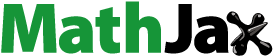
Abstract
Medical-grade polymers present challenges in long-term healthcare waste management due to resistance in degradation mechanisms. Collaborative nanocomposite technology in biomedical polymers holds promise for enhancing biodegradability. This study examined the biodegradability of LLDPE/TiO2-ZnO film in various environmental conditions (compost soil, pond soil, coastline, and landfill soil, hydrolytic and simulated body fluid models) and further assessment using field emission scanning electron microscopy (FESEM) and Coupled Plasma Optical Emission spectroscopy. Nanocomposite present in LLDPE polymer could enhance the biodegradability potential of the film possibility via hydrolysis and free radicals’ activities, especially in high moisture content environment. Deformation holes in the film were observed via FESEM confirming degradability activities. The biodegradability rate is also could be influenced by microbe-rich environments. The insights gained from this study have the potential to contribute to the advancement of biodegradable polymers and the improvement of waste management technology in the healthcare industry.
1. Introduction
Synthetic polymeric materials are important for healthcare-related products such as tubing, IV fluid bottles, blood bags, sutures, dialysis membrane, catheter, syringes, feeding tubes implants [Citation1–6]. Polyethylene (PE) is the most common thermoplastic produced globally because of its excellent mechanical properties, chemical inertness, low-cost production, and ease of manufacturing process [Citation7–10]. And linear low-density polyethylene (LLDPE) has gained much interest amongst researchers in biomedical field for better tensile strength, flexibility, stress cracking adjustment and biocompatibility properties [Citation11].
Nevertheless, this medical-grade polymer has exhibited significant resistance to degradation mechanisms [Citation1] and is often associated with long-term healthcare waste management challenges [Citation12], as it may take up to 100 years to degrade [Citation13–16]. The World Health Organization (WHO) has defined medical waste encompasses various sources, including but not limited to hospitals, healthcare facilities, research institutions, mortuaries, autopsy centres, animal research and testing labs, blood banks, collection services, and residential care facilities for the elderly. Lim and San Thian [Citation16] have highlighted the importance of biodegradable polymer in managing plastic waste. In general, polymer degradation can occur by hydrolysis, photo-oxidation, chemical/enzymatic, irradiation, physical and microbes assisted degradation [Citation9,Citation17].
Collaborative nanocomposite technology within medical-grade polymers holds the promise of enhanced biodegradability. A nanocomposite is a term used to describe a combination of two or more nanomaterials, each contributing unique properties, thereby offering advantages across various industries [Citation18–27]. Previous preliminary investigations into LLDPE/TiO2-ZnO films using an in vitro system revealed their potential for biodegradation, aided by Pseudomonas aeruginosa bacteria [Citation28]. In this study, we delve further into the continuous assessment of LLDPE/TiO2-ZnO nanocomposite films in real-world environments to gain a deeper understanding of their biodegradability, with a particular focus on employing in situ models.
2. Experimental
The study stands out for its interdisciplinary methodology, which encompasses materials science, microbiology, and environmental science.
2.1. Synthesis of LLDPE/TiO2-ZnO nanocomposite films
Solvents used in this study; titanium (IV) isopropoxide (97%, Sigma Aldrich), Zinc acetate dehydrate (98%, Sigma Aldrich), ethanol (95%, Biotech Lab Supplies) and 1,2-dichlorobenzene (Merck) were purchased to synthesis the TiO2-ZnO photocatalyst. Whereas, LLDPE pellets were purchased from Lotte Chemical Titan (M) Sdn. Bhd. It was used as received without further purification. Briefly, both TiO2 and ZnO photocatalysts were synthesized separately and mixed heterogeneously using the sol-gel method to the exact molar ratio TiO2-ZnO (1:3). Further, it was mixed equally with LLDPE pellets to prepare LLDPE/25T75Z with weight % of 5% (LLDPE/TiO2-ZnO film) [Citation29].
2.2. Preparation of study material
LLDPE/TiO2-ZnO film was exposed to UV for one hour and surface sterilized by rinsing with 70% ethanol several times. It was then being immersed entirely in sterile distilled water at 37°C for 12 h.
