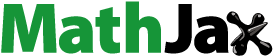
Abstract
Novel bidentate chelator, 2-(((1H-1,2,4-triazol-5-yl)imino)methyl)-4-(o-tolyldiazenyl)phenol was employed for designing and preparation of transition metallic complexes. The synthetics were characterized based on various analytical, spectral, and theoretical tools. Based on the various analysis data, the azo-Schiff base chelator (H2L) linked with metallic cations as a neutral/uni-negative bidentate chelator via the protonated/deprotonated hydroxyl oxygen and azomethine nitrogen atoms adopted a distorted octahedral, tetrahedral, or square planar geometry. The mass spectroscopy confirmed that the molecular weights of azo-Schiff base and some of its complexes closely match with that of the estimated m/z values. Additionally, the structure of the azo-Schiff base ligand and some metallic complexes were studied by Density functional theory (DFT) calculations to assess the molecular orbitals (LUMO&HOMO) as well as the molecules electrostatic potential (MEP) to complete the picture. The synthetics were evaluated for their in vitro activity against Escherichia coli, Aspergillus niger, and Bacillus subtilis. According to this research, unbound ligand molecules are inactive while their metallic complexes have low to moderate activity.
1. Introduction
One of the treasures of discovering new chemical compounds with biological and pharmacological activity is coordination chemistry. It is a brand-new, highly beneficial source of synthetic and semi-synthetic pharmaceuticals that enhances the activity of drugs to overcome the pathogens resistant [Citation1]. The azo-Schiff base ligand was created when azo-dyes moiety (-N=N-) and Schiff-base with functional azomethine linkage (-C=N-) are combined [Citation2]. The physiochemical and biological features of this flexibility class of chelators and their associated chelated complexes are improved by the inclusion of two active moieties in a single molecule [Citation3]. The therapeutic properties of azo-compounds was discovered in Prontosil, sulphonamide associated azo-dye, which used as a medication against microbial infections [Citation4,Citation5]. The biological properties of azo-compounds have made them a focus of attention due to their potential as fungicidal [Citation6–9], anti-oxidant [Citation10], anticancer [Citation11], anti-inflammatory [Citation6,Citation12], antiviral (HIV) [Citation13], and bacteriostatic agents [Citation6,Citation7]. The Schiff bases with distinctive azomethine group were also aroused the attention of many researchers because of their biological activities and excellent therapeutic applications as well as their chelating properties [Citation14]. It has previously been reported that the presence of the azomethine moiety(-C=N-) is primarily responsible for their biological activity [Citation15–17]. Consequently, compounds with an azomethine moiety represent a significant class of compounds as pharmaceutical and therapeutic agents with a broad variety of pharmacological effects with analgesic [Citation15], antiproliferative, anticancer [Citation18–22], anti-inflammatory [Citation15,Citation23], antiepileptic [Citation24], antitumor [Citation25–28], antibacterial [Citation29–33], antidepressant [Citation34], antifungal [Citation30–33], anti-oxidant [Citation19,Citation25–27], antidiabetic [Citation35,Citation36], enzyme & protein inhibitor [Citation23] herbicidal [Citation27,Citation37], and antiviral viruses (HIV, influenza A, COVID-19 and a21, denovirus) [Citation26,Citation27,Citation38]. Deswal et al. showed that the Co(II), Ni(II), Cu(II) and Zn(II) complexes of 2-(((3-mercapto-5-(pyridin-3-yl)-4H-1,2,4-triazol-4-yl)imino)methyl)-4-nitrophenol have moderate to good alpha-amylase and alpha-glucosidase inhibitory effect [Citation39]. Nowadays, Schiff base metal complexes have employed as precursors and effective low-cost production techniques for preparing metal oxide nanoparticles by thermal decomposition [Citation40]. Schiff bases are also an significant class of compounds that are highly instrumental in formation of complexes as well as act as sensors for various metal ions [Citation41,Citation42]. Recently, metal complexes of Schiff base assembled from natural products which used for coatings displayed antibacterial-renew dual-function anti-biofouling activity [Citation43] and used as catalysis in the several reaction as their action as catalysts in reduction of nitro aromatic compounds [Citation42], oxidation of primary and secondary alcohols [Citation44] as well as in the Suzuki–Miyaura and Mizoroki-Heck cross-coupling reactions [Citation45]. The current work studies the novel metallic complexes of azo-Schiff base incorporating both the azomethine (C=N-) and Azo- (C–N=N–C) linkages. It aimed to synthesis of VO2+, Fe3+, Ru3+, Co2+, Mn2+, Ni2+, Cu2+ Zn2+ and complexes of new azo-Schiff base namely, 2-(((1H-1,2,4-triazol-5-yl)imino)methyl)-4-(o-tolyldiazenyl)phenol (H2L, 1) (Scheme ). Consequently, physio-chemical, and analytic techniques such NMR, UV-Vis, FT-IR, elemental analyzer, ESI-MS, molar conductance, magnetic moment, and thermal measurements as well as theoretical calculations were used to characterize the structure of the produced compounds. The geometry of the chelator and its metallic complexes was examined auxiliary to the density functional theory (DFT) calculations at the B3LYP/6-311++G(d, p) levels. To complete the picture, we assessed not only the overall energy but also the molecular orbitals (LUMO&HOMO) and the molecular electrostatic potential (MEP). Additionally, the in vitro antifungal and antibacterial activities of the chelator and its complexes was examined to discover novel, highly effective antimicrobial compounds to address the issue of antimicrobial resistance that most commonly occurs with the usage of medications.
2. Materials and methods
The DMSO, absolute ethanol 1H-1,2,4-triazol-5-amine, and metal salts, (assay = 98.0–99.9%) were bought from Merck and employed with no extra purification. A published method was used to prepare 2-hydroxy-5-(o-tolyldiazenyl)benzaldehyde [Citation46].
2.1. Apparatus
In Cairo University's Micro-Analytical Laboratory, Egypt, the elements (N, H, and C) for the produced compounds were analyzed, while the metal contents (VO2+, Fe3+, Ru3+, Co2+, Mn2+, Ni2+, Cu2+, Zn2+) and Cl- ions were assessed using conventional analytical techniques [Citation47,Citation48]. On an infrared spectrophotometer (Perkin–Elmer-1430) at National research centre, Egypt, KBr plates were employed to measure the IR spectra over of 400–4000 cm−1 range. On a SHMADZU 2600 spectrophotometer, the electronic absorption spectrum was measured using 1-cm quartz cell over of 200–1100 nm range in DMSO at university of Jeddah. On mass spectrometer (JEUL JMS-AX-500), at National research centre, Egypt, the mass spectrometry was determined while on a JEOL EAC-400 spectrometer at Qassim University, Saudi Arabia, the NMR spectra was measured in DMSO-d6. The complexes TG analysis was measured on a Perkin Elmer 7 thermal analyzer over a range of 25–800°C at a heating rate of 10 °C/min at National research centre, Egypt. At university of Jeddah, the magnetic susceptibility was recorded at 25°C using a Gouy Matthey’s Balance and computed by a published equation [Citation49]. Tacussel type CD6NG conductivity bridge was used to test the complexes’ molar conductance (10−3 M/DMSO), and the published equation was used to compute it [Citation50]. Unfortunately, there were countless failed attempts to get a single crystal. The clarity of prepared compounds has been verified by TLC utilizing various mobile phases.
2.2. Synthesis of azo-Schiff base ligand (H2L)
The ligand, 2-(((1H-1,2,4-triazol-5-yl)imino)methyl)-4-(o-tolyldiazenyl)phenol (H2L, 1) was prepared by refluxing a mixture of 2-hydroxy-5-(o-tolyldiazenyl)benzaldehyde (240 mg, 1 mmol, 30 mL of EtOH) and 1H-1,2,4-triazol-5-amine (84 mg, 1 mmol, 50 mL EtOH) in presence of 0.1 mL glacial CH3COOH for five hours. Once the coloured solid had formed, the reaction mixture was allowed to cool at 25°C before being separated, washed with EtOH, and dried in a vacuum to produce azo-Schiff base (H2L, 1) (271.7 mg, 0.887 mmol, 88.7%) (Figure ) as a yellowish green solid, (Melting point) m.p. = 240°C. Anal. (Elemental analysis) for C16H14N6O (306.3 g /mol): Found (calcd.) %C 62.46 (62.74), %H 4.42 (4.61), %N 27.37 (27.44). FT-IR (KBr, cm−1), 3398 ν(22O-H33), 3233 ν(30N-H32), 3080w, 3000, 2964w, 2888w ν(C–H), 1660 ν(23C = N24), 1605 ν(26C = N29)&ν(27C = N31), 1475 ν(11N = N12), 1267 ν(20C-O22), 1000 ν(29N-N31). 1H-NMR (400 MHz, DMSO-d6): δ(ppm) = 14.37, 8.35 (s, 1H, NH30), 12.619 (s, 1H, OH33),7.96 (d, 2H, C-H8&9), 8.395 (m, 1H, CH7&10), 7.31 (d, 1H, CH21), 8.819 (d, 1H, CH17), 9.13 (s, H, CH19), 9.56 (s, H, CH25), 8.173 (s, H, CH28), 2.67(s, 3H, CH3). 13C-NMR (90 Mhz, DMSO-d6): δ(ppm) = 134.17 (C1&5), 122.42 (C2&4), 150.63 (C3), 140.42 (C6), 144.12 (C13), 128.29 (C14), 125.51 (C15), 113.79 (C16), 117.21 (C18), 166.31 (C20), 163.42 (C23), 159.58 (C26), 146.6 (C27), 27.45 (C34).
2.3. General procedure for the synthesis of metal complexes
VO2+, Fe3+, Ru3+, Co2+, Mn2+, Ni2+, Cu2+, Zn2+, and complexes were synthesized by refluxing an equimolar amounts of the following salts’ ethanolic solution (50 mL): VOSO4·3H2O (217.0 mg), FeCl3·6H2O (271.3 mg), RuCl3·3H2O (261.5 mg), Co(CH3COO)2·4H2O (249.1 mg), Mn(CH3COO)2·4H2O (245.1 mg), Ni(CH3COO)2·4H2O (248.8 mg), Cu(CH3COO)2·2H2O (199.7 mg), CuCl2.2H2O (170.5 mg), Cu(NO3)2.3H2O (241.6 mg), CuSO4·5H2O (249.7 mg), Zn(CH3COO)2·2H2O (219.5 mg), and UO2(CH3COO)2·2H2O (424.2 mg) with 2-(((1H-1,2,4-triazol-5-yl)imino)methyl)-4-(o-tolyldiazenyl)phenol (H2L, 1) (306.3 mg, 30 mL of EtOH). For four hours, the refluxing was done while being stirred. After heating and rinsing many times with hot EtOH, the resulting solid-colored complexes separated out and were eventually dried under vacuum over P4O10.
2.3.1. VO2+-complex (2)
Color: light brown, yield (50.6%), mp = 266 oC, molar conductance (Λm) = 34.9 ohm−1cm2mol−1. Anal. for [VO(H2L)SO4(H2O)2], C16H18VSN6O8, (505.36 g/mol): Found(calcd.) %C 38.52(38.13), %H 3.31(3.59), %N 17.01(16.63), %V 9.77(10.08). FT-IR (KBr, cm−1), 3488 ν(22O-H33), 3422 ν(H2O), 3188 ν(30N-H32), 3046w, 2920, 2875w ν(C–H), 1633 ν(23C = N24), 1601 ν(26C = N29)&ν(27C = N31), 1474 ν(11N = N12), 1319 ν(20C-O22), 1022 ν(29N-N31) 1155, 1061 ν(SO4), 970 (O = V), 577 (22O→V), 491 ν(24N→V).
2.3.2. Fe3+-complex (3)
Color: reddish brown, yield (56.4%), mp = 280 oC, Λm = 22.2 ohm−1cm2mol−1. Anal. for [Fe(HL)Cl2(H2O)2].2H2O, C16H21FeN6O5Cl2, (504.13 g/mol): Found (calcd.) %C 38.09(38.12), %H 3.98(4.20), %N 16.47(16.67), %Cl 13.87 (14.06), %Fe 10.88 (11.08) FT-IR (KBr, cm−1), 3400 ν(H2O), 3180 ν(30N-H32), 3090w, 2945, 2875w ν(C–H), 1611 ν(23C = N24), 1598 ν(26C = N29)&ν(27C = N31), 1468 ν(11N = N12), 1309 ν(20C-O22), 1017 ν(29N-N31) 589 (22O-Fe), 488 ν(24N→Fe).
