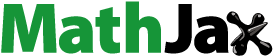
Abstract
Ag-modified ZnO thin films on Zn-electroplated Cu substrate with different Ag-contents were prepared via a simple sol–gel spin-coating procedure followed by a thermal shock method with AgNO3, and then characterized by XRD, Raman, FTIR, FESEM, EDX, and UV-visible absorption spectroscopy. Their photocatalytic performance was evaluated by the degradation of methylene blue. Due to the sol–gel spin-coating process, the film was successfully immobilized on mechanically robust Zn/Cu plates, making it promising for applications in harsh practical conditions and facilitating recovery. When our ZnO thin films were surface-modified with AgNO3 at 1 and 2 mol%, they exhibited the best photocatalytic activity under UVA light and visible light, respectively, which can be explained by the appearance of maze-like architecture on their surface with vertical ZnO walls, surface defects and metallic silver nanoparticles. However, when the Ag-content was increased to 3 mol%, the maze-like structure collapsed, leading to decreases in photocatalytic performance.
GRAPHICAL ABSTRACT

1. Introduction
In recent years, silver-doping has been widely applied as one of the most promising routes to modify ZnO-based photocatalysts [Citation1–3]. It is widely acknowledged that the photocatalytic activity of bare ZnO nanoparticles was typically limited by their large band gap and the fast recombination of photogenerated electron–hole pairs. Conversely, the presence of silver species on the surface of ZnO nanoparticles can effectively broaden the UV-visible absorption spectrum owing to the effect of surface plasmon resonance [Citation4] as well as delay the recombination of charged carriers [Citation5], which improves their photocatalytic performance under both UV light and visible light. Hence, many methods have been proposed to prepare Ag-doped ZnO nanopowders, including coprecipitation [Citation6,Citation7], sol–gel [Citation8,Citation9], hydrothermal [Citation10,Citation11], and green biomimetic methods [Citation12]. Nevertheless, there is always a need for the development of simple and speedy methods to synthesize Ag-doped ZnO photocatalysts. Recently, we successfully modified ZnO nanoparticles with silver by using our own-developed thermal shock method [Citation13], an easy and convenient technique based on the sudden heating of a mixture containing ZnO and AgNO3. This method allowed us to quickly create Ag(0) species on the surface of ZnO nanoparticles, which were believed to be the main reason for the enhanced photocatalytic performance. However, most Ag-doped ZnO nanoparticles still displayed one drawback. Due to their fine powder form, these particles are difficult to separate from the solution after photocatalytic reactions, potentially becoming a source of secondary pollution.
To overcome this problem, several attempts were carried out to prepare Ag-doped ZnO thin films on different recyclable substrates. For example, Thongsuriwong et al. reported the preparation of Ag-doped ZnO thin films coated on glass slides by a sol–gel dip-coating method. Under blacklight irradiation, the prepared films exhibited both high photocatalytic activities for the degradation of methylene blue, rhodamine B, and reactive orange as well as high antibacterial properties against E. coli [Citation14]. Also, Sutanto et al. used the sol–gel spray-coating method to synthesize Ag-substituted ZnO thin films onto glass substrates, which produced enhanced efficiencies on UVA light-induced-photodegradation of methylene blue [Citation15]. In another work, 6 wt% Ag/ZnO thin film was prepared by a sol–gel spin-coating method onto glass substrates and was successfully applied for the photocatalytic degradation of three azo dyes (reactive red 195, reactive yellow 145, and reactive orange 122) under simulated solar illumination [Citation16]. Nevertheless, to the best of our knowledge, there has been no research utilizing the thermal shock method to rapidly modify the surface of ZnO thin films with Ag agents. In addition, most Ag-modified ZnO thin films have been synthesized on glass substrates, which are fragile and susceptible to damage during use.
In summary, to address the conventional disadvantages of powdered ZnO photocatalysts and improve their applicability, it is imperative to develop a process for synthesizing Ag-modified ZnO thin films applied to a sustainable substrate. Therefore, in this study, we introduce a novel approach to prepare Ag-modified ZnO thin films on zinc-electroplated Cu plates. This method involves a straightforward sol–gel spin-coating procedure, followed by our thermal shock technique, in which AgNO3 serves as the modifying agent. The selection of zinc-electroplated Cu plates as the substrate is also a novelty in our research since copper plates usually exhibit high tensile strength, making our photocatalytic systems suitable for practical applications. Moreover, before the immobilization of ZnO nanoparticles on substrates by sol–gel method, the surface of zinc-electroplated Cu plates was oxidized to form ZnO layers, which are expected to provide high bonding strength between ZnO nanoparticles and substrate surface. In this work, the influences of Ag-contents used in thermal shock treatment on the crystal structure, morphology, surface functional groups, optical properties and photocatalytic performances of ZnO thin films under both UVA light and visible light were investigated and discussed in detail.
2. Materials and methods
2.1. Chemicals
In this study, all reagents, including zinc acetate dihydrate (Zn(CH3COO)2.2H2O), ethanol (C2H5OH), diethanolamine (NH(CH2CH2OH)2), silver nitrate (AgNO3), zinc chloride (ZnCl2), boric acid (H3BO3), potassium chloride (KCl), hydrochloric acid (HCl), and methylene blue (C16H18ClN3S.3H2O) are commercially available, reagent grade or analytical grade, and were used as purchased without any additional purification. Distilled water was always used for all experiments. The procedure of thin film synthesis and surface modification with Ag agent is summarized in Figure .
