain AKRC01 in presence of agro-waste as effective substrate using response surface methodology. J Pure Appl Microbiol. 2020;14(1):351–362.
- Shah AI, Jobanputra JB. Production and partial purification of laccase produced by Bacillus species isolated from contaminated soil. Int J Innov. 2017;6(9):17862–17869.
- Rajeswari M, Bhuvaneswari V. Production of extracellular laccase from the newly isolated Bacillus sp. PK4. Afr J Biotechnol. 2016;15(34):1813–1826. doi:10.5897/AJB2016.15509
- Bradford MM. A rapid and sensitive method for the quantitation of microgram quantities of protein utilizing the principle of protein dye binding. Anal Biochem. 1976;72(1-2):248–404 254. doi:10.1016/0003-2697(76)90527-3
- Ali NS, Huang F, Qin W, et al. Identification and characterization of a new Serratia proteamaculans strain that naturally produces significant amount of extracellular laccase. Front Microbiol. 2022;13:878360. doi:10.3389/fmicb.2022.878360
- Bagewadi ZK, Mulla SI, Ninnekar HZ. Optimization of laccase production and its application in delignification of biomass. Int J Recycl Org Waste Agric. 2017;6:351–365. doi:10.1007/s40093-017-0184-4
- Fariq A, Yasmin A, Jamil M. Production, characterization and antimicrobial activities of bio-pigments by Aquisalibacillus elongatus MB592, Salinicoccus sesuvii MB597, and Halomonas aquamarina MB598 isolated from Khewra salt range, 424 Pakistan. Extremophiles. 2019;23(4):435–449. doi:10.1007/s00792-019-01095-7
- Mukhopadhyay M, Banerjee R. Purification and biochemical characterization of a newly produced yellow laccase from Lentinus squarrosulus MR13. 3 Biotech. 2015;5(3):227–236. doi:10.1007/s13205-014-0219-8
- Ko EM, Leem YE, Choi H. Purification and characterization of laccase isozymes from the 406 white-rot basidiomycete Ganoderma lucidum. Appl Microbiol Biot. 2001;57(1):98–102.
- Afreen S, Shamsi TN, Baig MA, et al. A novel multicopper oxidase (laccase) from Cyanobacteria purification characterization with potential in the decolorization of anthraquinonic dye. Plos one. 2017;12:1–4. doi:10.1371/journal.pone.0175144
- Laemmli UK. Cleavage of structural proteins during the assembly of the head of bacteriophage T4. Nature. 1970;227(5259):680–685. doi:10.1038/227680a0
- Shevchenko A, Tomas H, Havli J, et al. In-gel digestion for mass spectrometric characterization of proteins and proteomes. Nat Protoc. 2016;1(6):2856–2860. doi:10.1038/nprot.2006.468
- Santhanam N, Vivanco JM, Decker SR, et al. Expression of industrially relevant laccases: prokaryotic style. Trends Biotechnol. 2011;29(10):480–489. doi:10.1016/j.tibtech.2011.04.005
- Lineweaver H, Burk D. The determination of enzyme dissociation constants. J Am Chem Soc. 1934;56(3):658–666. doi:10.1021/ja01318a036
- Liu Y, Grimm M, Dai WT, et al. CB-Dock: a web server for cavity detection-guided protein–ligand blind docking. Acta Pharmacol Sin. 2020;41(1):138–144. doi:10.1038/s41401-019-0228-6
- Kumar S, Stecher G, Li M, et al. MEGA x: molecular evolutionary genetics analysis across computing platforms. Mol Biol Evol. 2018;35(6):1547. doi:10.1093/molbev/msy096
- Siroosi M, Amoozegar MA, Khajeh K, et al. Decolorization of dyes by a novel sodium azide-resistant spore laccase from a halotolerant bacterium, Bacillus safensis sp. strain S31. Water Sci Technol. 2018;77(12):2867–2875. doi:10.2166/wst.2018.281
- Hongyan L, Zexiong Z, Shiwei X, et al. Study on transformation and degradation of bisphenol A by Trametes versicolor laccase and simulation of molecular docking. Chemosphere. 2019;224:743–750. doi:10.1016/j.chemosphere.2019.02.143
- Dwivedi UN, Singh P, Pandey VP, et al. Structure-function relationship among bacterial, fungal and plant laccases. J Mol Catal B: Enzym. 2021;68:117–128. doi:10.1016/j.molcatb.2010.11.002
- Kaira GS, Dhakar K, Pandey A. A psychrotolerant strain of Serratia marcescens (MTCC 4822) produces laccase at wide temperature and pH range. Amb Express. 2015;5(1):1–8. doi:10.1186/s13568-014-0092-1
- Rao A, Ramakrishna N, Arunachalam S, et al. Isolation, screening and optimization of laccase-producing endophytic fungi from Euphorbia milii. Arab J Sci Eng. 2019;44:51–64. doi:10.1007/s13369-018-3431-8
- Gnanamani A, Jayaprakashvel M, Arulmani M, et al. Effect of inducers and culturing processes on laccase synthesis in Phanerochaete chrysosporium NCIM 1197 and the constitutive expression of laccase isozymes. Enzyme Microb Technol. 2006;38(7):1017–1021. doi:10.1016/j.enzmictec.2006.01.004
- Kuhar F, Papinutti L. Optimization of laccase production by two strains of Ganoderma lucidum using phenolic and metallic inducers. Rev Argent Microbiol. 2014;46:144–149. doi:10.1016/S0325-7541(14)70063-X
- Sharma D, Kaur M, Borkar S, et al. Screening and identification of novel laccase producing entomopathogenic fungus Beauveria pseudobassiana PHF4 and optimization of production conditions of enzyme. Acta Sci Microbiol. 2023;6(6):2581–3226.