2.3.3. Ru3+-complex (4)
Color: brown, yield (77.8%), mp > 300 oC, (Λm) = 14.5 ohm−1cm2mol−1. Anal. for [Ru(HL)Cl2(H2O)2], C16H17RuN6O3Cl2, (513.32 g/mol): Found (calcd.) %C 37.40(37.44), %H 3.09(3.34), %N 16.43(16.37), %Cl 13.77 (13.56), %Ru 19.34 (19.69). FT-IR (KBr, cm−1), 3432 ν(H2O), 3309 ν(30N-H32), 3095w, 2995, 2870w ν(C–H), 1640 ν(26C = N27), 1601 ν ν(26C = N29)&ν(27C = N31), 1470 ν(11N = N12), 1306 ν(20C-O22), 1012 ν(29N-N31) 600 (22O-Ru), 481 ν(24N→Ru).
2.3.4. Co2+-complex (5)
Color: reddish brown, yield (67.6%), mp = 281 oC, Λm = 12.9 ohm−1cm2mol−1. Anal. for [Co(HL)(O)COCH3(H2O)3], C18H22CoN6O6, (477.34 g/mol): Found(calcd.) %C 45.00(45.29), %H 5.03(4.65), %N 17.46(17.61), %Co 12.09(12.35). FT-IR (KBr, cm−1), 3407 ν(H2O), 3200 ν(30N-H32), 3060w, 2923, 2850w ν(C–H), 1616 ν(23C = N24), 1600 ν(26C = N29)&ν(27C = N31), 1476 ν(11N = N12), 1292 ν(20C-O22), 1009 ν(29N-N31) 531 (22O-Co), 469 ν(24N→Co), 1558/1352(206) νs(CH3COO)/νas(CH3COO)(Δ).
2.3.5. Mn2+-complex (6)
Color: brown, yield (66.7%), mp > 300 oC, Λm = 4.4 ohm−1cm2mol−1. Anal. for [Mn(HL)(O)COCH3(H2O)], C18H18MnN6O4, (437.32 g/mol): Found(calcd.) %C 49.13(49.44), %H 3.87(4.15), %N 18.89(19.22), %Mn 12.61(12.56). FT-IR (KBr, cm−1), 3420 ν(H2O), 3150 ν(30N-H32), 3060w, 2921, 2850w ν(C–H), 1613 ν(26C = N27), 1600 ν(26C = N29)&ν(27C = N31), 1480 ν(11N = N12), 1290 ν(20C-O22), 1014 ν(29N-N31) 505 (22O-Mn), 491 ν(24N→Mn), 1542/1339(203) νs(CH3COO)/νas(CH3COO)(Δ).
2.3.6. Ni2+-complex (7)
Color: light brown, yield (71.1%), mp = 276 oC, Λm = 14.9 ohm−1cm2mol−1. Anal. for [Ni(HL)(O)COCH3(H2O)], C18H18NiN6O4, (441.07 g/mol): Found(calcd.) %C 48.86(49.02), %H 4.02(4.11), %N 18.85(19.05), %Ni 13.06(13.31). FT-IR (KBr, cm−1), 3441 ν(H2O), 3209 ν(30N-H32), 3027w, 2920, 2861w ν(C–H), 1635 ν(23C = N24), 1607 ν(26C = N29)&ν(27C = N31), 1465 ν(11N = N12), 1303 ν(20C-O22), 1015 ν(29N-N31) 500 (22O-Co), 482 ν(24N→Co), 1587/1384(203) νs(CH3COO)/νas(CH3COO)(Δ).
2.3.7. Cu2+-complex (8)
Color: dark green, yield (76.1%), mp = 270 oC, Λm = 7.1 ohm−1cm2mol−1. Anal. for [Cu(HL)(O)COCH3(H2O)], C18H18CuN6O4, (455.90 g/mol): Found(calcd.) %C 48.95(48.48), %H 3.89(4.07), %N 14.16(14.35), %Cu 13.99(14.25). FT-IR (KBr, cm−1), 3420 ν(H2O), 3213 ν(30N-H32), 3029w, 2920, 2858w ν(C–H), 1612 ν(23C = N24), 1600 ν(26C = N29)&ν(27C = N31), 1482 ν(11N = N12), 1288 ν(20C-O22), 1014 ν(29N-N31) 541 (22O-Cu), 481 ν(24N→Cu), 1569/1351(218) νs(CH3COO)/νas(CH3COO)(Δ).
2.3.8. Cu2+-complex (9)
Color: dark green, yield (66.5%), mp = 290 oC, Λm = 11.2 ohm−1cm2mol−1. Anal. for [Cu(HL)Cl(H2O)], C16H15CuN6O2Cl, (422.3 g/mol): Found(calcd.) %C 45.23(45.50), %H 3.35(3.58), %N 19.58(19.90), %Cu 14.95(15.05). FT-IR (KBr, cm−1), 3413 ν(H2O), 3162 ν(30N-H32), 3030w, 2921, 2862w ν(C–H), 1627 ν(26C = N27), 1607 ν(26C = N29)&ν(27C = N31), 1477 ν(11N = N12), 1304 ν(20C-O22), 1013 ν(29N-N31) 511 (22O-Cu), 456 ν(24N→Cu).
2.3.9. Cu2+-complex (10)
Color: dark olive, yield (73%), mp = 300 oC, Λm = 21.1 ohm−1cm2mol−1. Anal. for [Cu(HL)NO3(H2O)], C16H15CuN7O5, (448.9 g/mol): Found(calcd.) %C 42.50(42.81), %H 3.27(3.37), %N 21.55(21.84), %Cu 14.01(14.16). FT-IR (KBr, cm−1), 3419 ν(H2O), 3244 ν(30N-H32), 3044w, 2931, 2854w ν(C–H), 1617 ν(26C = N27), 1601 ν(29C = N32)&ν(30C = N34), 1478 ν(11N = N12), 1283 ν(20C-O22), 1021 ν(29N-N31) 551 (22O-Cu), 491 ν(24N→Cu), 1408, 1363, 966 ν (NO3)(Δ = 45).
2.3.10. Cu2+-complex (11)
Color: dark olive, yield (61.8%), mp=280 oC, Λm = 27.5 ohm−1cm2mol−1. Anal. for [Cu(H2L)SO4(H2O)] C16H16CuSN6O6, (483.95 g/mol): Found(calcd.) %C 39.46(39.71), %H 3.01(3.33), %N 17.21(17.37), %S 6.18(6.62), %Cu 12.89(13.13). FT-IR (KBr, cm−1), 3407 ν(H2O), 3180 ν(30N-H32), 3027w, 2940, 2875w ν(C–H), 1629 ν(23C = N24), 1609 ν(26C = N29)&ν(27C = N31), 1468 ν(11N = N12), 1324 ν(20C-O22), 1011 ν(29N-N31) 533 (22O-Cu), 461 ν(24N→Cu), 1154, 1053 ν(SO4).
2.3.11. Zn2+-complex (12)
Color: light brown, yield (61.7%), mp = 284 oC, Λm = 5.4 ohm−1cm2mol−1, Anal. for [Zn(HL)(O)COCH3(H2O)3].4H2O C18H30ZnN6O10, (555.85 g/mol): Found(calcd.) %C 38.71(38.90), %H 5.24(5.44), %N 15.15(15.12), %Zn 11.55(11.76). FT-IR (KBr, cm−1), 3410 ν(H2O), 3225 ν(30N-H32), 3060w, 2937, 2855w ν(C–H), 1610 ν(23C = N24), 1600 ν(26C = N29)&ν(27C = N31), 1465 ν(11N = N12), 1319 ν(20C-O22), 1016 ν(29N-N31) 546 (22O-Zn), 474 ν(24N→Zn), 1575/1388(188) νs(CH3COO)/νas(CH3COO)(Δ). 1H-NMR (DMSO-d6, 400 MHz,): δ(ppm) = 8.62 (s, 1H, NH30), 7.88 (d, 2H, C-H8&9), 8.12 (m, 1H, CH7&10), 7.55 (d, 1H, CH21), 8.71 (d, 1H, CH17), 8.92 (s, H, CH19), 9.00 (s, H, CH25), 7.86 (s, H, CH28), 2.57(s, 3H, CH3), 1.98 (s, 3H, CH3COO).
2.3.12. UO22+-complex (13)
Color: light brown, yield (62.8%), mp = 281 oC, Λm = 7.1 ohm−1cm2mol−1, Anal. for [UO2(HL)(O)COCH3(H2O)3].6H2O, C18H34UN6O14, (796.53 g/mol): Found(calcd.) %C 27.04(27.14), %H 4.16(4.30), %N 10.43(10.55), %U 29.74(29.88). FT-IR (KBr, cm−1), 3400 ν(H2O), 3131 ν(30N-H32), 3077w, 2956, 2866w ν(C–H), 1622 ν(23C = N24), 1603 ν(26C = N29)&ν(27C = N31), 1473 ν(11N = N12), 1309 ν(20C-O22), 1017 ν(29N-N31) 589 (22O-U), 499 ν(24N→U), 963 (O = U = O), 1568/1366(202) νs(CH3COO)/νas(CH3COO)(Δ).
2.4. Theoretical calculations
Based on Density Functional Theory (DFT), quantum chemical parameters was determined by Gaussian 09 Rev. A.02-MSP program [Citation51]. The software Gauss View Rev. 5.0.9 was employed to visualize the resulted data [Citation52]. The optimization of dihydrazone-oxime’s molecular structure in ground state was done based on DFT with Beckee3eLeeeYangeParr (B3LYP) functional levels with basis set (6-311G+(d,p)) [Citation53,Citation54].
2.5. Microbicidal evaluation
The assessment of in-vitro antifungal and antibacterial activities of the produced compounds (1–13) was performed utilizing agar well diffusion technique versus different fungal and bacterial species as Escherichia coli, (E. coli), Aspergillus niger (A. niger), and Bacillus subtilis (B. subtilis) [Citation55,Citation56]. The fungus and bacterium species were cultivated at pH 7.4 on Czapek Dox's agar (CDA), and Mueller-Hinton agar correspondingly. For A. niger, the agar plates were incubated at 28°C for 4 days, but for bacteria at 37°C for 24 h. A positive control amphotericin B (AmB) for fungi and Tetracycline (Tc) for bacteria, as well as negative (DMSO; 2% v/v) were also involved for comparison. The presence of inhibitory areas reflects the tested drugs’ antimicrobial activity. However, the three independent replicates’ average was determined and the activity index was calculated [Citation25].
3. Results and discussion
The ligand, 2-(((1H-1,2,4-triazol-5-yl)imino)methyl)-4-(o-tolyldiazenyl)phenol (H2L, 1) was reacted with the salts of the following metallic cations VO2+, Fe3+, Ru3+, Co2+, Mn2+, Ni2+, Cu2+, Zn2+, UO22+ leading to the construction of complexes with the subsequent general formulas [M(HL)YXZ(H2O)].nH2O (where M = Fe3 + (3), Ru3 + (4), Co2 + (6), Mn2 + (7), Ni2 + (5), Cu2 + (8–10), Zn2 + (12) and UO22 + (13), X = Cl, CH3COO or NO3 and Y = Cl, or H2O, Z = H2O and n = 0, 2 or 6), [Cu(H2L)SO4(H2O)] and [VO(H2L)SO4(H2O)2]. The prepared compounds’ structure was elucidated analytically, theoretically, thermally and by several spectroscopical tools, such UV-Vis., NMR, and FT-IR as well as mass spectrometry. The data of different measurements and theoretical calculations were recorded in the experimental section and Tables – as well as Tables S1–S4. The postulated molecular formulae were supported by elemental analysis and mass spectrometry, which also revealed that all complexes formed in a (1L:1M) molar ratio (Figures –). All complexes have molar conductance value ranged from of 4.1–34.9 Ω−1mol−1cm2, indicative to the non-electrolyte nature of the synthetics (2–13) and the Cl−, NO3−, SO42− or CH3COO- are directly connected to the metallic cations [Citation57]. A few complexes’ noticeably high values may be caused by the partial solvolysis by DMSO molecules [Citation57].