2.3. Soil biodegradability profile
The LLDPE/TiO2-ZnO film was cut in rectangular with dimensions of 1.35 cm2 and burial in natural soil conditions as shown in Figure . The surface of the soil was dug from four selected natural conditions (compost soil, pond soil, coastline, and landfill). It was placed horizontally inside the holes at a depth of 5 inches below the surface and conducted for 12 months. This method has been described previously [Citation20,Citation30,Citation31]. The weight loss was carefully measured every 3, 6, and 12 months to initial weight before burial. The percentage of weight loss was calculated according to the equation: Weight remaining (%) = 100 × Mt / M0, where M0 and Mt are the masses at the immersion of 0 and time point, respectively [Citation23].
2.4. Hydrolytic degradation profile
LLDPE/TiO2-ZnO film (1.35 cm2) was immersed in Phosphate-buffered saline (PBS) solution at 3, 6, 9, 12, and 15 weeks and 10 mL of PBS solution was used for each sample. The pH values of the PBS solution were monitored every week by an electrolyte-type pH meter. All samples were removed from the PBS solution at the predetermined time point and rinsed gently with distilled water. Then, it was wiped with filter paper to remove surface water followed by drying at room temperature overnight. Subsequently, these films were weighed to determine the mass of dried samples. The weight loss rate was determined by direct measurement according to the previous equation (Section 2.3).
Further, the morphologies of treated LLDPE/TiO2-ZnO film were characterized by field emission electron microscopy (FESEM, Leo Gemini, Carl Zeiss, Oberkochen, Germany) at 5.0 KV and magnification of (500x and 5.0 kx).
2.5. Simulated body fluid (SBF) profile
The ability of LLDPE to release TiO2-ZnO ions was studied by immersion in SBF solution for up to 28 days and an aliquot of solutions was taken out after each period; Day 1, 7, 14, and 28 and kept at −80 0C. SBF solution was prepared according to the standard procedure as described in Table guided by Oyane et al. [Citation32]. The volume of SBF immersion volume was calculated based on the formula: Vs = Sa / 10, Vs is the volume of SBF (mL) and Sa is the apparent surface area of specimens (mm2). The element in the solution was analysed by means of inductively Coupled Plasma Optical Emission spectroscopy (ICP-OES).
Table 1. List of reagents for simulated body fluid solution.
3. Results and discussion
3.1. Soil biodegradability potential
The present work in situ field validation is an extension of our previous in vitro work which discussed on microorganism assisted-biodegradation process [Citation33]. The unique composition of nanocomposite in LLDPE/TiO2-ZnO film might contribute to biodegradability potential however the rate of biodegradability depending on the specific environments condition. Although detail analysis of microbial flora was not carried out, several types of microbial strains have been identified in three different landforms as described from similar studies in Figure . For example, microorganisms, including prokaryotes (bacteria) and eukaryotes (fungi and algae), have known their involvement in microbial mineralisation management techniques. Biological agents such as Pseudomonas, Streptomyces, Rhodococcus, Staphylococcus, Bacillus and Fusarium are common strains identified for PE degradation [Citation34]. In this study, the biodegradability test of study materials LLDPE and LLDPE/TiO2-ZnO film were investigated by buried under different landforms at 3, 6, and 12 months periods.
Figure 2. Microorganisms involved in polymer biodegradation studies in different areas. Locally isolated microbial strains have been identified in different sites for degradation from related articles.

The biodegradability profiles of LLDPE/TiO2-ZnO film in compost and pond soils, in terms of weight loss, were found at 40.89% and 27.95%, respectively (Table ). Furthermore, in the compost soil, the biodegradable potential of LLDPE/TiO2-ZnO film was observed as early as 6 months. Studies have reported compost and pond soils provide unique ecological niches, pH (6.5–8.0) and humidity (45–60%) that support aerobic homogeneous biodegradability activities [Citation35–37].