2.2. Synthesis of ZnO nanoparticles immobilized on Zn-electroplated Cu substrates
To enable the steady deposition of zinc oxide nanoparticles on copper substrates, these substrates were coated with zinc layers via an electroplating procedure followed by a chemical oxidation process. In a typical procedure, 25.0 g of H3BO3 and 60.0 g of ZnCl2 were mixed with 1000 mL of distilled water under continuous stirring to prepare the electrolyte solution. Subsequently, 200.0 g of KCl was gently added to this solution. The resulting solution was magnetically stirred at room temperature for 24 h. Before the electroplating process, thin copper plates (70.0 mm × 25.0 mm × 0.5 mm) were pre-treated by immersion in a 1 mol L–1 HCl solution and then polished with wet P1000 sandpapers to remove oxide layers on their surface. Distilled water and ethanol were also used to rinse the copper plates, which provides a clean and uniform surface. Next, a zinc plating system was established with two pure zinc plates functioning as the anodes and a thin copper plate as the cathode. A steady direct current (DC) of 2.5 A dm−2 was applied for 15 min to form a thin layer of metallic zinc across the entire surface of copper substrate. The zinc-electroplated Cu plates were thoroughly washed with distilled water, dried at 150°C for 10 min. Afterwards, a chemical oxidation process was carried out to transform the thin Zn(0) layer to a ZnO layer. This process is necessary to enhance the adhesion between the plate surface and the zinc oxide nanoparticles, which were further coated on the substrates using our sol–gel method. During this process, the Zn-electroplated Cu substrates were completely immersed in 1 mol L–1 NaOH solution for 10 min. The residual NaOH solution was then gently washed off with distilled water, and the plates were dried at 150°C for 10 min.
In the second step, 6.652 g of zinc acetate dihydrate (Zn(CH3COO)2.2H2O), 3.154 g of diethanolamine (DEA), and absolute ethanol were used as the source material, stabilizer, and solvent for the sol–gel spin-coating procedure, respectively. These chemicals were mixed together to obtain 100 mL clear solution. Then, 3.0 mL of distilled water was added dropwise into this solution to facilitate the hydrolysis reaction. The resulting solution was aged at room temperature for three days to produce the coating solution, which is a clear, transparent, and homogenous solution. This solution was loaded into a 25 mL burette placed about 0.5 cm above the surface of spin coater where the treated zinc-electroplated Cu substrate was fixed in the middle. Next, 1.00 mL of the coating solution was dropwise on the surface of substrate and the spinning process was performed at room temperature with the speed of 2000rpm. The obtaining plate was dried at 150°C for 10 min and then annealed for 5 min at 400°C. To achieve the thin film with appropriate thickness, the spin-coating and the subsequent annealing treatment were carried out three times. The final product is labeled as ZnO/Zn/Cu.
2.3. Surface modification of ZnO thin film with Ag agent
In the next section of our study, the surface of ZnO thin films deposited on Zn/Cu plates was modified with Ag agent by a simple thermal shock method. Initially, the ZnO/Zn/Cu samples were immersed in a mixture containing 5.0 mL of absolute ethanol and 15.0 mL of AgNO3 solution with varying molar concentrations (corresponding to the molar Ag+/ZnO ratios of 0.01, 0.02, and 0.03). The samples were dried at 150°C for 30 min and subsequently subjected to a thermal shock process for 5 min by rapidly placing them in an electric furnace preset at 300°C. After the thermal shock, the samples were allowed to cool to room temperature, then thoroughly washed with distilled water and finally dried at 150°C for 15 min to obtain the Ag-modified ZnO thin films, labeled as Ag-ZnO/Zn/Cu-X (where X represents the molar Ag+/ZnO ratio). The numerical photographs of Cu plates after each step of synthesis and surface modification of ZnO thin films are presented in Figure .
2.4. Characterization
In order to investigate the crystallite structure and the phase composition of unmodified and Ag-modified ZnO/Zn/Cu samples, the X-ray diffraction (XRD) patterns were acquired on an EMPYREAN (PANalytical) diffractometer (Cu Kα radiation, λ = 1.5406 Å) in the 2θ range of 5–90° at a scan speed of 0.0260 ° s−1. From these patterns, the average crystallite size of ZnO nanoparticles and the dislocation density (δ, line m–2) were calculated using the following formulas [Citation17]:
(1)
(1)
(2)
(2) where d is the crystallite size corresponding to the (hkl) plane, k is the constant depending on crystallite shape (0.94), λ is the wavelength of Cu Kα radiation (1.5406 Å), β is the full width at half maximum (FWHM) of the most intense peaks, θ is their diffraction angle, and δ (line nm–2) is the dislocation density.
The Raman spectra were recorded using a HORIBA XploRA One Raman microscope equipped with a 532-nm laser source to further characterize the crystalline structure and the surface defects. The morphology and the particle size were observed by field emission scanning electron microscopy (HITACHI S-4800, Japan) with an accelerating voltage of 10.0 kV. The HITACHI S-4800 instrument was also used to record the energy-dispersive X-ray (EDX) spectra of our samples in order to determine their elemental composition. Moreover, a V-770 UV-Visible/NIR spectrophotometer (Jasco, Japan) was employed to study the optical properties of Ag-ZnO/Zn/Cu-X samples. Their Fourier transform infrared (FTIR) spectra were measured by a Frontier FT-IR/NIR spectrometer to determine their surface functional groups.