- Sondhi S, Saini K. Response surface-based optimization of laccase production from Bacillus sp. MSK-01 using fruit juice waste as an effective substrate. Heliyon. 2019;5:17–18. doi:10.1016/j.heliyon.2019.e01718
- Abd El Monssef RA, Hassan EA, et al. Production of laccase enzyme for their potential application to decolorize fungal pigments on aging paper and parchment. Ann Agric Sci. 2016;61(1):145–154. doi:10.1016/j.aoas.2015.11.007
- Umar A, Ahmed S. Optimization, purification and characterization of laccase from Ganoderma leucocontextum along with its phylogenetic relationship. Sci Rep. 2022;12:1–14. doi:10.1038/s41598-021-99269-x
- Zhang Y, Lv Z, Zhou J, et al. Application of eukaryotic and prokaryotic laccases in biosensor and biofuel cells: recent advances and electrochemical aspects. Appl Microbiol Biot. 2018;102(24):10409–10423. doi:10.1007/s00253-018-9421-7
- Zheng F, An Q, Meng G, et al. A novel laccase from white rot fungus Trametes orientalis: purification, characterization, and application. Int J Biol Macromol. 2017;102:758–770. doi:10.1016/j.ijbiomac.2017.04.089
- Bertrand B, Martínez-Morales F, Tinoco-Valencia R, et al. Biochemical and molecular characterization of laccase isoforms produced by the white-rot fungus Trametes versicolor under submerged culture conditions. J Mol Catal B: Enzym. 2015;122:339–347. doi:10.1016/j.molcatb.2015.10.009
- Yasar G, Guven UG, Guduk E, et al. Partial purification and characterization of the novel halotolerant and alkalophilic laccase produced by a new isolate of Bacillus subtilis LP2. Biocatal Biotransfor. 2019;37(4):268–277. doi:10.1080/10242422.2019.1594790
- Yang J, Lin Q, Ng TB, et al. Purification and characterization of a novel laccase from Cerrena sp. HYB07 with dye decolorizing ability. Plos One. 2014;9(10):e110834. doi:10.1371/journal.pone.0110834
- Gunne M, Urlacher VB. Characterization of the alkaline laccase Ssl1 from Streptomyces sviceus with unusual properties discovered by genome mining. Plos One. 2012;7(12):e52360. doi:10.1371/journal.pone.0052360
- Zhang C, Diao H, Lu F, et al. Degradation of triphenylmethane dyes using a temperature and pH stable spore laccase from a novel strain of Bacillus vallismortis. Bioresource Technol. 2012;126:80–86. doi:10.1016/j.biortech.2012.09.055
- Sahay R, Yadav RSS, Yadav KDS. Purification and characterization of laccase secreted by L. lividus. Appl Biochem Biotechnol. 2019;157(2):311–320. doi:10.1007/s12010-008-8265-5
- Kaushik G, Thakur IS. Purification, characterization and usage of thermotolerant laccase from Bacillus species for biodegradation of synthetic dyes. Appl Biochem Microbiol. 2013;49(4):352–359. doi:10.1134/S0003683813040169
- Yao Y, Zhou G, Lin Y, et al. A highly thermotolerant laccase produced by Cerrena unicolor strain CGMCC 5.1011 for complete and stable malachite green decolorization. AMB Express. 2020;10(1):1–11. doi:10.1186/s13568-019-0926-y
- Agrawal K, Shankar J, Verma P. Insight into multicopper oxidase laccase from Myrothecium verrucaria ITCC-8447: a case study using in silico and experimental analysis. Environ Sci Health B. 2020;55(12):1048–1060. doi:10.1080/03601234.2020.1812334
- Agrawal K, Shankar J, Verma P. Multicopper oxidase (MCO) laccase from Stropharia sp. ITCC-8422: an apparent authentication using integrated experimental and in silico analysis. 3 Biotech. 2020;10(9):413. doi:10.1007/s13205-020-02399-8
- Si J, Wu Y, Ma HF, et al. Selection of a pH-and temperature-stable laccase from Ganoderma australe and its application for bioremediation of textile dyes. J Environ Manage. 2021;299:113619. doi:10.1016/j.jenvman.2021.113619
- Si J, Peng F, Cui B. Purification, biochemical characterization and dye decolorization capacity of an alkali-resistant and metal-tolerant laccase from Trametes pubescens. Bioresour Technol. 2013;128:49–57. doi:10.1016/j.biortech.2012.10.085