Table 2. Soil burial profiles of LLDPE and LLDPE/TiO2-ZnO film by the percentage of weight loss.
In the coastline landform, a slight reduction of the initial weight of LLDPE/TiO2-ZnO film was observed at 12 months. The most common microbes found at coastlines are microfungi [Citation38]. In Malaysia, Zakaria et al. [Citation38] found seven genera of microfungi in sandy beach soil in Teluk Aling, Teluk Bahang, Pulau Pinang, Malaysia (Figure ). In high-salt environments, the survival of microbes is limited because sodium chloride (NaCl) salt acts as an antimicrobial agent that disrupts osmotic gradients and challenging for many microorganisms to thrive. This phenomenon supports the observation of reduced biodegradable activities in such environment.
On the other hand, the biodegradability profiles of LLDPE/TiO2-ZnO film in the Landfill were observed as early as 3 months. Present studies were unable to provide data for 12 months due to limitations in controlling the landfill sites. However, the unique ecological condition of each landform provides different LLDPE/TiO2-ZnO film biodegradability potential. Amongst the mentioned microorganisms, previous research identified Pseudomonas sp. isolated from plastic waste dumped sites potentially caused nearly 15–20% weight loss especially involving PE films [Citation39,Citation40]. Our previous in vitro model has observed P. aeruginosa-assisted degradation activities on LLDPE/TiO2-ZnO film via several parameters such as (1) bacterial growth, (2) weight loss reductions and (3) morphological changes. Other factors also played a role in the biodegradability of the film such as the presence of glucose in the medium, boosting the bacterial growth, hence enhancing biodegradability of the film [Citation33]. In general, findings from this study found that LLDPE/TiO2-ZnO film degrade more effectively than bare LLDPE nanocomposite films after a year.
3.2. Hydrolytic degradation potential
The hydrolytic degradation potential was evaluated using in vitro assays for 15 weeks. The pH and weight loss profiles were recorded as in Figure . The pH value was constantly maintained at 7.3 for the first six weeks. However, the pH starts to drop gradually from neutral to acidic pH; 6.43 for LLDPE and 6.94 for LLDPE/TiO2-ZnO film. The degradability activities of these films might contribute to changes in pH conditions possibly attributed to the release of reactive oxygen species (ROS). It was found the photocatalytic activity of TiO2 was better in neutral condition (90%) as compared to acid condition (40%) due to the release of hydroxyl radicals [Citation41]. Upon immersion in aqueous environments, it directly comes in contact with H2O and O2, thereby causing e− / h+ to combine together and generate O2•− and •OH radicals (Equations (1) and (2)). The released O2•− into the surrounding also combines with H+ to generate HO2• radicals and further transforms into hydrogen peroxide (H2O2) with the presence of free e− (Equations (3) and (4)).
(1)
(1)
(2)
(2)
(3)
(3)
(4)
(4)
Figure 3. Hydrolytic degradation profiles of LLDPE and LLDPE/TiO2-ZnO film. (A) pH profiles and (B) weight loss kinetics. Data were recorded by an independent experiment.

These free radicals could directly interact with water-based salt solutions to dissociate LLDPE nanocomposite films. In this test, LLDPE/TiO2-ZnO film degradation activity is higher compared to LLDPE films which complements previous observations in the soil buried test. Further observation on topology characterization by FESEM found that higher deformation holes were observed on LLDPE/TiO2-ZnO film compared to LLDPE films (Figure ). These deformation holes further support the degradability potential of LLDPE/TiO2-ZnO film.
3.3. Biodegradability agents
In previous reports, LLDPE/TiO2-ZnO film had shown promising photodegradation efficiency that was mainly driven by three reactive species which are •OH, O2•− and h+ [Citation42]. These molecules could act as double-sword properties for bactericidal and biodegradable agents in LLDPE/TiO2-ZnO film. Nanocomposite in polymer technology could suppress the electron-hole recombination which led to photocatalytic activities [Citation43]. This improves the capability to absorb more oxygen onto surfaces and further release •OH which might contribute to the degradability potential in LLDPE films. A part of hydrophilic properties, the generation of •OH radicals by nanocomposites also present as the strongest oxidizing which is associated with LLDPE film’s lifetime [Citation44]. These activities suggested might be a surge in aqueous mediums or high moisture and oxygen content, especially in soil [Citation45,Citation46].