2.5. Photocatalytic tests
The UVA-induced or visible light-induced photocatalytic performances of ZnO/Zn/Cu and Ag-ZnO/Zn/Cu-X samples were evaluated via the degradation of methylene blue (MB) under an UVA lamp (9-W Osram Dulux S) or a visible light lamp (9-W Radium 78), respectively. During a typical photocatalytic test, three identical photocatalytic samples were immersed in a glass jacketed reaction beaker containing 250.0 mL of MB solution (10−5 M) at an initial pH of 7. A water circulation system was used to maintain the solution temperature at around 30°C. Prior to illumination, the solution was stirred continuously in the dark for 60 min to establish the adsorption–desorption equilibrium of MB molecules on the surface of photocatalytic materials. At regular intervals, 5 mL of the aliquots were taken and analyzed at 664 nm using a Helios Omega UV-Visible spectrophotometer.
3. Results and discussion
3.1. XRD results
The structural investigation of our prepared ZnO thin films was carried out by XRD characterization, as depicted in Figure . All the XRD patterns exhibit the characteristic peaks centered at 34.95°, 37.86°, 43.18°, 48.03°, 62.80°, and 79.34°, which are well matched with the binary Cu5Zn8 alloy phase (JCPDS card number 025-1228). The presence of ZnO wurtzite phase (JCPDS card number 36–145) was also identified by the diffraction peaks at 31.80° ((100) line), 34.47° ((002) line), 36.29° ((101) line), 47.60° ((102) line), 56.60° ((110) line), and 62.94° ((103) line), proving the successful formation of ZnO thin film on Zn/Cu plates. These diffraction peaks are well symmetrical and intense, which indicates a relatively high crystallinity for both crystalline phases in our Ag-ZnO/Zn/Cu-X samples. Notably, among the various characteristic peaks of the ZnO wurtzite phase, the peak at 31.80° is consistently the most intense, suggesting the preferential growth of the (100) crystal plane of ZnO on the surface of oxidized Zn/Cu substrates. Moreover, the Ag-modified samples revealed some additional diffraction peaks at 37.88°, 64.71°, and 77.54°, which can be attributed to the (111), (220), and (311) crystal planes of the face-centered-cubic (FCC) phase of metallic Ag (JCPDS card number 04-0783), respectively. This result demonstrates that the thermal shock treatment of ZnO thin film with AgNO3 can effectively create Ag(0) species on the surface of our catalysts. Besides, it should be noted that the FCC phase of metallic Ag is usually identified via 4 characteristic peaks situated at 37.9° ((111) line), 44.3° ((200) line), 64.5° ((220) line), and 77.5° ((311) line). Hence the absence of (200) crystal plane could be related to the effects of thin film formation on a specific substrate, which may limit the growth of certain crystal planes. Table displays the crystallite size and dislocation density for all of our samples. The crystallite size of ZnO in the thin film was observed to increase when a small amount of Ag (1 mol%) was introduced for surface modification, resulting in the reduction of dislocation density. However, as the Ag content rises from 1 mol% to 2 and 3 mol%, the crystallite size shows a tendency to decrease, leading to the increase in dislocation density, which is similar to the findings of Sabry et al. [Citation7].
Figure 3. XRD patterns of unmodified ZnO/Zn/Cu and surface-modified ZnO/Zn/Cu samples with different Ag-contents.

Table 1. Average crystallite size and dislocation density of ZnO/Zn/Cu and Ag-ZnO/Zn/Cu-X samples.
3.2. Raman spectra
Figure presents the Raman spectra of unmodified and surface-modified ZnO/Zn/Cu samples. The Raman data is summarized in Table . All the Raman spectra reveal the characteristic vibrational modes of ZnO, including A1 transverse-optical (TO), high frequency E2, and A1 longitudinal optical (LO) modes identified by the peaks at 398, 437, and 581 cm–1, respectively. Among them, the E2 (high) vibration mode is attributed to the hexagonal wurtzite phase [Citation18,Citation19], which is in good agreement with the XRD results whereas the A1 (LO) vibration mode is commonly associated with the presence of defects in ZnO lattice, such as oxygen vacancies and/or zinc interstitials [Citation20–22]. Two other Raman peaks are found at 334 and 500 cm−1, which can be assigned to the E2 (high) – E2 (low) and E1 (transverse-optical) + E2 (low) multiphonon scattering modes, respectively [Citation23]. As shown in Figure , although the Raman spectra of ZnO/Zn/Cu and Ag-ZnO/Zn/Cu-0 samples are quite similar, the intensity ratio of the A1 (LO) peak to the E2 (high) peak of Ag-ZnO/Zn/Cu-0 is higher than that of ZnO/Zn/Cu, indicating the increase in surface defects due to the thermal shock process [Citation19].
Table 2. Summary of Raman data of unmodified and surface-modified ZnO/Zn/Cu samples.
Figure 4. Raman spectra of unmodified ZnO/Zn/Cu and surface-modified ZnO/Zn/Cu samples with different Ag-contents.

For Ag-modified samples (Ag-ZnO/Zn/Cu-1, Ag-ZnO/Zn/Cu-2, and Ag-ZnO/Zn/Cu-3), the E2 (high) – E2 (low) mode is not clearly observed but the characteristic A1 (TO), E2 (high), and E1 (TO) + E2 (low) modes remain detectable. It was also observed that the A1 (LO) peak is likely to grow further, broaden and shift towards lower wavenumbers (from 581 cm−1 to approximately 560 cm−1). Moreover, in these samples, a broad Raman peak was found at around 480 cm−1. These evolutions are consistent with previous studies on Ag-doped ZnO photocatalysts [Citation23–25], indicating the impacts of thermal-shock-modification with AgNO3 on the surface of our catalysts.