Table 1. Data of prepared compounds’ EAS.
Table 2. ESR parameters of Cu2+complexes.
Table 3. The prepared compounds’ various quantum chemical parameters.
Table 4. Prepared compounds’ energetic properties.
3.1. Spectroscopical studies
3.1.1. FT-IR spectra
In the experimental section, the FT-IR spectral information (experimental and calculated) (Figures S1–S4) was presented and clarified as follow: the literature values of OH and NH stretching vibrations are found to be in 3400–3600 and 3150–3300 cm−1 ranges [Citation58]. In the studied Azo-Schiff base molecule (H2L, 1), there is a hydroxyl υ(O-H) and υ(N-H) bond. The stretching vibration bands of these bonds have been calculated at 3750, 3275 and 3230 cm−1. In the experimental spectrum there are two bands at 3484, 3233 cm−1 in the ligand’s FT-IR spectrum may be allocated to the hydroxyl υ(O-H) and υ(N-H) groups successively. The molecule of Azo-Schiff base (H2L, 1), includes three azomethine bonds, the literature values of C = N stretching vibrations are between 1500 and 1700 cm−1 [Citation59]. In the studied Azo-Schiff base (H2L, 1), stretching vibration bands have been calculated at 1690, 1640 and 1600 cm−1. In the experimental spectrum, vibrations observed at 1660 and 1605 cm−1 have been assigned to the C = N stretching bands of Schiff base and triazole moieties. In the experimental spectrum, the medium bands which observed at 1475, 1267 and 1000 may be ascribed to the of azo (N = N), phenolic (C–O) linkages and (N-N) linkage of a triazole moiety successively with theoretical values at 1490, 1100 and 1255 cm−1. The coordination mode of azo-Schiff base (H2L, 1) can be ascertained by comparing the complexes’ FT-IR to those of un-chelated azo-Schiff base (H2L, 1). From this comparison we can conclude that the azo-Schiff base (H2L,1) interacted with different metallic cations in one of the following two ways. Firstly, it interacted with Fe3+, Ru3+, Co2+, Mn2+, Ni2+, Cu2+(8–10), Zn2+ and UO22+ as uni-negative bidentate ligand linked covalency the metallic cations via deprotonated phenolic hydroxyl group (O22) and chelated via the azomethine nitrogen atoms (N24). The following observations served as confirmation of this chelation method; (i) The disappearing of the phenolic hydroxyl group’s stretching frequency with simultaneous positive shift of phenolic linkage (C–O) by 16–52 cm−1 [Citation60]. (ii) the negative shift in the Schiff base azomethine group’s stretching frequency (23C=N24) by 20–50 cm−1. (iii) the appearance of new stretching frequencies in the ranges of 500–600 and 456–491 cm−1 may be inductive to the ν(22O-M) and ν(24N→M) successively [Citation61]. In the second manner, the azo-Schiff base (H2L,1) interacted with VO2+ and Cu2 + (11) ions as a neutral bidentate ligand in which the coordination took place via the protonated hydroxyl (22OH) and Schiff base azomethine’s (24N) groups. The following observations served as confirmation of this chelation method; (i) the simultaneous shift of the phenolic linkage (C-OH) and the phenolic hydroxyl (OH) group's stretching frequencies [Citation60]. (ii) The negative shift in the Schiff base azomethine group’s stretching frequency (23C = N24) by 27 and 31, respectively cm−1. (iii) The emergence of new stretching frequencies at 577, 533 and 491, 461 cm−1 which could be inductive to the ν(22O→M) and ν(24N→M) successively [Citation61]. In Co2+, Mn2+, Ni2+, Cu2 + (8), Zn2+ and UO22+ complexes, the appearance of two distinctive stretching frequency bands in the of 1542–1587 cm−1 and 1339-1388 cm−1 ranges may be ascribed to υas.(COO-) and υsy.(COO-) successively, signifying that the acetate group participated in the chelation with metallic cations. Based on the difference’s magnitude between the υas(COO-) and υsy.(COO-), the coordination mode of acetate group can be determined. The difference between the νas and νs stretching frequency bands (Δ = νas-νs) which varied from 188 to 218 cm−1, indicating that the acetate anion linked with metallic cations in a monodentate manner [Citation62,Citation63]. While the two bands at 1155, 1061 and 1154, 1053 cm−1, in the sulfato complexes’ FT-IR spectra successively imputing to the mono-chelated sulphate group which was corroborated by their low values of molar conductivity [Citation2,Citation64]. The nitrato Cu2 + (10) complex displayed three bands at ν5(1408), ν1(1363) and ν2(966) implying that the nitrate anion participated in the coordination process. The difference between ν5 and ν1 stretching frequency bands (ν5-ν1) is 45 cm−1 indicating that the NO3− anion is linked with the Cu2+ cation as a uni-dentate chelator [Citation62,Citation65]. The new stretching frequency band at 963 and 970 cm−1 in the VO2+ and UO22+ complexes FT-IR’ spectra might be ascribed to the ν(O = U = O) and ν(V = O) successively [Citation61,Citation66]. According to literature, the methyl groups’ C-H vibrations appear around 2900 cm1, while the aromatic’ C-H vibrations are commonly seen at about 3000 cm1. So, the feeble bands observed in the 3095–3027 cm1 range may be ascribed to the symmetric vibrational bands of aromatic protons, while the bands observed in the 2920–2995 cm1 and from 2854 to 2875 cm1 regions may be ascribed to pure asymmetric and symmetric methyl protons’ vibrational bands [Citation67].
3.1.2. Nuclear magnetic resonance (NMR)
To get essential info around the hydrogen bonding and coordination mode of the azo-Schiff base (H2L,1), as well as to identify its structure. The 13C- and 1H-NMR spectra were obtained in DMSO-d6 and summarized in section 2.2. In 1HNMR the disappearance of aldehydic proton of the reactant at ≈ 9.9 ppm indicates to the formation of imine group between the triazole’s amine and the aldehydic moiety of the diazenyl reactant. In addition, the disappearance of aldehydic carbon at ≈190 ppm of the reactant in 13CNMR and it is replaced with up field carbon at 166.31 ensured the formation of imine moiety in the azo-Schiff base [Citation68,Citation69]. In 1HNMR (Figure S5), the compound has three exchangeable protons, two protons at 8.35, 14.37 which are assigned to the protons of NH implying to the anti- and syn-configurations of the azo-Schiff base around. Therefore, the ligand formed is a mixture of two configurations as shown in Figure . From the size of the two signals, we can conclude that the most abundant one is the anti-structure [Citation70]. The third signal appeared at 12.619 assigned to phenolic hydroxyl proton. This result was supported by the fact that their intensity decreased when the solvent was changed from DMSO to DMSO + D2O, which coincided with the downfield value of C-20 at 166.31 ppm in the benzene ring because of the existence of OH at C-20. 1HNMR also indicated the presence of one downfield proton at 9.56, which could be ascribed to the methine proton produced from the imine formation. Additionally, the CH signals overlapped in the 7.31–9.13 ppm range imputing to the two phenyl rings’ aromatic protons. The noted chemical shift of H17, H8&9 (δ = 8.819, 8.395 ppm) is a characteristic downfield value of protons in o-position for the diazinyl moiety, whereas the downfield shift of the singlet peak of H19(9.13) and the up-filed shift of H21 (7.31) suggested the presence of the hydroxyl group at ortho and meta positions to these two protons, respectively [Citation71]. The proton of the triazole ring appears at 8.173 ppm. The Zn2+ complex’s 1H-NMR spectrum revealed that the chemical shift of the phenolic hydroxy proton (33H-O22) is disappeared indicating that the hydroxyl group participated in the metallic ion’s chelation in its deprotonated form which endorsed the proposed chelation mode. The azo-Schiff base’s 13C-NMR spectrum is illustrated in Figure S6. It showed two chemical shifts 159.58 and 146.6 ppm which could be assigned to two carbons of triazole ring (26C&27C) while the chemical shift of C20 (δ = 166.31 ppm) indicates the presence of a hydroxyl group attached to this carbon. The chemical shifts of carbons C2, C4, C14, C15 (δ = 122.47, 125.51, 125.51, 128.29 ppm) are typical values for carbon attached to C13 (δ = 144.12 ppm) and C3 (δ = 150.63 ppm) connected to azo group (N = N). However, the peaks observed at 134.16, 140.47, 113.79, and 117.21 ppm were allocated to the aromatic carbons C1&5, C6, C16, and C18, respectively. The peak appearing at 19.57 ppm could be due to the methyl carbon attached to the benzene ring. The assignment of carbon and protons is confirmed by comparing with similar compounds [Citation72].
3.1.3. Azo-Schiff base’s mass spectrometry (MS)
The azo-Schiff base’s MS (Figure S7) proved the expected composition of it with molecular ion peak (M+) at 306 m/z in EI-mass. Additionally, many fragments appeared in the mass spectrometry conforming the structure. The ligand revealed several fragments at 292, 290, 276, 239, 216, 212, 188, 119, 96, 92, 77 and 69 m/z. Which could be clarified as follows. The two fragments (A&B) at m/z 292 and 290, may be because of the missing of hydroxyl or methyl group from the azo-Schiff base molecule respectively which consequently lose methyl or hydroxy group resulting to the fragment (C) formation (m/z = =276). While the 2-(iminomethyl)-4-(o-tolyldiazenyl)phenol fragment that resulted from the loss of a triazole moiety may be attributed to the d-fragment (m/z = 239) which lose C = NH moiety resulting to the fragment (E) formation (m/z = 212) that directly lose a phenolic moiety forming 1-(o-tolyl)-2λ2-diazene (F). This fragment consequently loses azo-linkage (N = N) forming toluene ending with the formation of phenyl moiety. The fragments A&B may lose a phenyl ring resulting in the formation of G-fragment (z = 216) which directly loses a N2 forming a fragment (H, m/z = 188). The fragment (H) lost a phenolic moiety forming a fragment (I, m/z = 96) which directly lost a C-NH moiety forming a triazole moiety (Figure S8). The MS of [Cu(HL)Cl(H2O)] (Figure S9) revealed a weak molecular ion peak at 422 with low intensity and another peak at 405 with low intensity corresponding exactly to [Cu(HL)Cl]. Also, there are peaks at 369 and 306 which may correspond to [Cu(HL)] and (HL). On the other hand, the MS of [Zn(HL)((O)COCH3)(H2O)3].4H2O (Figure S10) showed a peak at 483 (7%) corresponding exactly [Zn(HL)((O)COCH3)(H2O)3] with no peaks higher than this one. This species showed peaks at 460(2%), 448(3) and 430(3) which formed after the successive removal of coordinated water molecules. The medium peak observed at 370 (15%) may correspond to [Zn(HL)] while the peak at 306 may be due to ligand molecule after the elimination of Zn atom.