Figure 4. Topology analysis of LLDPE/TiO2-ZnO film by FESEM after hydrolytic degradation treatment. Hydrolytic biodegradation activity observed on bare LLDPE and LLDPE/25T75Z/5% nanocomposites films.

Polymer degradability potential might be directly affected by hydrolytic reactions [Citation47,Citation48]. There is a possibility of the weight in LLDPE/TiO2-ZnO film showing a greater reduction in weight in soil buried and hydrolytic degradation test earlier. The loss of h+ due to the polarity of water and hydroxide (OH-) ions cause the loss in the C–H bond in LLDPE films [Citation49]. After direct exposure to moisture, it creates acid groups which reduce the pH. In neutral conditions, a study done by Diantoro et al. Citation2018 found more release of •OH from the reaction of TiO2 with water molecules [Citation41]. When the pH was reduced, this caused the conditions to become slightly acidic, and excessive h+ was found in the solutions. Excessive h+ reduces the generation of •OH and will counter back to H2O molecules when react together. It can be clearly seen in Figure , there is no single hole can be observed on the surface of untreated LLDPE films. However, the in LLDPE/TiO2-ZnO film showed an obvious hole after being treated for 15 weeks. This formation of holes will help to speed up the degradation of LLDPE films and thus, result in the reduction of weight in LLDPE/TiO2-ZnO film.
Further analysis of ion release profiles by SBF supported the above findings. As shown in Figure , LLDPE/25T75Z films showed continuous Zn2+ ion release in SBF solution since day 1 until day 28. In conclusion, the nanocomposite in LLDPE/TiO2-ZnO film could provide the capability to capture holes and electrons more effectively which is crucial in polymer degradation activity. The release of a high number of holes and electrons from LLDPE/TiO2-ZnO film adsorbs more oxygen and water molecules, thereby improving ROS and free •OH generation. The enhancement of degradation rate by a photocatalytic activity also produces CO2 as a side product from the reaction and is used as additional energy for further microbial biodegradation.
Figure 5. Zinc ion released profile in SBF for 28 days. Graph of mean zinc ion released in SBF solution exposed to LLDPE/25T75Z/5% nanocomposite films from two independent ICP-OES analyses. Results are expressed as mean ± standard deviation.

Nanocomposites in LLDPE/25T75Z/5% nanocomposite films could enhance microbial growth in a nutrient-rich environment [Citation50,Citation51]. In the previous report, microbes like P. aeruginosa have shown could assist in the degradation of LLDPE/25T75Z/5% nanocomposite films after 30 days [Citation20]. Other factors such as temperature, humidity, oxygen, pH, light, and water in the environment are also critical in polymer degradation activities [Citation26–28]. It was clear that the presence of water molecules in a medium or moisture could cause a hydrolysis reaction between TiO2-ZnO nanocomposite by the release of •OH and carbon dioxide (CO2) molecules influencing the microbial activities. These molecules will be used as an additional food source for microorganisms and help enzymatic reactions into metabolic products such as water, CO2, methane (CH4) that are significant in the biodegradation process [Citation52].
4. Conclusion
The finding from this study suggests that the nanocomposite present in LLDPE polymer could enhance the biodegradability potential of LLDPE/25T75Z/5% nanocomposite films.
High moisture content could assist the microbial biodegradation process of LLDPE/25T75Z/5% nanocomposite films.
The findings from this study provide knowledge for potential improvement of biodegradable polymers for healthcare-related products and waste management technology.
Acknowledgement
Rabiatul Basria S. M. N. Mydin (RM) is the principal investigator who contributed to the concept, idea, experimental design, and writing process and gave final approval of this paper for publication.
Disclosure statement
No potential conflict of interest was reported by the author(s).