3.3. FTIR spectra
FTIR spectroscopy was employed to investigate the impact of thermal shock with AgNO3 on the surface functional groups of our samples (Figure ). All these materials show an intense absorption peak in the fingerprint region, at about 447–468 cm–1, which is assigned to the interatomic Zn–O stretching bonds, confirming the successful formation of ZnO on the surface of Zn-electroplated Cu plates [Citation26–28]. Moreover, this peak shows a tendency to shift to higher wavenumbers (460–468 cm–1) when the samples were surface-modified with AgNO3. In the previous works, the blue shift of Zn–O peak could be explained by the changes in bond length due to the incorporation of Ag+ ions in ZnO lattice [Citation29] or to the formation of Ag-complexes related to Zn sites [Citation30]. Besides, three Ag-modified ZnO/Zn/Cu samples exhibit a broad absorption band at 3448–3450 cm–1 and a small peak at 1626 cm–1, corresponding to the surface O-H stretching and bending vibrations, respectively [Citation27,Citation31]. It was also observed that without Ag-modification, the ZnO/Zn/Cu and Ag-ZnO/Zn/Cu-0 only show very weak absorption band at around 3434 cm–1. These results suggest that the surface Ag-modification by thermal shock method can enhanced the amount of hydroxyl groups on the surface of ZnO thin films, which is expected to affect their photocatalytic activities.
3.4. FESEM images and EDX results
Figure displays the microscopic morphologies of Zn/Cu substrate, unmodified and surface-modified ZnO/Zn/Cu materials investigated through FESEM analysis. As shown in Figure a and b, after surface oxidation with NaOH solution, the Zn/Cu substrate appears to exhibit a structure of parallel and stacked nanosheets, forming adjacent multi-layered plateaus. However, when ZnO thin film was coated on Zn/Cu substrate by sol–gel process, the surface underwent significant modification. It was observed that ZnO/Zn/Cu sample consists of uniform, spherical ZnO nanoparticles with particle sizes ranging from approximately 12–25 nm (Figure c and d). These nanoparticles aggregate to build a short chain-wall-like architecture with countless vertical walls spreading across the whole surface. The distance between such vertical walls is found to be about 25–75 nm. For Ag-ZnO/Zn/Cu-0 (the sample treated by thermal shock process without AgNO3), the vertical walls appear to be elongated and fused together in large bundles (Figure e and f).
Figure 6. FESEM images (with different magnifications) of Zn/Cu substrate (a, b), unmodified ZnO/Zn/Cu (c, d), Ag-ZnO/Zn/Cu-0 (e, f), Ag-ZnO/Zn/Cu-1 (g, h), Ag-ZnO/Zn/Cu-2 (i, j), and Ag-ZnO/Zn/Cu-3 (k, l) samples.

Interestingly, in the presence of AgNO3, the thermal shock treatment was found to significantly affect the morphology of ZnO thin films. In fact, when modifying by thermal shock method with AgNO3, the vertical walls composed of ZnO nanoparticles were separated to each other, with distances between them ranging from 50 to 150 nm. This creates a maze-like structure on the surface of Ag-ZnO/Zn/Cu-1 (Figure g and h) and Ag-ZnO/Zn/Cu-2 samples (Figure i and j). Additionally, numerous quasi-spherical particles were detected on the top of the walls or inside the spaces between the walls. These nanoparticles, with the particle size of 20–150 nm, should be attributed to Ag(0) nanoparticles, which is in good agreement with the XRD results. Nevertheless, when the Ag-content reached 3 mol%, the maze-like structure seems to collapse, leaving behind many thick and overlapping ZnO slates (Figure k and l). Notably, the Ag(0) nanoparticles tended to grow and aggregate into large clusters with the particle size of 50–300 nm, which is significantly larger than the size of Ag(0) nanoparticles in our other Ag-modified samples.
In order to quantify the surface elemental composition of our samples, the EDX analysis was performed (Table ). Accordingly, no sign of copper element was observed for all samples, indicating that the accelerated electron beam used in EDX technique is not able to reach the Cu substrate. Furthermore, no impurity element is detected. The ZnO/Zn/Cu and Ag-ZnO/Zn/Cu-0 samples only show the presence of zinc and oxygen elements on their surface with the atomic Zn/O ratio close to the stoichiometry of ZnO. This suggests that the ZnO thin film prepared by our sol–gel spin-coating procedure nearly covered the entire surface of Zn/Cu substrate. For all Ag-doped samples, the silver element is observed in their surface elemental composition as an obvious consequence of thermal shock modification with AgNO3. The atomic percentage of silver was also found to increase with the molar concentration of AgNO3.
Table 3. Surface elemental composition and band gap energy (Eg) of unmodified ZnO/Zn/Cu and surface-modified ZnO/Zn/Cu samples.
3.5. UV-visible absorption spectra
Figure displays the UV-visible absorption spectra of ZnO/Zn/Cu and Ag-ZnO/Zn/Cu-X materials. Using the Kubelka–Munk function, the band gap energies (Eg) of these samples were calculated and presented in Table . A broad and strong absorption band in both UVB and UVA regions was observed for all our samples, indicating that these samples can respond to UV light for photocatalytic reactions. According to Table , the band gap values of Ag-modified ZnO thin films exhibit negligible deviation when compared to the unmodified sample or modified sample without Ag agent. However, both Ag-ZnO/Zn/Cu-1, Ag-ZnO/Zn/Cu-2, and Ag-ZnO/Zn/Cu-3 samples reveal more intense UV absorption band (with the maximum absorption at 315 nm) than ZnO/Zn/Cu and Ag-ZnO/Zn/Cu-0 samples, which could lead to higher UV-induced photocatalytic performance for Ag-modified samples. Besides, the surface modification with AgNO3 was also able to create an absorption band in the visible region (from 415 to 715 nm), corresponding to the dark colors of Ag-modified samples. This enhanced visible-light absorption can be explained by the surface plasmon resonance effects of Ag nanoparticles on the surface of ZnO thin films [Citation24,Citation31–34]. As a consequence, when the Ag-content increases, the absorption in the visible light region was found to be improved.