3.1.4. Magnetic moment (µeff) and electronic absorption spectroscopic (EAS) measurements
The azo-Schiff base’s EAS and that of metallic complexes (1–13) were determined in DMSO and itemized in Table and shown in Figures S11–S14. Ligand’s EAS revealed two sets of bands in the UV and visible regions. The bands that appeared at the shortest wavelengths (283 and 260 nm) could be attributed to the π→π* and π→π* transitions, which were attributed to intra-ligand transitions and transitions in the phenyl moieties [Citation73]. While the two bands at 313 and 333 could be inductive to the n→π* transition of Schiff base and triazole azomethine groups [Citation73]. The complexes’ n-π* transitions were shifted somewhat compared to the free ligand, which is most likely due to the chelation of azomethine-nitrogen with the metallic ions [Citation74]. In hexa-bonded VO2+ complex the ground state 2T2g and 2Eg splits into 2B2g, 2Eg and 2B1g, 2A1g, respectively thus in octahedral field there are three spin allowed transitions corresponding to 2B2g(dxy)→2Eg(dxz,dyz)(ν1), 2B2g(dxy)→2B1g(dx2-y2)(ν2) and 2B2g(dxy)→2A1g(dz2)(ν3) successively [Citation75]. So the peaks that appeared at 1048, 809, and 470 nm in the EAS of the VO2+ complex (2) are imputable to the 2B2g(dxy)→2Eg(dxz,dyz)(ν1), 2B2g(dxy)→2B1g(dx2-y2)(ν2), and 2B2g(dxy)→2A1g(dz2)(ν3) transitions successively [Citation76]. These transitions are consistent with a distorted octahedral structure for the VO2+ cation (Figure ) [Citation77,Citation78]. This VO2+-complex has an eff value of 1.67 BM, which is consistent with the spin-only value [Citation77,Citation78]. In the hexa-coordinated Fe3+ complex, the ground state (6S) splits into 6A1g, 4T1g (P), 4T2g, 4A1g, and 4E1g. Thus, in the octahedral field, there are three spin-allowing bands relating to the following 6A1g(S)→4T1g(G) (∼950 nm), 6A1g(S)→4T2g(G) (∼600 nm), and 6A1g(S)→4A1g(G) 4E1g(G) (∼450 nm) transitions [Citation79]. According to this explanation, the EAS for the Fe3+ complex (3) demonstrated three peaks at 898, 585, and 445 nm, referring to the above transitions, which are well-suited to the distorted octahedral geometry (Figure ) [Citation75]. The µeff value of Fe3+ complex (3) at room temperature was found to be 5.88 BM inductive to a five-unpaired electron system, which is well-suited to high-spin octahedral Fe3+ cations [Citation76]. The Ru3+ complex exhibits a magnetic moment value of 1.66 BM, which is compatible with a spin-only value for low-spin Ru3+ species [Citation65]. The low-spin Ru3+ complex has a 5t2g arrangement, with the first excited doublet levels being 2T1g and 2A2g in the order of decreasing energy that arise from the 4t2g→1eg configuration. As a result, two peaks that correspond to 2T2g→2T1g and 2T2g→2A2g are feasible. The Ru3+ complex’s EAS (4) nevertheless demonstrated one band at 533 that may be ascribed to the 2T2g→2A2g transition. While the band at 425 nm may be the result of a charge transfer transition (LMCT) from the filled chelator (HOMO) orbital to the single-busied ruthenium t2 orbital (LUMO),. The band position is similar to that of other octahedrally structured Ru3+ complexes [Citation77,Citation80]. In octahedral symmetry for Co2+ complexes, ground state (4F) splits into 4A2g, 4T2g and 4T1g (P). Thus, in the octahedral field, three spin-allowing transitions correspond to the (v1) 4T1g (F)→4T2g (F), (v2) 4T1g (F)→4A2g, and (v3) 4T1g (F)→4T1g (p) [Citation77,Citation81]. According to this description, the EAS for Co2+ complex (5) showed two peaks at 568 and 1090 nm imputable to the (v2)4T1g(F)→4A2g and (v1)4T1g(F)→4T2g(F) transitions successively, which is well-suited with an octahedrally structured structure (Figure ) [Citation77,Citation81]. The distortion of this structure is suggested based on its small value of ν1/ν2 (1.92) in comparison to the typical range for octahedrally Co2+ complexes (1.95–2.48). This is coherent with the broadness of the bands, which is attributed to the envelope of the electronic transitions from 4Eg (4T1g) to 4Eg and 4B2g components of 4T2g in a tetragonally distorted octahedral setting [Citation82]. The Co2+ complex (5) resembles a high-spin Co2+ ion (d7) where its µeff is equal to 4.46 BM, which is equivalent to three unpaired electrons [Citation83]. The Mn2+ complex’s EAS showcased feeble bands at 545 and 491 nm, which are attributed to 6A1g→4T1g(4G) (υ1) and 6A1g→4T2g(υ2) transitions successively, corresponding to a tetrahedral geometry around the Mn2+ ion (Figure ) [Citation84]. The third and fourth bands 6A1g→4Eg(G), 4A1g(G) (v3), and 6A1g→4T2g(D)(v4) do not appear here because of the broadening of the bands [Citation77,Citation85]. This Mn2+ complex has a µeff value of 5.78 BM, which is consistent with a system having five unpaired electrons and is in close proximity to a high-spin Mn2+ ion (d5)[Citation86]. In the tetrahedral symmetry of the Ni2+ complex, the 3T2g state term originating from the 3F level becomes the ground state. Thus, in the tetrahedral field, there are three spin allowable bands comparable to the next (v3)3T1g(F)→3T1g(P) (625), (ν2)3T1g(F)→3A2g(P) (1000), and (ν1)3T1g(F)→3T2g(F) (2000nm) transitions. The Ni2+ complex’s EAS exhibited two peaks at 940, 663 nm, imputable to the 3T1g(F)→3A2g(P)(ν2) and 3T1g(F)→3T1g(P)(v3) transitions successively, which is well-suited with a tetrahedral geometry (Figure ) [Citation77,Citation87]. The 3rd band, which is attributed to the (ν1)3T1g(F)→3T2g(F) (2000nm), is not noticeable because it is out of spectrophotometer scale. The Ni2+ complex (7) was found to have an µeff value of 3.08 BM at room temperature, which is within the typical range for high-spin tetrahedral Ni2+ complexes and rules out the idea of a square planar structure [Citation88]. The EAS of Cu2+ complexes (8–11) showed three bands in the ranges 1083–1093 and 622–670, 428–485 nm. These bands are ascribable to the following transitions: (υ1)2B1g→2B2g (dx2-y2→dyzdxz), (υ2)2B1g→2A1g (dx2-y2→dz2), and (υ3)2B1g→2Eg (dx2-y2→dxy), which are typical for square planar Cu2+ complexes (Figures –) [Citation77]. The Cu2+ complexes’ magnetic moment values range from 1.88 to 2.20 BM, which are consistent with the existence of one unpaired electron of Cu2+ ions in a square planar structure. Two bands were visible on the EAS of the UO22+ complex at 486 and 423 nm. Charge transfer from the UO22+ moiety's oxygen to the U6+ ion's f-orbital (O→U) is believed to be the cause of the lower energy band (486 nm). This band boarding suggests that the energy of the two O-U charge transfer transitions for oxo cations are different [Citation89]. However, the higher energy band is thought to be responsible for the charge transfer from the ligand to the UO22+ ion (LMCT)[Citation90].
3.1.5. Cu2+ complexes ESR spectrum
The data of Cu2+-complexes ESR spectra was tabulated in Table . It showed anisotropic signals with g|| values ranged from 2.214 to 2.26, g⊥ values ranged from 2.042 to 2.060 and giso values ranged from 2.099 to 2.124 confirming to a d9 configuration denoting to axially symmetric anisotropy type of a d(x2-y2) ground state [Citation91,Citation92]. The g-values for Cu2+-complexes (7–8) and (10–11) supported that they have one of the following geometries an octahedral or square planar while the trend g||>g┴>2.0023 suggesting that these geometries could be deformed [Citation93]. The g-value can be correlated to the parameter term of exchange interaction G by equation, G = (g||-2)/(g┴-2) [Citation92]. According to Hathaway if G is less than 4.0, a considerable exchange coupling is present but if G is more than 4.0, exchange coupling is negligible. The Cu2+ complexes G-values equal to 4.19, 5.30, 4.41 and 5.62, respectively implying that the exchange interaction between Cu2+ ions is negligible [Citation94]. Also, the g||/A|| values can be serve as a diagnostic of stereochemistry [Citation95]. According to literature, for square-planar complexes the g||/A|| values ranged from 105 to135 cm−1 but for tetragonally deformed complexes ranged from 150 to 250 cm−1. The g||/A|| value for the Cu2+ complexes equal to 12.97, 118.97, 133.84 and 112.71, respectively. These data confirmed that the Cu2+ complexes have a square planar. If the g|| < 2.3 the environment is mainly covalent while if g|| > 2.3 the environment is essentially ionic [Citation96]. The g||-values for the Cu2+ complexes under study were ranged from to 2.214 to 2.26, implying to the covalency nature of bonds in these complexes [Citation96]. The relationship between the g-values and the orbital reduction factor (k) can be established through the following equations [Citation97,Citation98].
where ΔExz and ΔExy are the electronic transitions 2B1g→ 2B2g and 2B1g→ 2Eg, sequentially, λo = −828 cm−1 is the spin orbit coupling of free Cu2+ ion. If k = 1 the environment is ionic, but if k < 1 environment is covalent. The calculating values of k is less than unity (0.72–0.86) suggesting to the covalency nature of these complexes which is in agreeable with the results attained from the g|| values [Citation98]. The value of in-plane σ-bonding parameters αCu2 can be appreciated according to Kivelson and Neiman [Citation96].
where P is the free ion dipolar term equals 0.036,
is the parallel coupling constant expressed in cm−1. If the value of αCu2 = 1, the bond could be pure ionic but if αCu2 = 0.5, the bond could be pure covalent [Citation91,Citation93]. The values of αCu2 for Cu2+-complexes were found to be range from 0.665 to 0.787 suggesting to covalency nature of in-plane σ-bonding [Citation99]. The out-of plane and in-plane π-bonding coefficients γ2 and β2 can be estimated by published equations [Citation97,Citation100].
The Cu2+ complexes γ2 and β2 values were found to be ranged from 0.27 to 0.70 and from 0.63 to 0.75 successively, demonstrating that the in-plane and out-of-plane π-bonding have a covalency nature [Citation63,Citation97].
3.1.6. Thermogravimetric analysis
By measuring the weight loss as a function of temperature, the TGA analysis can be used to not only determine the thermal stability of complexes under investigation but also to give essential information about the water molecules’ nature in the complexes’ skeleton. As record in Table S1 and shown in Figures S15–S17, the isolated complexes’ thermograms recorded in temperature ranged from room temperature to 800°C. The complexes’ thermogram showed noteworthy continuous phases of decomposition in the temperature range of 41–632°C, which culminated in the creation of residues made of metal oxide. The subsequent steps of decomposition may be explained as follows. In the thermograms of Fe3+, and Zn2+, four mass loss steps were seen. The first stage of the degradation took place between 34 and 133°C, with weight losses of 6.71, and 1255% (calcd at 7.15, and 12.96%) related to the liberation of two, or four hydrated water molecules, respectively. The second decaying stage occurred in the range of temperature of 142–248°C with loss of two or three chelated water molecules, with a mass loss of 6.49, or 10.01% (calcd 7.15, 9.72%) successively. The third stage of decaying occurred in the 234–354°C temperature range which was accompanied by loss of two chloride or one acetate anion, which resulted in mass losses of 13.87, and 10.34% (calcd. 14.07, 10.69%). The fourth phase of decaying took place between 311 and 632°C, with mass losses of 52.91, and 50.89% (calcd at 53.42, 51.98), corresponding to the complete complexes’ breakdown with the formation of Fe2O3, and ZnO, respectively. The VO2+, Ru3+, Co2+, Mn2+, Ni2+, and Cu2+ complexes’ thermograms revealed three weight lose steps. In the temperature range 95–310°C, the first breakdown step involves the loss of one, two, or three chelated water molecules associated with weight falling of 4.05, 6.88, 11.00, 4.41, 3.81, 3.55, 4.55, 3.88 and 3.33% (calcd. 3.70, 7.02, 11.32, 4.12, 4.08, 4.04, 4.27, 4.01 and 3.72%). The second breakdown step occurred in temperature range 194–380°C associated with falling in weight equal to 19.41, 13.46, 11.87, 13.76, 13.41, 13.76, 8.41, 13.41 and 19.76% (calcd. 19.70,13.81, 12.45, 13.59, 13.48, 13.33, 8.40, 13.81 and 19.84%) referring to the losing of sulfate, chloride, acetate, or nitrate anions. The third decomposing stage happened in the temperature range 280–543°C with losing in weight equal to 58.96, 55.43, 60.89, 66.56, 63.77, 66.01, 67.76, 64.76 and 58.20% (calcd. 57.95, 54.80, 60.53, 66.07, 65.51, 64.79, 68.50, 64.56 and 60.00%) leading to the complete complexes’ breakdown leaving the corresponding metal oxide (V2O5, Ru2O3, CoO, MnO, NiO, and CuO) [Citation101–104]. While in the TG-graph of UO22+, three mass loss steps were seen. The first stage of the degradation took place between 50 and 160°C, with weight losses of 13.81% (calcd at 13.57%) related to the liberation of six hydrated water molecules. The second decaying stage occurred in the range of temperature of 160–255°C with loss of chelated water molecules two and one acetate ion, with a mass loss of 14.50% (calcd 14.25%). The third stage of decaying occurred between 260 and 528°C, with mass losses of 35.76% (calcd 36.28%), corresponding to the complete complex’s breakdown with the formation of UO3.