Data availability statement
All data and set of results are applicable upon requests from the corresponding author on a reasonable request.
Additional information
Funding
References
- Gunatillake PA, Adhikari R. Nondegradable synthetic polymers for medical devices and implants. In: Biosynthetic polymers for medical applications; 2016. p. 33–62. doi:10.1016/b978-1-78242-105-4.00002-x
- Maitz MF. Applications of synthetic polymers in clinical medicine. Biosurf Biotribol. 2015;1(3):161–176. doi:10.1016/j.bsbt.2015.08.002
- Teo AJ, Mishra A, Park I, et al. Polymeric biomaterials for medical implants and devices. ACS Biomater Sci Eng. 2016;2(4):454–472. doi:10.1021/acsbiomaterials.5b00429
- Lyu S, Untereker D. Degradability of polymers for implantable biomedical devices. Int J Mol Sci. 2009;10(9):4033–4065. doi:10.3390/ijms10094033
- Lendlein A, Behl M, Hiebl B, et al. Shape-memory polymers as a technology platform for biomedical applications. Expert Rev Med Devices. 2010;7(3):357–379. doi:10.1586/erd.10.8
- Serrano MC, Ameer GA. Recent insights into the biomedical applications of shape-memory polymers. Macromol Biosci. 2012;12(9):1156–1171. doi:10.1002/mabi.201200097
- Su CH, Chen HL, Ju SP, et al. The mechanical behaviors of polyethylene/silver nanoparticle composites: an insight from molecular dynamics study. Sci Rep. 2020;10(1):1–14. doi:10.1038/s41598-019-56847-4
- Khanam PN, Al Maadeed MAA. Processing and characterization of polyethylene-based composites. Adv Manuf Polym Compos Sci. 2015;1(2):63–79. doi:10.1179/2055035915Y.0000000002
- Malpass DB. Introduction to industrial polyethylene: properties, catalysts, and processes. London: John Wiley & Sons; 2010.
- Paxton NC, Allenby MC, Lewis PM, et al. Biomedical applications of polyethylene. Eur Polym J. 2019;118:412–428. doi:10.1016/j.eurpolymj.2019.05.037
- Dai S, Li Z, Meng Y, et al. A comprehensive review of synthesis, properties and modification of several common polyethylenes. In: Eighth international conference on energy materials and electrical engineering (ICEMEE 2022); 2023 Apr. SPIE. Vol. 12598. p. 830–839.
- Chamanee G, Sewwandi M, Wijesekara H, et al. Global perspective on microplastics in landfill leachate; occurrence, abundance, characteristics, and environmental impact. Waste Manage. 2023;171:10–25. doi:10.1016/j.wasman.2023.08.011
- Albertsson AC, Karlsson S. Polyethylene degradation and degradation products; 1990.
- Suresh B, Maruthamuthu S, Palanisamy N, et al. Investigation on biodegradability of polyethylene by bacillus cereus strain Ma-Su isolated from compost soil. Int Res J Microbiol. 2011;2(2):292–302.