3.6. Photocatalytic activity
Figure a and b depict the ln(C0/C) versus time plots of MB degradation in the presence of our photocatalytic materials under UVA and visible light irradiation, respectively. The UV-visible spectral evolution of MB solution during the UVA light-induced photocatalytic tests of Ag-ZnO/Zn/Cu-1 and Ag-ZnO/Zn/Cu-2 samples were also presented in Figure c and d, respectively. These figures revealed a consistent reduction in the absorbance intensity of MB solution at 664 nm when exposed to UVA light in the presence of our Ag-modified ZnO thin films, which can be attributed to the photocatalytic activities of ZnO nanoparticles deposited on the surface of thin films. In fact, according to the photocatalytic mechanism of semiconductors [Citation35,Citation36], under appropriate light irradiation, the ZnO nanoparticles can be excited to produced electron–hole pairs. These photogenerated charge carriers are able to migrate to the surface and react with adsorbed oxygen and water molecules, leading to the formation of various radicals, such as hydroxyl radicals. Thanks to their strong and non-selective oxidation ability, the hydroxyl radicals can mineralize MB molecules, causing the reduction of MB absorbance intensity. Furthermore, as evidenced in Figure a and b, the MB degradation is proved to follow the pseudo-first-order Langmuir–Hinshelwood kinetic model. Hence, the apparent rate constants (kapp) can be calculated by the following equation (EquationEq. 3(3)
(3) )
(3)
(3) where C is the MB concentration (mol L−1) at time t and C0 is the initial MB concentration (mol L−1).
Figure 8. Ln(C0/C) versus time plot of MB degradation under UVA irradiation (a) and under visible light (b) for unmodified ZnO/Zn/Cu and surface-modified ZnO/Zn/Cu samples with different Ag-contents. C is the MB concentration (mol L−1) at time t and C0 is the initial MB concentration (mol L−1). UV-visible spectral evolutions of MB solution during the UVA light-induced photocatalytic tests of Ag-ZnO/Zn/Cu-1 (c) and Ag-ZnO/Zn/Cu-2 (d) samples.

These rate constants are used to evaluate the photocatalytic activities of these samples (Table ). Accordingly, three Ag-modified samples with varying Ag+ contents from 1 mol% to 3 mol% exhibit superior rate constants than the unmodified ZnO/Zn/Cu sample under both UVA and visible light irradiation, which proves that the thermal-shock modification with AgNO3 can effectively improve the photocatalytic performance of ZnO thin films. It should be noted that the rate constant of MB degradation in the presence of Ag-ZnO/Zn/Cu-0 was also found to be higher than that of unmodified sample (but still lower than Ag-modified samples), indicating that the thermal shock process (without AgNO3) also contributes to the enhanced photocatalytic activity.
Table 4. Comparison of MB degradation rate constants and MB degradation efficiency per area of unmodified ZnO/Zn/Cu and surface-modified Ag-ZnO/Zn/Cu-X samples under UVA light and visible light irradiation.
Remarkably, our Ag-ZnO/Zn/Cu-1 sample showed the highest rate constant of MB degradation under UVA illumination (kapp = 0.140 h−1), which is approximately 2.4 times greater than that of unmodified sample. However, under visible light irradiation, the best photocatalyst is Ag-ZnO/Zn/Cu-2 sample with the highest kapp value (kapp = 0.0169 h−1), which is about 1.7 times higher than that of ZnO/Zn/Cu sample. These results highlight the effectiveness of our thermal shock method and emphasize the critical role of Ag+ content in the improvement of ZnO/Zn/Cu photocatalytic systems. Table compares the MB degradation rate constants of our photocatalytic thin films with undoped and Ag-doped ZnO thin films of other reports [Citation37–40]. It is evident that the photocatalysts in our work exhibited superior MB decomposition efficiency compared to certain studies, while they do not perform as well as others. However, it should be noted that this comparison is solely for reference, as these studies employed different catalyst quantities, light source powers, and MB concentrations.
Table 5. Comparative MB degradation rate constants of photocatalytic ZnO-based thin films in this study and previous reports.
3.7. Discussion
According to the experimental results, the thermal shock method without AgNO3 can slightly enhance the photocatalytic activity of ZnO thin film. However, when combined with AgNO3, the thermal shock impressively further improved the performance of ZnO/Zn/Cu photocatalysts under both UVA light and visible light. This great enhancement can be attributed to a series of complex changes in the crystal structure, morphology, surface hydroxyl groups, surface structure, and elemental composition, due to the synergic effects of our thermal shock treatment and the incorporation of AgNO3 as a doping agent.
As shown in FESEM images, the two best photocatalysts, Ag-ZnO/Zn/Cu-1 and Ag-ZnO/Zn/Cu-2, show the maze-like structure with great distance between the vertical walls. Such surface architecture most likely leads to larger light-exposed area, thereby enhancing the photocatalytic activity. In contrast, the samples with a denser chain-wall structure and narrower spaces between the vertical walls on their surface demonstrate lower photocatalytic activity. This trend is particularly evident for the photocatalytic activity in the UVA region. In fact, the Ag-ZnO/Zn/Cu-1 sample exhibits the highest UVA-induced photocatalytic activity owing to the higher distance between the vertical walls than other samples. As the molar concentration of AgNO3, used in the surface modification procedure, increased, the presence of Ag(0) nanoparticles became denser, consequently reducing the inter-wall spaces and then declining the photocatalytic activity.