3.2. Density functional theory elucidation
3.2.1. Geometrical optimization
Figures and S18–S20 depict the completely optimized structure for the azo-Schiff (H2L, 1) and its chelated complexes. The complexes’ chosen bond length and bond angle values showed distorted octahedral, square planar or tetrahedral geometry around the metallic cations (Tables S2–S3). In complexes the deprotonated/protonated phenolic oxygen and azomethine nitrogen atoms of azo-Schiff base (H2L) bonded the metallic cations. They displayed a slight shrinkage at bond lengths of the azomethine linkage (23C=N24) and the protonated phenolic hydroxy linkage (20C−22OH) as in VO2+ and Cu2+ (11) complexes. But they displayed an elongation at bond lengths of deprotonated phenolic hydroxy linkage (20C-O22). The bond length between the metallic cation and deprotonated phenolic oxygen atom was found to be in the 1.80–1.91 Å range. While the bond length between the protonated phenolic oxygen or azomethine nitrogen atoms and metallic cations were found to be in the 2.68496–2.85694 Å range. In hexa-coordinated VO2+, Fe3+, Ru3+, Co2+ and Zn2+ complexes, the two axial sites were busied by two chelated water molecules while in Fe3+, Ru3+, Co2+ and Zn2+ complexes but in VO2+ complex the axial positions occupied by one chelated water molecule and vanadyl oxygen. While the four equatorial locations were inhabited by phenolic oxygen, azomethine nitrogen, two chloride anions in Fe3+, Ru3+, but in complexes VO2+, Co2+ and Zn2+ complexes, the four equatorial locations were busied by phenolic oxygen, azomethine nitrogen and chelated molecule molecules. In tetrahedral Ni2+ and Mn2+ the four locations of tetrahedron were inhabited by azomethine nitrogen, phenolic oxygen, acetate anion and chelated water oxygen. In square planar Cu2+ complexes the four corners of square were occupied by azomethine nitrogen, phenolic oxygen, anion (Acetate, Chloride, nitrate, or sulphate) and chelated water oxygen. The angles of bond in the coordination sphere of complexes were indicative to distortion of the structure. The geometric index for four-coordinate complexes, τ4 was calculated by τ4 = 360° − (α+ β)/141°. Where α and β are the two greatest valance angles of coordination center. According to literature, for square-planar geometry the τ4 values equal 0 but for tetrahedral geometry the τ4 values equal 1. While intermediate geometry, involving seesaw and trigonal pyramidal, will be ranged from 0 to 1. The τ4 values for the four-coordinate Ni2+ Mn2+ and Cu2+ complexes equal to 0.91, 0.93, 0.44, 0.39, 0.37 and 0.51, respectively. These data confirmed that the Ni2+ and Mn2+ complexes have predominately distorted tetrahedral geometry while Cu2+ complexes are predominately distorted square planar geometry [Citation105,Citation106].
3.2.2. Molecular parameters
Basing on Koopman theorem, The azo-Schiff base’s quantum chemical parameters and that of its metal chelates (2–12) were calculated and their data were itemized in Tables – [Citation107]. The azo-Schiff base’s molecular orbital and that of its chelated complexes (LUMO and HOMO) were demonstrated by DFT/B3LYP/6-311G+(d,p) calculation and it is shown in Figure . The negatively and positively charged areas were shown using red and green colors successively. Figure demonstrated that the HOMO molecular orbital was spread nearly over the azo moiety including one phenyl ring, some carbons atoms of the second phenyl ring and phenolic oxygen whereas the LUMO molecular orbital was spread over the triazole moiety, azo linkage, one phenyl ring and some carbons of the second phenyl ring as well as phenolic oxygen and azomethine nitrogen. The energies of the HOMO and LUMO as well as the nearby molecular orbitals are negative, demonstrating the stability of the azo-Schiff base molecule [Citation108]. The azo-Schiff base’s chelation with metallic cations happened via the phenolic oxygen atom (O22), and azomethine nitrogen atom (N24) which are the effective positions for chelation because they have high electronegative charge. From Figure shows that the HOMO level is naturally positioned on the toluene moiety and azo-linkage’s nitrogen atoms (N11&12) in addition to atoms of phenyl ring indicating that the O22 is the preferred position for nucleophilic attacking with the cations inductive that this atom has large coefficients of HOMO density and they are directed to the metallic centers [Citation109]. The compounds separation energy values (ΔE = ELUMO−EHOMO) verified that the complexes (2–12) have ΔE ranged from 0.0593 to 0.1003 which are less stable and more reactive than the azo-Schiff (H2L, 1) with ΔE = 0.1017 [Citation110]. The term “electron affinity” refers to the energy expended if the system accepts an additional electron (EA = -ELUMO) while the term “ionization potential” refers to the amount of energy needed to expel one electron from a species (IP = -EHOMO) [Citation109,Citation111]. The absolute softness (σ = 1/η) and absolute hardness (η = (IP-EA)/2) could be utilized as a scale to reflect an atom's capacity to accept electrons and resist charge transfer, respectively [Citation112]. The stability and reactivity of molecules might be predicted using these two factors [Citation112]. Hard molecules have a large energy gap compared to soft molecules’ modest. As a result, the soft molecules’ reactivity is higher than that of hard molecules because soft molecules may easily transfer electrons to a receiver. The ligand molecules are the most efficient for coordination because, in coordinative compounds, the metal cations act as Lewis’ acids whereas the ligand molecules function as Lewis’ bases. The HOMO–LUMO diagram, which shows how reactive a molecule is, also shows a molecule's ability to transmit a charge and an electron to an accepting species. The molecule’s bioactivity is due to this property, which makes it easy for electrons to transfer from the HOMO to LUMO. The HOMO electron density is more evenly distributed in the backbone of complexes, as seen in Figure , which also denotes increased reactivity [Citation113]. Consequently, the metallic cations are effectively chelated by the chelator with an appropriate σ value. This conclusion is assured from the chelator’s chemical potential value (Pi = -χ) [Citation108,Citation109]. From values of absolute electronegativities (χ = (IP + EA)/2), index of reactivity (ΔNmax = −Pi/η), chemical potentials (Pi = -χ), index of electrophilicity (ω = Pi 2/(2η)) [Citation114], we can conclude that (i) the Nmax is the measuring of the system's energy stabilization if it gains an extra electronic charge from its surroundings (ii) The chemical potential (Pi) recognizes the quantity of charge and the direction in which it is transferred, where the electrophiles are the chemical that may take electrons from their surrounding and must reduce their energy when doing. So, its Pi values must be negative as reinforced by the data in Table . (iii) The ω determines the ability of chemical species to receive electrons. Therefore, the high ω value indicates a well- functioning electrophile while low value implies a well-functioning nucleophile. (iv) The metallic cations were linked by azo-Schiff base (H2L, 1) by oxygen atom of hydroxy group (O22) and nitrogen atom of azomethine group (N24) which own high electronegative charge demonstrating that these nitrogen and oxygen atoms are the effective sites for attachment. (v) The complexes dipole moment values are ranged from 3.68 to 15.4 Debye which are greater than that of azo-Schiff base, (H2L, 1) (3.29 Debye), confirming that the attachment occurs by oxygen atom of hydroxy group (O22) and nitrogen atom of azomethine group (N24). Other energetic properties of the synthesized compounds which computed by DFT method are itemized in Table . The data of Table showed that the calculated complexes’ binding energy are more than that of azo-Schiff base. This suggests that the stability of complexes is more than that of azo-Schiff base [Citation115].
3.2.3. Milliken atomic charges for the ligand
Milliken population analysis was used to determine the Milliken atomic charges of H2L atoms by applying the DFT/B3LYP levels with basis set (6-311 + G(d,p)) in gas phase, which is shown in Figure S21 and listed in Table S4. The atomic charge playing a key role in the estimation of molecular system’s quantum chemical parameters as the atomic charge effect on the vibrational spectra, dipole moment, polarizability, electronic structure, and other properties of molecular system [Citation58]. The Milliken analysis data discovered that the hydrogen atoms have positive charge (1.567 × 10−1–3.742 × 10−1 a.u), because of their losing electronic charge to the neighboring carbon atoms, whereas the hydrogen atoms attached to electronegative atoms (H-32 & H-33) have the highest positive charge of all hydrogen atoms because of their attachment to high electronegatively atoms (N,O) [Citation58]. All oxygen and nitrogen atoms possess a negative Milliken atomic charge demonstrating that the oxygen and nitrogen atoms acted as electron receptors and server as donating atom for chelation.
3.2.4. Molecular electrostatic potential (MEP)
The charge distributions of azo-Schiff base and its variable charged zones are described and shown by MEP, which is estimated based on DFT calculation (Figure ). MEP is typically employed as a reactivity diagram and to discover hydrogen bonding interactions, as well as nucleophilic and electrophilic reactions, in organic compounds [Citation58,Citation116]. The energy interaction between the molecule's nucleus protons, and electrons is represented by the MEP, V(r) at r(x, y, z) coordinates. The value of V(r) for the system under study was calculated using the following equation [Citation58,Citation116].
where, r’, ZA, and ρ(r′) indicates to the dummy integrated, and variables nucleus’ (A) charge that placed at RA, electron charge density of molecule respectively [Citation117]. The MEP employs a variety of colors to depict the various electrostatic potential values on the surface of molecule (from blue to red). Electron-rich locations are symbolized by a red color, whereas electro-positive locations are symbolized by the blue color, whereas electrostatic potentials close to zero are symbolized by the green color. The dihydrazone-oxime’s MEP 3D picture was displayed in Figure . As displayed in Figure , the greatest negative potential sites were located on the electronegatively atoms (O&N) which are the preferred locations for metallic chelation. The strongest positively potential regions (blue) were focused on a few carbon and hydrogen atoms, (+0.035 × 10−1 to 3.742 × 10−1 a.u). This result implies that nitrogen and oxygen atoms are more repellent than hydrogen atoms, which have more attractive characteristics.
3.3. In vitro anti-microbial screening
The antimicrobial assignment results of the ligand (H2L,1), and its metal chelates (2–13) versus B. subtilis, E. coli (bacteria) and A. niger (fungus) is shown in Table and shown in Figure . The complexes exhibited antifungal effects with inhibition zones ranging from 8 to 20 mm with indexes of activity from 47% to 118%. Both Zn2+ and UO22+ complexes exhibited the high potency antifungal activity with zones of inhibition equal to 20 and 17 mm with activity indexes 118% and 100% (of Am B), respectively. Ru3+ complex showed the least antifungal activity among all metal complexes investigated. The complexes exhibited bactericidal activity versus E. coli and B. subtilis with zones of inhibition in the 12–33- and 10–28-mm ranges with activity indexes in the 33%–92% and 26%–72%, respectively. The most potent complexes against E. coli are VO2+ and Ru3+ complex with inhibition zone equal to 33 mm with activity index 92% while versus B. subtilis the UO22+ is the most potent complex. The least potent complexes are Ni2+ and Mn2+ complexes. The complexes’ antimicrobial action is higher than that of the azo-Schiff base. This can be justified by Tweedy's chelation theory [Citation118] and/or the concept of cell permeability [Citation119], which can both be used to explain how complexes function. According to this theory, the polarity of metallic ions is diminished because of the sharing of their charge with the chelators’ donor atoms and the probability of π-electron delocalization over the chelate rings. This polarity diminishing leads to an increase in the lipophilic nature and this assists the penetration of complex molecules through the lipid membrane of the microbe cells. The overlap between the orbitals of the metal ions and the ligand’s molecular orbitals may be the cause of some complexes’ significant antibacterial activity. This overlapping may also help further distribute the positive charge of metal ions among the ligand molecule's donor groups. the resonance of π-electrons and the lipo-solubility of the whole chelate are increased and as a result, the metallic ions’ polarity is reduced. Therefore, it facilitates the diffusion of the complexes into microorganisms. The low activity of azo-Schiff base (H2L, 1) and some of its metallic complexes against some microscopic strains might be attributed to the high polarity and hydrophobic nature of this compounds which decrease in the interaction with lipid membrane of the microorganism. Furthermore, these microbial strains’ efflux pumps prevent these compounds from penetrating the lipid membrane, providing protection against these complexes [Citation120]. While chelation does indeed augment the biological activity of these complexes, several other factors come into play. These factors include the dipole moment, the geometry of the complexes, their concentration, molecular size, bond length, coordination sites, and hydrophobicity, all of which collectively influence their antimicrobial activity. Therefore, it is evident that the enhancement of antimicrobial activity is not solely attributed to chelation but rather results from a combination of various contributing factors [Citation121,Citation122]. The most exciting point of microbicides assignments is that this azo-Schiff base is inactive while other types of Schiff base such as ethyl-6-ethyl-2-((2- hydroxybenzylidene)amino)-4,5,6,7-tetrahydrothieno[2,3-c]pyridine-3-carboxylate [Citation2], N-(4-fluorobenzylidene)-2-(quinolin-2-yloxy)acetohydrazide [Citation123], 3-(-(1,5-dimethyl-3-oxo-2-phenyl-2,3-dihydro-1H-pyrazol-4-yl)methylene)hydrazonoindolin-2-one [Citation124,Citation125], 3-((hydroxyimino)butan-2-ylidene)benzohydrazide [Citation126], Also the azo-compounds were more active than Azo-Schiff base [Citation8,Citation33,Citation118,Citation127], Also, their metal complexes show enhanced biological activity over azoSchiff base complexes. The lower activity of azoSchiff base and its complexes against both bacteria and fungi might be attributed to the presence of the azo group.