- Khabbaz F, Albertsson AC. Rapid test methods for analyzing degradable polyolefins with a pro-oxidant system. J Appl Polym Sci. 2001;79(12):2309–2316. doi:10.1002/1097-4628(20010321)79:12<2309::AID-APP1038>3.0.CO;2-E
- Lim BKH, San Thian E. Biodegradation of polymers in managing plastic waste – a review. Sci Total Environ. 2022;813:151880. doi:10.1016/j.scitotenv.2021.151880
- Kulkarni A, Dasari H. Current status of methods used in degradation of polymers: a review. MATEC Web Conf. 2018;144:02023. doi:10.1051/matecconf/201814402023
- Zhou Q, Song H, Sun T, et al. Cataluminescence on 2D WS2 nanosheets surface for H2S sensing. Sens Actuators B. 2022;353:131111. doi:10.1016/j.snb.2021.131111
- Su Y, Liu Y, Li W, et al. Sensing–transducing coupled piezoelectric textiles for self-powered humidity detection and wearable biomonitoring. Mater Horiz. 2023;10(3):842–851. doi:10.1039/D2MH01466A
- Borelbach P, Kopitzky R, Dahringer J, et al. Degradation behavior of biodegradable man-made fibers in natural soil and in compost. Polymers. 2023;15(13):2959. doi:10.3390/polym15132959
- Meng K, Teng Y, Ren W, et al. Degradation of commercial biodegradable plastics and temporal dynamics of associated bacterial communities in soils: a microcosm study. Sci Total Environ. 2023;865:161207. doi:10.1016/j.scitotenv.2022.161207
- Harun NH, Mydin RBS, Sreekantan S, et al. LLDPE/TiO2-ZnO nanocomposite films induces transitory oxidative stress response on human fibroblast and blood cell lines models. J Biomim Biomater Biomed Eng. 2023;61:77–91. doi:10.4028/p-2aa27K
- Harun NH, Mydin RBS, Sreekantan S, et al. In vitro bio-interaction responses and hemocompatibility of nano-based linear low-density polyethylene polymer embedded with heterogeneous TiO2/ZnO nanocomposites for biomedical applications. J Biomater Sci Polym Ed. 2021;32(10):1301–1311. doi:10.1080/09205063.2021.1916866
- Harun NH, Mydin RBS, Sreekantan S, et al. Bactericidal capacity of a heterogeneous TiO2/ZnO nanocomposite against multidrug-resistant and non-multidrug-resistant bacterial strains associated with nosocomial infections. ACS Omega. 2020;5(21):12027–12034. doi:10.1021/acsomega.0c00213
- Harun NH, Mydin RBSN, Sreekantan S, et al. The bactericidal potential of LLDPE with TiO2/ZnO nanocomposites against multidrug resistant pathogens associated with hospital acquired infections. J Biomater Sci Polym Ed. 2020;31(14):1757–1769. doi:10.1080/09205063.2020.1775759
- Basiron N, Sreekantan S, Jit Kang L, et al. Coupled oxides/LLDPE composites for textile effluent treatment: effect of neem and PVA stabilization. Polymers. 2020;12(2):394. doi:10.3390/polym12020394
- Harun NH, Mydin RBSN, Noordin SS, et al. Hemocompatibility profiles of LLDPE with TiO2/ZnO nanocomposites for biomedical application according to ISO 10993-4 And ASTM 756-00 (2000) guidelines. BioNanoScience. doi:10.1007/s12668-023-011182-2
- Harun NH, Mydin RBS, Sreekantan S, et al. In vitro biodegradation evaluation of linear low density polyethylene embedded with TiO2/ZnO couple oxides. IOP Conf Ser Mater Sci Eng. 2020;932(1):12032. doi:10.1088/1757-899X/932/1/012032
- Li H, Chang J, Cao A, et al. In vitro evaluation of biodegradable poly (butylene succinate) as a novel biomaterial. Macromol Biosci. 2005;5(5):433–440.
- Sintim HY, Bary AI, Hayes DG, et al. In situ degradation of biodegradable plastic mulch films in compost and agricultural soils. Sci Total Environ. 2020;727:138668. doi:10.1016/j.scitotenv.2020.138668
- Raghavan D. Characterization of biodegradable plastics. Polym Plast Technol Eng. 1995;34(1):41–63. doi:10.1080/03602559508017212
- Oyane A, Kim HM, Furuya T, et al. Preparation and assessment of revised simulated body fluids. J Biomed Mater Res A. 2003;65(2):188–95.
- Harun NH, Mydin RBS, Sreekantan S, et al. In vitro biodegradation evaluation of linear low density polyethylene embedded with TiO2/ZnO couple oxides. IOP Conf Ser Mater Sci Eng. 2020;932(1):12032. doi:10.1088/1757-899x/932/1/012032
- Pathak VM. Review on the current status of polymer degradation: a microbial approach. Bioresour. 2017;4(1):1–31.