Moreover, although the thermal shock method does not considerably affect the crystal structure of ZnO, it does increase the number of surface defects of ZnO nanoparticles, as indicated by Raman spectra. The presence of surface oxygen vacancies or zinc vacancies has been reported to act as electron traps, inhibiting the recombination of photogenerated electron–hole pairs [Citation41,Citation42]. Besides, the surface oxygen vacancies have been known as active sites for water dissociation, which can produce hydroxyl groups on the surface of ZnO nanoparticles [Citation43]. It was observed that all three Ag-modified samples show an intense absorption peak at 3448–3450 cm–1 in their FTIR spectra, indicating a large number of surface hydroxyl groups. Since hydroxyl radicals are usually generated by the reaction between surface hydroxyl groups and photogenerated holes [Citation44], the large amount of hydroxyl groups on the surface of Ag-modified samples should be also considered as one of the reasons for their high photocatalytic performance. Additionally, such types of defects can create intermediate energy levels near the valence band of ZnO, facilitating the absorption of visible light and thereby further improving the photocatalytic activity under the visible light illumination [Citation45,Citation46].
Last but not least, the presence of metallic silver on the sample surface, proved by XRD, FESEM, and EDX studies, should play a vital role in the photocatalytic reactions of ZnO thin films. It was proved that the photogenerated electrons can flow from the conduction band of ZnO semiconductor to the conduction band of Ag nanoparticles owing to the alignment of the Fermi levels of Ag and ZnO [Citation47,Citation48]. This electron migration can reduce the recombination of photogenerated electron and holes, which prolongs the lifetime of these charge carriers and consequently enhances the photocatalytic activity. Besides, the surface plasmon resonance effect of Ag(0) nanoparticles also contributes significantly to the visible light absorption of our Ag-modified ZnO/Zn/Cu samples, which can produce many electrons on Ag surface [Citation49]. Then, these electrons can be injected from the surface plasmon band of Ag nanoparticles into the conduction band of ZnO, which further promotes the photocatalytic process. As a result, when the Ag-content increases, the photocatalytic performance, especially under visible light, was clearly enhanced. However, when the Ag-content exceeded 2 mol%, the maze-like structure of ZnO walls on the surface of our catalyst was shattered, which reduced the photocatalytic activity under both UVA light and visible light.
4. Conclusion
In this work, ZnO thin films were successfully deposited on Zn-electroplated Cu substrates using a simple sol–gel method and subsequently surface-modified by thermal shock method with AgNO3 at different molar concentrations. The resulting ZnO thin film was observed to cover nearly the entire surface of our materials. The characterization studies also revealed that the phase composition, surface structure, surface functional groups, morphology, and surface elemental composition of these samples were strongly influenced by the thermal shock process as well as the Ag-content used in the modification procedure. As a result, our ZnO/Zn/Cu samples, modified with 1 mol% Ag and 2 mol% Al, exhibited the best photocatalytic activities under UVA light and visible light, respectively. The enhancement in photocatalytic activity can be attributed to the formation of maze-like architecture on the surface of these samples, the generation of surface defects, the increase in surface hydroxyl groups, and the surface plasmon resonance effects of silver nanoparticles. Furthermore, the deposition of ZnO thin films on Zn-electroplated Cu substrates allows for the effortless and rapid separation of these photocatalysts from the solution after treatment, which promises to facilitate their practical applications.
Disclosure statement
No potential conflict of interest was reported by the author(s).
Additional information
Funding
References
- Nagasundari SM, Muthu K, Kaviyarasu K, et al. Current trends of silver doped zinc oxide nanowires photocatalytic degradation for energy and environmental application. Surf Interfaces. 2021;23:100931, doi:10.1016/j.surfin.2021.100931
- Ifijen HI, Maliki M, Anegbe B. Synthesis, photocatalytic degradation and antibacterial properties of selenium or silver doped zinc oxide nanoparticles: a detailed review. OpenNano. 2022;8:100082, doi:10.1016/j.onano.2022.100082
- Rajput RB, Shaikh R, Sawant J, et al. Recent developments in ZnO-based heterostructures as photoelectrocatalysts for wastewater treatment: a review. Environ Adv. 2022;9:100264, doi:10.1016/j.envadv.2022.100264
- Chen KH, Pu YC, Chang KD, et al. Ag-nanoparticle-decorated SiO2 nanospheres exhibiting remarkable plasmon-mediated photocatalytic properties. J Phys Chem C. 2012;116(35):19039–19045. doi:10.1021/jp306555j