Figure 8. The microbicidal activity’s order of azo-Schiff base and its complexes (1 mg/mL/DMSO, at 28/37°C, pH 7.4).

Table 5. The prepared compounds’ microbicidal activity (mg/mL/DMSO, at 28/37°C, pH 7.4).
4. Conclusion
Condensation of 2-hydroxy-5-(o-tolyldiazenyl)benzaldehyde with 1H-1,2,4-triazol-5-amine yielded a potentially bi-dentated Azo-Schiff base namely, 2-(((1H-1,2,4-triazol-5-yl)imino)methyl)-5-((4-nitrophenyl)diazenyl)phenol (H2L, 1) which formed Ru3+, Fe3+, VO2+, Ni2+, Co2+, Mn2+, Cu2, Zn2+ and UO22+ complexes of the types [M(HL)XYZ(H2O)].nH2O, [Cu(H2L)SO4(H2O)] and [VO(H2L)SO4(H2O)2] in presence of counter ion (acetate, chloride, nitrate or sulphate). The structure of these prepared complexes was elucidated by physico-chemical, magnetic susceptibility, molar conductance, thermal studies, electronic, mass, nuclear magnetic resonance, and infrared spectra as well as theoretical studies. The results demonstrated that the metal-chelator stoichiometry in all complexes is 1M:1L in which the azo-Schiff base chelator worked as a monobasic/neutral NO-bidentate chelator bonding the metallic center via oxygen atom of deprotonated/protonated hydroxyl group and azomethine nitrogen atom adopting distorted octahedral, tetrahedral, or square planar geometry around the metallic center. Thermal gravimetric analysis (TGA) was used to examine thermal behavior, which focuses on the complexes’ ranges of stability and breakdown. Calculations based on DFT were employed to hypothetically analyze both the free azo-Schiff base’s 3D optimized structure and the metal complexes’ structures. The HOMO and LUMO energies were utilized for estimation the quantum chemical characteristics such as the energy gap, absolute electronegativity, chemical potential chemical hardness and chemical softness. The data of theoretical calculation displayed that all synthetics have negative HOMO and LUMO energies inductive to their stabilities. The in vitro antimicrobial tests reveal that the complexes have a high level of effectiveness as antifungal and antibacterial reagents more than the un-complexed azo-Schiff base ligand. Among the newly prepared complexes the Zn2+ and UO22+ complexes were the most effective complexes against fungi. They showed activity more than or resemble AmB. While the VO2+, Ru3+ and Zn2+ complexes were the most effective complexes versus bacteria with activity close to Tc. This finding suggests that a unique model of molecules with such high potency has a lot of promising. More investigation is necessary to find novel, potent congeners to address the microbial resistance problems with antimicrobial medicines. In our forthcoming research endeavors, our primary emphasis will be on the synthesis of innovative hybrid metal complexes incorporating azo-azomethine which is able to enhance the stability, decrease the toxicity, and enhance the biological activity, Furthermore, searching for novel synthetics is a valued target to overawe the microbial resistance problems of antimicrobial agents.
Supplemental Material
Download MS Word (6.7 MB)Disclosure statement
No potential conflict of interest was reported by the author(s).
Additional information
Funding
References
- Abou-Dobara MI, Diab MA, El-Sonbati AZ, et al. Correlation between the structure and biological activity studies of supramolecular coordination azodye compounds. Arab J Chem. 2017;10:S1316–S1327. doi:10.1016/j.arabjc.2013.03.018
- Shakdofa MME, Labib AA, Abdel-Hafez NA, et al. Synthesis and characterization of VO2+, Co2+, Ni2+, Cu2+ and Zn2+ complexes of a schiff base ligand derived from ethyl 2-amino-6-ethyl-4,5,6,7-tetrahydrothieno[2,3-c]pyridine-3-carboxylate and their investigation as fungicide agents. Appl Organomet Chem. 2018;32(12):e4581. doi:10.1002/aoc.4581
- Sarigul M, Sari A, Kose M, et al. New bio-active azo-azomethine based Cu(II) complexes. Inorg Chim Acta. 2016;444:166–175. doi:10.1016/j.ica.2016.01.042
- Pervaiz M, Sadiq S, Sadiq A, et al. Azo-Schiff base derivatives of transition metal complexes as antimicrobial agents. Coord Chem Rev. 2021;447:214128. doi:10.1016/j.ccr.2021.214128
- Pervaiz M, Riaz A, Munir A, et al. Synthesis and characterization of sulfonamide metal complexes as antimicrobial agents. J Mol Struct. 2020;1202:127284. doi:10.1016/j.molstruc.2019.127284
- Alghuwainem YAA, Abd El-Lateef HM, Khalaf MM, et al. Synthesis, structural, DFT, antibacterial, antifungal, anti-inflammatory, and molecular docking analysis of new VO(II), Fe(III), Mn(II), Zn(II), and Ag(I) complexes based on 4-((2-hydroxy-1-naphthyl)azo) benzenesulfonamide. J Mol Liq. 2023;369:120936. doi:10.1016/j.molliq.2022.120936
- Alghuwainem YAA, El-Lateef HMA, Khalaf MM, et al. Synthesis, DFT, biological and molecular docking analysis of novel manganese(II), iron(III), cobalt(II), nickel(II), and copper(II) chelate complexes ligated by 1-(4-nitrophenylazo)-2-naphthol. Int J Mol Sci. 2022;23(24):15614.
- Abdou A, Mostafa HM, Abdel-Mawgoud A-MM. Seven metal-based bi-dentate NO azocoumarine complexes: synthesis, physicochemical properties, DFT calculations, drug-likeness, in vitro antimicrobial screening and molecular docking analysis. Inorg Chim Acta. 2022;539:121043. doi:10.1016/j.ica.2022.121043
- Abd El-Lateef HM, Khalaf MM, Kandeel M, et al. New mixed-ligand thioether-quinoline complexes of nickel(II), cobalt(II), and copper(II): synthesis, structural elucidation, density functional theory, antimicrobial activity, and molecular docking exploration. Appl Organomet Chem. 2023;37(7):e7134.
- Mohammadi A, Khalili B, Tahavor M. Novel push-pull heterocyclic azo disperse dyes containing piperazine moiety: synthesis, spectral properties, antioxidant activity and dyeing performance on polyester fibers. Spectrochim Acta – Part A. 2015;150:799–805. doi:10.1016/j.saa.2015.06.024
- Deb T, Choudhury D, Guin PS, et al. A complex of Co(II) with 2-hydroxyphenyl-azo-2′-naphthol (HPAN) is far less cytotoxic than the parent compound on A549-lung carcinoma and peripheral blood mononuclear cells: reasons for reduction in cytotoxicity. Chem-Biol Interact. 2011;189(3):206–214. doi:10.1016/j.cbi.2010.11.007
- Rani P, Srivastava VK, Kumar A. Synthesis and antiinflammatory activity of heterocyclic indole derivatives. Eur J Med Chem. 2004;39(5):449–452. doi:10.1016/j.ejmech.2003.11.002
- Tonelli M, Vazzana I, Tasso B, et al. Antiviral and cytotoxic activities of aminoarylazo compounds and aryltriazene derivatives. Bioorg Med Chem. 2009;17(13):4425–4440. doi:10.1016/j.bmc.2009.05.020
- Zafar W, Ashfaq M, Sumrra SH. A review on the antimicrobial assessment of triazole-azomethine functionalized frameworks incorporating transition metals. J Mol Struct. 2023;1288:135744. doi:10.1016/j.molstruc.2023.135744
- Pandey A, Rajavel R, Chandraker S, et al. Synthesis of schiff bases of 2-amino-5-aryl-1,3,4-thiadiazole and its analgesic, anti-inflammatory and anti-bacterial activity. E-J Chem. 2012;9(2):2524–2531. doi:10.1155/2012/145028
- Middya P, Saha A, Chattopadhyay S. A comprehensive overview on the synthesis, structures and applications of mono-nuclear transition metal complexes with asymmetrically substituted ‘salen-type’ Schiff bases. Inorg Chim Acta. 2023;545:121246. doi:10.1016/j.ica.2022.121246
- Yadav M, Yadav D, Pal Singh D, et al. Pharmaceutical properties of macrocyclic schiff base transition metal complexes: urgent need in today’s world. Inorg Chim Acta. 2023;546:121300. doi:10.1016/j.ica.2022.121300
- Al-Shemary RK, Mohapatra RK, Kumar M, et al. Synthesis, structural investigations, XRD, DFT, anticancer and molecular docking study of a series of thiazole based Schiff base metal complexes. J Mol Struct. 2023;1275:134676. doi:10.1016/j.molstruc.2022.134676
- Talebi A, Salehi M, Khaleghian A, et al. Evaluation of anticancer activities, apoptosis, molecular docking, and antioxidant studies of new Ni(II), VO(IV), Cu(II) and Co(III) Schiff base complexes. Inorg Chim Acta. 2023;546:121296. doi:10.1016/j.ica.2022.121296
- Yin L, Zhang S, Zhou T, et al. Synthesis, characterization, and anticancer activity of mononuclear Schiff-base metal complexes. J Mol Struct. 2023;1275:134683. doi:10.1016/j.molstruc.2022.134683
- Deswal Y, Asija S, Kumar D, et al. Transition metal complexes of triazole-based bioactive ligands: synthesis, spectral characterization, antimicrobial, anticancer and molecular docking studies. Res Chem Intermed. 2022;48(2):703–729. doi:10.1007/s11164-021-04621-5
- Agarwal P, Asija S, Deswal Y, et al. Recent advancements in the anticancer potentials of first row transition metal complexes. J Indian Chem Soc. 2022;99(8):100556. doi:10.1016/j.jics.2022.100556
- Krishna GA, Dhanya TM, Shanty AA, et al. Transition metal complexes of imidazole derived Schiff bases: antioxidant/anti-inflammatory/antimicrobial/enzyme inhibition and cytotoxicity properties. J Mol Struct. 2023;1274:134384. doi:10.1016/j.molstruc.2022.134384
- Sridhar SK, Pandeya SN, Stables JP, et al. Anticonvulsant activity of hydrazones, Schiff and Mannich bases of isatin derivatives. Eur J Pharm Sci. 2002;16(3):129–132. doi:10.1016/S0928-0987(02)00077-5
- El-Gammal OA, Mohamed FS, Rezk GN, et al. Structural characterization and biological activity of a new metal complexes based of Schiff base. J Mol Liquids. 2021;330:115522. doi:10.1016/j.molliq.2021.115522
- El-Bindary MA, El-Desouky MG, El-Bindary AA. Metal–organic frameworks encapsulated with an anticancer compound as drug delivery system: synthesis, characterization, antioxidant, anticancer, antibacterial, and molecular docking investigation. Appl Organomet Chem. 2022;36(5):e6660. doi:10.1002/aoc.6660
- El-Bindary MA, El-Bindary AA. Synthesis, characterization, DNA binding, and biological action of dimedone arylhydrazone chelates. Appl Organomet Chem. 2022;36(4):e6576. doi:10.1002/aoc.6576
- Abdel-Rhman MH, Motawea R, Belal A, et al. Spectral, structural and cytotoxicity studies on the newly synthesized n'1,n'3-diisonicotinoylmalonohydrazide and some of its bivalent metal complexes. J Mol Struct. 2022;1251:131960. doi:10.1016/j.molstruc.2021.131960
- Abbasi Tyula Y, Goudarziafshar H, Yousefi S, et al. Template synthesis, characterization and antibacterial activity of d10 (Zn2+, Cd2+, Hg2+) Schiff base complexes: a novel supramolecular Cd2+complex with two 1D helical chains, and its hirshfeld surface analysis. J Mol Struct. 2023;1272:134051. doi:10.1016/j.molstruc.2022.134051
- Mamta S, Pinki AC. In vitro cytotoxicity and antimicrobial evaluation of novel 24–28 membered Schiff base octaazamacrocyclic complexes of manganese(II): synthesis, characterization, DFT and molecular docking studies. J Mol Struct. 2023;1275:134667. doi:10.1016/j.molstruc.2022.134667
- Alqasaimeh M, Abu-Yamin A-A, Matar S, et al. Preparation, spectroscopic investigation, biological activity and magnetic properties of three inner transition metal complexes based on (2-((p-tolylimino)methyl)phenol) Schiff base. J Mol Struct. 2023;1274:134458. doi:10.1016/j.molstruc.2022.134458
- Elkanzi NAA, Hrichi H, Salah H, et al. Synthesis, physicochemical properties, biological, molecular docking and DFT investigation of Fe(III), Co(II), Ni(II), Cu(II) and Zn(II) complexes of the 4-[(5-oxo-4,5-dihydro-1,3-thiazol-2-yl)hydrazono]methylphenyl 4-methylbenzenesulfonate Schiff-base ligand. Polyhedron. 2023;230:116219. doi:10.1016/j.poly.2022.116219
- Kumar N, Asija S, Deswal Y, et al. Development of new tridentate ligands bearing hydrazone motif and their diorganotin(IV) complexes: synthesis, spectral, antimicrobial and molecular docking studies. Res Chem Intermed. 2022;48(12):5133–5154. doi:10.1007/s11164-022-04860-0
- Thomas AB, Nanda RK, Kothapalli LP, et al. Synthesis and biological evaluation of Schiff’s bases and 2-azetidinones of isonocotinyl hydrazone as potential antidepressant and nootropic agents. Arab J Chem. 2016;9:S79–S90. doi:10.1016/j.arabjc.2011.02.015
- Deswal Y, Asija S, Tufail A, et al. Instigating the in vitro antidiabetic activity of new tridentate Schiff base ligand appended M(II) complexes: from synthesis, structural characterization, quantum computational calculations to molecular docking, and molecular dynamics simulation studies. J. 2023;37(4):e7050.