- Vaverková MD, Adamcová D, Hermanová S, et al. Ecotoxicity of composts containing aliphatic-aromatic copolyesters. Pol J Environ Stud. 2015;24(4):1497–1505. doi:10.15244/pjoes/31227
- Herman B, Biczak R, Rychter P, et al. Degradation of selected synthetic polyesters in the industrial compost heap: effect on compost properties and phytotoxicity. Proc ECOpole. 2010;4(1):133–140.
- Ishigaki T, Sugano W, Nakanishi A, et al. The degradability of biodegradable plastics in aerobic and anaerobic waste landfill model reactors. Chemosphere. 2004;54(3):225–233. doi:10.1016/S0045-6535(03)00750-1
- Zakaria L, Yee TL, Zakaria M, et al. Diversity of microfungi in sandy beach soil of Teluk Aling, Pulau Pinang. Trop Life Sci Res. 2011;22(1):71.
- Balasubramanian V, Natarajan K, Hemambika B, et al. High-density polyethylene (HDPE)-degrading potential bacteria from marine ecosystem of Gulf of Mannar, India. Lett Appl Microbiol. 2010;51(2):205–211.
- Kyaw BM, Champakalakshmi R, Sakharkar MK, et al. Biodegradation of low density polythene (LDPE) by Pseudomonasspecies. Indian J Microbiol. 2012;52(3):411–419.
- Diantoro M, Kusumaatmaja A, Triyana K. Study on photocatalytic properties of TiO2 nanoparticle in various pH condition. J Phys Conf Ser. 2018;1011(1):12069.
- Saharudin KA, Sreekantan S, Basiron N, et al. 3ZnO/TiO2 coupled oxides LLDPE nanocomposite: effect of various weight percent of sol-gel synthesized catalyst on structural and bacteriostatic activity against S. aureus and E. coli. Biomed J Sci Tech Res. 2018;8(4):1–10.
- Pant B, Ojha GP, Kuk YS, et al. Synthesis and characterization of ZnO-TiO2/carbon fiber composite with enhanced photocatalytic properties. Nanomaterials. 2020;10(10):1960. doi:10.3390/nano10101960
- Sangareswari M, Sundaram MM. Development of efficiency improved polymer-modified TiO2 for the photocatalytic degradation of an organic dye from wastewater environment. Appl Water Sci. 2017;7(4):1781–1790. doi:10.1007/s13201-015-0351-6
- Luo YB, Wang XL, Wang YZ. Effect of TiO2 nanoparticles on the long-term hydrolytic degradation behavior of PLA. Polym Degrad Stab. 2012;97(5):721–728.
- Tu-morn M, Pairoh N, Sutapun W, et al. Effects of titanium dioxide nanoparticle on enhancing degradation of polylactic acid/low density polyethylene blend films. Mater Today Proc. 2019;17:2048–2061.
- Antunes A, Popelka A, Aljarod O, et al. Effects of rutile–TiO2 nanoparticles on accelerated weathering degradation of poly(lactic acid). Polymers. 2020;12(5):1096. doi:10.3390/polym12051096
- Zaaba NF, Jaafar M. A review on degradation mechanisms of polylactic acid: hydrolytic, photodegradative, microbial, and enzymatic degradation. Polymer Eng Sci. 2020;60(9):2061–2075. doi:10.1002/pen.25511
- Massey S, Adnot A, Rjeb A, et al. Action of water in the degradation of low-density polyethylene studied by X-ray photoelectron spectroscopy. Express Polym Lett. 2007;1(8):506–511. doi:10.3144/expresspolymlett.2007.72
- Kapri A, Zaidi MGH, Goel R. Implications of SPION and NBT nanoparticles upon in-vitro and in-situ biodegradation of LDPE film. J Microbiol Biotechnol 2010;20(6):1032–1041. doi:10.4014/jmb.0912.12026
- Venubabu Thati A, Roy S, Prasad MA, et al. Nanostructured zinc oxide enhances the activity of antibiotics against Staphylococcus aureus. J Biosci Tech. 2010;1(2):64–69.
- Mohan K. Microbial deterioration and degradation of polymeric materials. J Biochem Technol. 2011;2(4):210–215.