- Deng Q, Duan X, Ng DHL, et al. Ag nanoparticle decorated nanoporous ZnO microrods and their enhanced photocatalytic activities. ACS Appl Mater Interfaces. 2012;4(11):6030–6037. doi:10.1021/am301682g
- Kareem MA, Bello IT, Shittu HA, et al. Synthesis, characterization, and photocatalytic application of silver doped zinc oxide nanoparticles. Clean Mater. 2022;3:100041, doi:10.1016/j.clema.2022.100041
- Sabry RS, Aziz WJ, Rahmah MI. Employed silver doping to improved photocatalytic properties of ZnO micro/nanostructures. J Inorg Organomet Polym. 2020;30:4533–4543. doi:10.1007/s10904-020-01661-z
- Onkani SP, Diagboya PN, Mtunzi FM, et al. Comparative study of the photocatalytic degradation of 2–chlorophenol under UV irradiation using pristine and Ag-doped species of TiO2, ZnO and ZnS photocatalysts. J Environ Manage. 2020;260:110145, doi:10.1016/j.jenvman.2020.110145
- Zhu X, Wang J, Yang D, et al. Fabrication, characterization and high photocatalytic activity of Ag–ZnO heterojunctions under UV-visible light. RSC Adv. 2021;11(44):27257–27266. doi:10.1039/D1RA05060E
- Turkyılmaz SS, Guy N, Ozacar M. Photocatalytic efficiencies of Ni, Mn. Photocatalytic efficiencies of Ni, Mn, Fe and Ag doped ZnO nanostructures synthesized by hydrothermal method: The synergistic/antagonistic effect between ZnO and metals. J Photochem Photobiol A. 2017;341:39–50. doi:10.1016/j.jphotochem.2017.03.027
- Rahmah MI, Majdi HS, WK A-A, et al. Synthesis of ZnO/Ag-doped C/N heterostructure for photocatalytic application. Int J Mod Phys B. 2023;37(24):2350239, doi:10.1142/S0217979223502399
- Choudhary MK, Kataria J, Bhardwajc VK, et al. Green biomimetic preparation of efficient Ag–ZnO heterojunctions with excellent photocatalytic performance under solar light irradiation: a novel biogenic-deposition-precipitation approach. Nanoscale Adv. 2019;1(3):1035–1044. doi:10.1039/C8NA00318A
- Nguyen HTP, Nguyen TMT, Hoang CN, et al. Characterization and photocatalytic activity of new photocatalysts based on Ag, F-modified ZnO nanoparticles prepared by thermal shock method. Arab J Chem. 2020;13(1):1837–1847. doi:10.1016/j.arabjc.2018.01.018
- Thongsuriwong K, Amornpitoksuk P, Suwanboon S. Photocatalytic and antibacterial activities of Ag-doped ZnO thin films prepared by a sol–gel dip-coating method. J Sol-Gel Sci Technol. 2012;62(3):304–312. doi:10.1007/s10971-012-2725-7
- Sutanto H, Wibowo S, Nurhasanah I, et al. Ag doped ZnO thin films synthesized by spray coating technique for methylene blue photodegradation under UV irradiation. Int J Chem Eng. 2016;2016; doi:10.1155/2016/6195326
- Abdelsamad AMA, Gad-Allah TA, Mahmoud FA, et al. Enhanced photocatalytic degradation of textile wastewater using Ag/ZnO thin films. J Water Process Eng. 2018;25:88–95. doi:10.1016/j.jwpe.2018.07.002
- Balsure SD, Gurav M, Kadam RH, et al. X-ray line profile analysis and magnetic and optical properties of FexZn0.95–xCr0.05O nanoparticles fabricated by sol-gel route. Cerâmica. 2022;68:24–32. doi:10.1590/0366-69132022683853187
- Yahia SB, Znaidi L, Kanaev A, et al. Raman study of oriented ZnO thin films deposited by sol–gel method. Spectrochim Acta A Mol Biomol Spectrosc. 2008;71(4):1234–1238. doi:10.1016/j.saa.2008.03.032
- Khan TM, Bibi T, Hussain B. Synthesis and optical study of heat-treated ZnO nanopowder for optoelectronic applications. Bull Mater Sci. 2015;38(7):1851–1858. doi:10.1007/s12034-015-1103-9
- Georgekutty R, Seery MK, Pillai SC. A highly efficient Ag-ZnO photocatalyst: synthesis, properties, and mechanism. J Phys Chem C. 2008;112(35):13563–13570. doi:10.1021/jp802729a
- Cai Y, Fan H, Xu M, et al. Rapid photocatalytic activity and honeycomb Ag/ZnO heterostructures via solution combustion synthesis. Colloids Surf A Physicochem Eng Asp. 2013;436:787–795. doi:10.1016/j.colsurfa.2013.08.008
- Mahardika T, Putri NA, Putri AE, et al. Rapid and low temperature synthesis of Ag nanoparticles on the ZnO nanorods for photocatalytic activity improvement. Results Phys. 2019;13:102209, doi:10.1016/j.rinp.2019.102209
- Zeferino RS, Flores MB, Pal U. Photoluminescence and Raman scattering in Ag-doped ZnO nanoparticles. J Appl Phys. 2011;109(1):014308, doi:10.1063/1.3530631
- Keshari AK, Singh M. Precession controlled synthesis and ligands assisted modulation of optical properties and Raman scattering in Ag doped ZnO nano-egg. Physica E Low Dimens Syst Nanostruct. 2020;123:114177, doi:10.1016/j.physe.2020.114177
- Sridhar A, Sakthivel P, Saravanakumar K, et al. Dual doping effect of Ag+ & Al3+ on the structural, optical, photocatalytic properties of ZnO nanoparticles. Appl Surf Sci Adv. 2023;13:100382, doi:10.1016/j.apsadv.2023.100382
- Li X, He G, Xiao G, et al. Synthesis and morphology control of ZnO nanostructures in microemulsions. J Colloid Interface Sci. 2009;333(2):465–473. doi:10.1016/j.jcis.2009.02.029