- Deswal Y, Asija S, Dubey A, et al. Cobalt(II), nickel(II), copper(II) and zinc(II) complexes of thiadiazole based Schiff base ligands: synthesis, structural characterization, DFT, antidiabetic and molecular docking studies. J Mol Struct. 2022;1253:132266. doi:10.1016/j.molstruc.2021.132266
- Kiwaan HA, El-Mowafy AS, El-Bindary AA. Synthesis, spectral characterization, DNA binding, catalytic and in vitro cytotoxicity of some metal complexes. J Mol Liq. 2021;326:115381. doi:10.1016/j.molliq.2021.115381
- El-Lateef HMA, Khalaf MM, Shehata MR, et al. Fabrication, DFT calculation, and molecular docking of two Fe(III) imine chelates as anti-COVID-19 and pharmaceutical drug candidate. Int J Mol Sci. 2022;23(7):3994. doi:10.3390/ijms23073994
- Deswal Y, Asija S, Tufail A, et al. Metal complexes of 1,2,4-triazole based ligand: synthesis, structural elucidation, DFT calculations, alpha-amylase and alpha-glucosidase inhibitory activity along with molecular docking studies. J Inorg Organomet Polym Mater. 2023;33:1–17.
- Singh A, Gogoi HP, Barman P. Synthesis of metal oxide nanoparticles by facile thermal decomposition of new Co(II), Ni(II), and Zn(II) Schiff base complexes-optical properties and photocatalytic degradation of methylene blue dye. Inorg Chim Acta. 2023;546:121292. doi:10.1016/j.ica.2022.121292
- Singh Rana V, Anand V, Shekhar Sarkar S, et al. A novel pyrene-based aggregation induced enhanced emission active Schiff base fluorophore as a selective “turn-on” sensor for Sn2+ions and its application in lung adenocarcinoma cells. J Photochem Photobiol A. 2023;436:114409. doi:10.1016/j.jphotochem.2022.114409
- Suneetha G, Ayodhya D, Sunitha Manjari P. Schiff base stabilized gold nanoparticles: synthesis, characterization, catalytic reduction of nitroaromatic compounds, fluorometric sensing, and biological activities. Results Chem. 2023;5:100688. doi:10.1016/j.rechem.2022.100688
- Liu W, Yan M, Zhao W. Antibacterial-renew dual-function anti-biofouling strategy: self-assembled Schiff-base metal complex coatings built from natural products. J Coll Interf Sci. 2023;629:496–507. doi:10.1016/j.jcis.2022.08.178
- Neshat A, Cheraghi M, Kucerakova M, et al. A Cu(II) complex based on a Schiff base ligand derived from ortho-vanillin: synthesis, DFT analysis and catalytic activities. J Mol Struct. 2023;1274:134545. doi:10.1016/j.molstruc.2022.134545
- Buldurun K, Sarıdağ T. Synthesis of Pd+2 complexes of Schiff bases containing methyl 2-amino-6-benzyl-4,5,6,7-tetrahydrothieno[2,3-c]pyridine-3-carboxylate and spectral and catalytic activities. J Mol Struct. 2023;1273:134278. doi:10.1016/j.molstruc.2022.134278
- Liu JN, Wu BW, Zhang B, et al. Synthesis and characterization of metal complexes of Cu(II), Ni(II), Zn(II), Co(II), Mn(II) and Cd(II) with tetradentate schiff bases. Turk J Chem. 2006;30(1):41–48.
- Vogel AI. Vogle’s text book of quantitative chemical analysis. New York: John Wiely & Sons; 1989, p. 906.
- Svehla G. Vogel’s textbook of macro and semi micro quantitative inorganic analysis. 5th ed. New York: Longman Inc.; 1979.
- Lewis L, Wilkins RG. Modern coordination chemistry. New York: Interscience; 1960.
- Boggess RK, Zatko DA. The use of conductivity data for the structure determination of metal complexes. J Chem Educ. 1975;52(10):649–651. doi:10.1021/ed052p649.
- Frisch MJ, Trucks GW, Schlegel HB, et al. Gaussian 09, revision A02. Wallingford (CT): Gaussian, Inc; 2016.
- Keith T, Millam J. Gaussview, version 5.0.9. Mission (KS): Semichem. Inc.; 2009.
- Lee C, Yang W, Parr RG. Development of the Colle-Salvetti correlation-energy formula into a functional of the electron density. Phys Rev B. 1988;37(2):785–789. doi:10.1103/PhysRevB.37.785
- Becke AD. Density functional thermochemistry. III. The role of exact exchange. J Chem Phys. 1993;98(7):5648–5662. doi:10.1063/1.464913
- Collee JG, Mackie TJ, McCartney JE. Mackie and McCartney practical medical microbiology. 14th ed. New York: Churchill Livingstone; 1996.
- Holder IA, Boyce ST. Agar well diffusion assay testing of bacterial susceptibility to various antimicrobials in concentrations non-toxic for human cells in culture. Burns. 1994;20(5):426–429. doi:10.1016/0305-4179(94)90035-3
- Geary WJ. The use of conductivity measurements in organic solvents for the characterisation of coordination compounds. Coord Chem Rev. 1971;7(1):81–122. doi:10.1016/S0010-8545(00)80009-0
- Eşme A, Sağdınç SG. Spectroscopic (FT–IR, FT–Raman, UV–Vis) analysis, conformational, HOMO-LUMO, NBO and NLO calculations on monomeric and dimeric structures of 4–pyridazinecarboxylic acid by HF and DFT methods. J Mol Struct. 2017;1147:322–334. doi:10.1016/j.molstruc.2017.06.110
- Ermiş E, Durmuş K, Aygüzer ÖU, et al. A new 2,2′-oxydianiline derivative symmetrical azomethine compound containing thiophene units: synthesis, spectroscopic characterization (UV–Vis, FTIR, 1H and 13C NMR) and DFT calculations. J Mol Struct. 2018;1168:115–126. doi:10.1016/j.molstruc.2018.05.021
- Aslan HG, Özcan S, Karacan N. Synthesis, characterization and antimicrobial activity of salicylaldehyde benzenesulfonylhydrazone (hsalbsmh)and its nickel(II), palladium(II), platinum(II), copper(II), cobalt(II) complexes. Inorg Chem Commun. 2011;14(9):1550–1553. doi:10.1016/j.inoche.2011.05.024
- El-Wahab ZHA, Mashaly MM, Salman AA, et al. Co(II), Ce(III) and UO2(VI) bis-salicylatothiosemicarbazide complexes: binary and ternary complexes, thermal studies and antimicrobial activity. Spectrochim Acta Part A. 2004;60(12):2861–2873. doi:10.1016/j.saa.2004.01.021
- Nakamoto K. Infrared and Raman spectra of inorganic and coordination compounds part B: applications in coordination, organometallic, and bioinorganic chemistry, 6th ed. Hoboken: John Wiley & Sons INC; 2009.
- El-Tabl AS, Shakdofa MME, Whaba MA. Synthesis, characterization and fungicidal activity of binary and ternary metal(II) complexes derived from 4,4′-((4-nitro-1,2-phenylene) bis(azanylylidene))bis(3-(hydroxyimino)pentan-2-one). Spectrochim Acta Part A. 2015;136(PC):1941–1949. doi:10.1016/j.saa.2014.10.112
- Papatriantafyllopoulou C, Raptopoulou CP, Terzis A, et al. Reactions of nickel (II) sulfate hexahydrate with methyl(2-pyridyl)ketone oxime: two mononuclear sulfato complexes containing the neutral ligand. Z Naturforsch B. 2007;62(9):1123–1132. doi:10.1515/znb-2007-0904
- Fouda MFR, Abd-Elzaher MM, Shakdofa MM, et al. Synthesis and characterization of a hydrazone ligand containing antipyrine and its transition metal complexes. J Coord Chem. 2008;61(12):1983–1996. doi:10.1080/00958970701795714
- Shakdofa MME, El-Saied FA, Rasras AJ, et al. Transition metal complexes of a hydrazone–oxime ligand containing the isonicotinoyl moiety: synthesis, characterization and microbicide activities. Appl Organomet Chem. 2018;32(7):e4376. doi:10.1002/aoc.4376
- Pavia DL, Lampman GM, Kriz GS, et al. Introduction to spectroscopy. 5th ed. Belmont: Cengage Learning; 2013.
- Kaya İ, Kolcu F, Demiral G, et al. Synthesis and characterization of imine polymers of aromatic aldehydes with 4-amino-2-methylquinoline via oxidative polycondensation. Des Monomers Polym. 2015;18(1):89–104. doi:10.1080/15685551.2014.971395
- Shakdofa MME, Morsy NA, Rasras AJ, et al. Synthesis, characterization, and density functional theory studies of hydrazone–oxime ligand derived from 2,4,6-trichlorophenyl hydrazine and its metal complexes searching for new antimicrobial drugs. Appl Organomet Chem. 2021;35(2):e6111. doi:10.1002/aoc.6111
- Venkatachalam TK, Pierens GK, Reutens DC. Synthesis, NMR structural characterization and molecular modeling of substituted thiosemicarbazones and semicarbazones using DFT calculations to prove the syn/anti isomer formation. Magn Reson Chem. 2014;52(3):98–105. doi:10.1002/mrc.4041
- Pandhurnekar CP, Meshram EM, Chopde HN, et al. Synthesis, characterization, and biological activity of 4-(2-hydroxy-5-(aryl-diazenyl)phenyl)-6-(aryl)pyrimidin-2-ols derivatives. Org Chem Inter. 2013;2013:1–10. doi:10.1155/2013/582079
- Shakdofa MME, El-tabl AS, Al-hakimi AS, et al. Synthesis, structural characterization and microbicide activities of mononuclear transition metal complexes of 2-(2-hydroxy-5-(p-tolyldiazenyl) benzylidene) hydrazinecarbothioamide. Ponte. 2017;73(6):52–74.