- Zhang G, Shen X, Yang Y. Facile synthesis of monodisperse porous ZnO spheres by a soluble starch-assisted method and their photocatalytic activity. J Phys Chem C. 2011;115(15):7145–7152. doi:10.1021/jp110256s
- Dhayagude AC, Nikam SV, Kapoor S, et al. Effect of electrolytic media on the photophysical properties and photocatalytic activity of zinc oxide nanoparticles synthesized by simple electrochemical method. J Mol Liq. 2017;232:290–303. doi:10.1016/j.molliq.2017.02.074
- Irine TM, Rathika A. Synthesis of silver (Ag) doped zinc oxide (ZnO) nanoparticles as efficientphotocatalytic activity for degradation methylene blue dye. J Adv Sci Res. 2022;13(2):129–135. doi:10.55218/JASR.202213217
- Shreema K, Mathammal R, Kalaiselvi V, et al. Green synthesis of silver doped zinc oxide nanoparticles using fresh leaf extract morinda citrifoliaand its antioxidant potential. Mater Today Proc. 2021;47:2126–2131. doi:10.1016/j.matpr.2021.04.627
- Maity J, Roy D, Bala T. Template-free synthesis of hexagonal ZnO disk and ZnO–Ag composite as potential photocatalyst. Hybrid Adv. 2023;3:100055, doi:10.1016/j.hybadv.2023.100055
- Yi Z, Xu X, Kang X, et al. Fabrication of well-aligned ZnO@Ag nanorod arrays with effective charge transfer for surface-enhanced Raman scattering. Surf Coat Technol. 2017;324:257–263. doi:10.1016/j.surfcoat.2017.05.084
- Pal AK, Pagal S, Prashanth K, et al. Ag/ZnO/Au 3D hybrid structured reusable SERS substrate as highly sensitive platform for DNA detection. Sens Actuators B Chem. 2019;279:157–169. doi:10.1016/j.snb.2018.09.085
- Chen X, Zhu L, Ma Z, et al. Derivatization reaction-based surface-enhanced Raman scattering for detection of methanol in transformer oil using Ag/ZnO composite nanoflower substrate. Appl Surf Sci. 2022;604:154442, doi:10.1016/j.apsusc.2022.154442
- Qi K, Cheng B, Yu J, et al. Review on the improvement of the photocatalytic and antibacterial. activities of ZnO. J Alloys Compd. 2017;727:792–820. doi:10.1016/j.jallcom.2017.08.142
- Waghchaure RH, Adole VA, Jagdale BS. Photocatalytic degradation of methylene blue, rhodamine B, methyl orange and eriochrome black T dyes by modified ZnO nanocatalysts: A concise review. Inorg Chem Commun. 2022;143:109764, doi:10.1016/j.inoche.2022.109764
- Hunge YM. Photoelectrocatalytic degradation of methylene blue using spray deposited ZnO thin films under UV illumination. MOJ Poly Sci. 2017;1(4):135–139. doi:10.15406/mojps.2017.01.00020.
- Navidpour AH, Kalantari Y, Salehi M, et al. Plasma-sprayed photocatalytic zinc oxide coatings. J Therm Spray Tech. 2017;26:717–727. doi:10.1007/s11666-017-0541-x
- Vallejo W, Cantillo A, Díaz-Uribe C. Methylene blue photodegradation under visible irradiation on Ag-doped ZnO thin films. Int J Photoenergy. 2020;2020:1, doi:10.1155/2020/1627498
- Daher EA, Riachi B, Chamoun J, et al. New approach for designing wrinkled and porous ZnO thin films for photocatalytic applications. Colloids Surf A Physicochem Eng Asp. 2023;658:130628, doi:10.1016/j.colsurfa.2022.130628
- Le TK, Flahaut D, Martinez H, et al. Surface fluorination of single-phase TiO2 by thermal shock method for enhanced UV and visible light induced photocatalytic activity. Appl Catal B. 2014;144:1–11. doi:10.1016/j.apcatb.2013.06.027
- Wang C, Wu D, Wang P, et al. Effect of oxygen vacancy on enhanced photocatalytic activity of reduced ZnO nanorod arrays. Appl Surf Sci. 2015;325:112–116. doi:10.1016/j.apsusc.2014.11.003
- Namai Y, Matsuoka O. Chain structures of surface hydroxyl groups formed via line oxygen vacancies on TiO2 (110) surfaces studied using noncontact atomic force microscopy. J Phys Chem B. 2005;109(50):23948–23954. doi:10.1021/jp058210r
- Minero C, Mariella G, Maurino V, et al. Photocatalytic transformation of organic compounds in the presence of inorganic ions. 2. Competitive reactions of phenol and alcohols on a titanium dioxide−fluoride system. Langmuir. 2000;16(23):8964–8972. doi:10.1021/la0005863
- Guo MY, Ng AMC, Liu F, et al. Effect of native defects on photocatalytic properties of ZnO. J Phys Chem C. 2011;115(22):11095–11101. doi:10.1021/jp200926u
- Xuan TMC, Tran TN, Botto C, et al. Zinc-containing precursor dependence of hydrothermal method for the synthesis of N-doped ZnO photocatalysts. Chem Eng Commun. 2021;208(2):149–158. doi:10.1080/00986445.2019.1694917
- Li Y, Wang Y, Liu L, et al. Ag/ZnO hollow sphere composites: reusable photocatalyst for photocatalytic degradation of 17α-ethinylestradiol. Environ Sci Pollut Res. 2014;21:5177–5186. doi:10.1007/s11356-013-2133-8
- Saravanan R, Karthikeyan N, Gupta VK, et al. Zno/Ag nanocomposite: an efficient catalyst for degradation studies of textile effluents under visible light. Mater Sci Eng C. 2013;33(4):2235–2244. doi:10.1016/j.msec.2013.01.046
- Li M, Xing Z, Jiang J, et al. Surface plasmon resonance-enhanced visible-light-driven photocatalysis by Ag nanoparticles decorated S-TiO2− nanorods. J Taiwan Inst Chem Eng. 2018;82:198–204. doi:10.1016/j.jtice.2017.11.023