- Gup R, Kirkan B. Synthesis and spectroscopic studies of copper(II) and nickel(II) complexes containing hydrazonic ligands and heterocyclic coligand. Spectrochim Acta Part A. 2005;62(4-5):1188–1195. doi:10.1016/j.saa.2005.04.015
- Rageh NM, Mawgoud AMA, Mostafa HM. Electronic spectra, solvatochromic behaviour, and acidity constants of some new azocoumarin derivatives. Chem Pap. 1999;53(2):107–113.
- Reddy SL, Endo T, Reddy GS. Advanced Aspects of Spectroscopy. London: InTech; 2012.
- Sharma R, Agarwal SK, Rawat S, et al. Synthesis, characterization and antibacterial activity of some transition metal cis-3,7-dimethyl-2,6-octadiensemicarbazone complexes. Transition Met Chem. 2006;31(2):201–206. doi:10.1007/s11243-005-6374-3
- Lever ABP. Inorganic electronic spectroscopy. Amsterdam: Elsevier science; 1984.
- Anitha C, Sheela CD, Tharmaraj P, et al. Synthesis and characterization of VO(II), Co(II), Ni(II), Cu(II) and Zn(II) complexes of chromone based azo-linked Schiff base ligand. Spectrochim Acta Part A: Mol Biomol Spectrosc. 2012;98:35–42. doi:10.1016/j.saa.2012.08.022
- Shebl M. Mononuclear, homo- and hetero-binuclear complexes of 1-(5-(1-(2-aminophenylimino)ethyl)-2,4- dihydroxyphenyl)ethanone: synthesis, magnetic, spectral, antimicrobial, antioxidant, and antitumor studies. J Coord Chem. 2016;69(2):199–214. doi:10.1080/00958972.2015.1116688
- Puthilibai G, Vasudhevan S, John Mary S. Synthesis, spectral, electrochemical and DNA binding properties of ruthenium (III) complexes of schiff bases containing N,O donor atoms. Mat Today: Proc. 2021;36:809–813. doi:10.1016/j.matpr.2020.07.008
- Sathyanarayana DN. Electronic absorption spectroscopy and related techniques. Hyderabad: Universities Press; 2001.
- Chandra S, Sharma S. Synthesis and spectral studies of transition metal complexes with 1,5:11,15-dimetheno-2,4,10,12-tetramethyl-[1,5,9,13]-tetraazahexadeca-1,3,5,6, 10,11,13,15,16,20-decene a sixteen-membered tetradentate macrocyclic ligand. Transition Met Chem. 2007;32(2):150–154. doi:10.1007/s11243-006-0117-y
- El-Tabl AS, Shakdofa MME, Herash BM. Antibacterial activities and spectroscopic characterization of synthetic metal complexes of 4-(3-(hydroxyimino)-4-oxopentan-2-ylidene)amino)-1,5- dimethyl-2-phenyl-1H-pyrazol-3(2H)-one. Main Group Chem. 2013;12(3):257–274. doi:10.3233/MGC-130106
- Sreeja PB, Kurup MRP, Kishore A, et al. Spectral characterization, X-ray structure and biological investigations of copper(II) ternary complexes of 2-hydroxyacetophenone 4-hydroxybenzoic acid hydrazone and heterocyclic bases. Polyhedron. 2004;23(4):575–581. doi:10.1016/j.poly.2003.11.005
- Vlad A, Avadanei M, Shova S, et al. Synthesis, structural characterization and properties of some novel siloxane-based bis-Schiff base copper(II), nickel(II) and manganese(II) complexes. Polyhedron. 2018;146:129–135. doi:10.1016/j.poly.2018.02.029
- Bal S, Bal SS. Cobalt(Il) and manganese(II) complexes of novel Schiff bases, synthesis, charcterization, and thermal, antimicrobial, electronic, and catalytic features. Adv Chem. 2014;2014:1–12.
- Dr. Rajnikant. Synthesis and studies on electronic spectra of Ni (II) complexes with 3-hydroxy-2-naphthalidene semicarbazone. Int J Sci Devel Res. 2000;5(3):177–180.
- Alaghaz ANMA, Bayoumi HA, Ammar YA, et al. Synthesis, characterization, and antipathogenic studies of some transition metal complexes with N,O-chelating Schiff's base ligand incorporating azo and sulfonamide moieties. J Mol Struct. 2013;1035:383–399. doi:10.1016/j.molstruc.2012.11.030
- Gandhi JB, Kulkarni ND. Binuclear complexes with uranyl ions in non-equivalent coordination environments: synthesis and electrochemistry of complexes with binucleating aroyl hydrazones and 2,2′-bipyridine. Transition Met Chem. 2000;25(2):209–212. doi:10.1023/A:1007086102556
- El-Saied FA, Shakdofa MME, Al-Hakimi AN. Synthesis, characterization and antimicrobial activities of hydrazone ligands derived from 2-(phenylamino)acetohydrazide and their metal complexes. J Korean Chem Soc. 2011;55(3):444–453. doi:10.5012/jkcs.2011.55.3.444
- Zaky RR, Ibrahim KM, Gabr IM. Bivalent transition metal complexes of o-hydroxyacetophenone [N-(3-hydroxy-2-naphthoyl)] hydrazone: spectroscopic, antibacterial, antifungal activity and thermogravimetric studies. Spectrochim Acta Part A. 2011;81(1):28–34.
- Hathaway BJ, Billing DE. The electronic properties and stereochemistry of mono-nuclear complexes of the copper(II) ion. Coord Chem Rev. 1970;5(2):143–207. doi:10.1016/S0010-8545(00)80135-6
- Nagashri K, Joseph J, Dhanaraj CJ. Copper(II) complexes of hydroxyflavone derivatives as potential bioactive molecule to combat antioxidants: synthesis, characterization and pharmacological activities. Appl Organomet Chem. 2011;25(9):704–717. doi:10.1002/aoc.1831
- Shebl M, El-ghamry MA, Khalil SME, et al. Mono- and binuclear copper(II) complexes of new hydrazone ligands derived from 4,6-diacetylresorcinol: synthesis, spectral studies and antimicrobial activity. Spectrochim Acta Part A. 2014;126:232–241. doi:10.1016/j.saa.2014.02.014
- Al-Hakimi AN, El-Tabl AS, Shakdofa MME. Coordination and biological behaviour of 2-(p-toluidino)-N'-(3-oxo-1,3- diphenylpropylidene) acetohydrazide and its metal complexes. J Chem Res. 2009;12:770–774. doi:10.3184/030823409X12596063380927
- Kivelson D, Neiman R. ESR studies on the bonding in copper complexes. J Chem Phys. 1961;35(1):149–155. doi:10.1063/1.1731880
- Mangalam NA, Prathapachandra Kurup MR. Versatile binding properties of a di-2-pyridyl ketone nicotinoylhydrazone ligand: crystal structure of a Cu(II) complex. Spectrochim Acta Part A. 2011;78(3):926–934. doi:10.1016/j.saa.2010.11.032
- Ray RK, Kauffman GB. An EPR study of some copper(II) coordination compounds of substituted biguanides. Part IV. Inorg Chim Acta. 1990;174(2):257–262. doi:10.1016/S0020-1693(00)80309-6
- Swamy B, Swamy JR. Preparation and characterization of some transition metal complexes of benzoic acid hydrazones of 2-aminonicotinaldehyde. Transition Met Chem. 1991;16(1):35–38. doi:10.1007/BF01127867
- Ray RK, Kauffmann GB. An EPR study of copper(II)-substituted biguanide complexes. Part III. Inorg Chim Acta. 1990;174(2):237–244. doi:10.1016/S0020-1693(00)80306-0
- Shakdofa MM, Abdel Hady Mousa H, Labib AA, et al. In vitro antimicrobial activity evaluation of newly synthesized furochromone schiff base complexes. J Egyptian J Chem. 2018;61(2):295–304.
- El-Tabl AS, Wahed MMA-E, Shakdofa MME, et al. Azo-Schiff base complexes synthesis, spectroscopic characterization and microbicide studies. J Chem Chemical Sci. 2017;7(3):170–191.
- Shakdofa MME, Mousa HA, Elseidy AMA, et al. Anti-proliferative activity of newly synthesized Cd(II), Cu(II), Zn(II),Ni(II), Co(II), VO(II), and Mn(II) complexes of 2-((4,9-dimethoxy-5-oxo-5H-furo[3,2-g]chromen-6-yl)methylene) hydrazinecarbothioamide on three human cancer cells. Appl Organomet Chem. 2018;32(1):e3936. doi:10.1002/aoc.3936
- Refat MS, El-Deen IM, Anwer ZM, et al. Bivalent transition metal complexes of coumarin-3-yl thiosemicarbazone derivatives: spectroscopic, antibacterial activity and thermogravimetric studies. J Mol Struct. 2009;920(1):149–162. doi:10.1016/j.molstruc.2008.10.059
- Yang L, Powell DR, Houser RP. Structural variation in copper(i) complexes with pyridylmethylamide ligands: structural analysis with a new four-coordinate geometry index, τ4. Dalton Trans. 2007;9:955–964. doi:10.1039/B617136B
- Ismael M, Abdou A, Abdel-Mawgoud A-M. Synthesis, characterization, modeling, and antimicrobial activity of FeIII, CoII, NiII, CuII, and ZnII complexes based on tri-substituted imidazole ligand. Z Anorg Allg Chem. 2018;644(20):1203–1214.
- Geerlings P, De Proft F, Langenaeker W. Conceptual density functional theory. Chem Rev. 2003;103(5):1793–1874. doi:10.1021/cr990029p
- Yousef TA, Alduaij OK, Abu El-Reash GM, et al. Semiempirical studies, spectral analysis, in vitro antibacterial and DNA degradation studies of heterocyclic thiosemicarbazone ligand and its metal complexes. J Mol Liquids. 2016;222:762–776. doi:10.1016/j.molliq.2016.07.075
- Masoud MS, Ali AE, Shaker MA, et al. Synthesis, computational, spectroscopic, thermal and antimicrobial activity studies on some metal–urate complexes. Spectrochim Acta Part A. 2012;90:93–108. doi:10.1016/j.saa.2012.01.028
- Beyazit N, Çatıkkaş B, Bayraktar Ş, et al. Synthesis, characterization and catecholase-like activity of new schiff base metal complexes derived from visnagin: theoretical and experimental study. J Mol Struct. 2016;1119:124–132. doi:10.1016/j.molstruc.2016.04.047
- Foresman JB, Frisch A. Exploring chemistry with electronic structure methods: a guide to using Gaussian. Pittsburgh: Gaussian; 1966.
- Fakhar Z, Govender T, Lamichhane G, et al. Computational model for the acylation step of the β-lactam ring: potential application for l,d-transpeptidase 2 in mycobacterium tuberculosis. J Mol Struct. 2017;1128:94–102. doi:10.1016/j.molstruc.2016.08.049
- Ugwu NF, Anarado CJO, Ibeji CU, et al. Synthesis, spectroscopic, antimicrobial activity and computational studies of some homoleptic and heteroleptic metal(II) complexes of 2-furoic acid hydrazone. ChemistrySelect. 2019;4(37):11206–11212. doi:10.1002/slct.201902870
- Sertçelik M. Synthesis, spectroscopic properties, crystal structures, DFT studies, and the antibacterial and enzyme inhibitory properties of a complex of Co(II) 3,5-difluorobenzoate with 3-pyridinol. J Chem Res. 2021;45:42–46.
- Mlahi MR, Afsah EM, Negm A, et al. Synthesis of 8-hydroxyquinolium chloroacetate and synthesis of complexes derived from 8-hydroxyquinoline, and characterization, density functional theory and biological studies. Appl Organomet Chem. 2015;29(4):200–208. doi:10.1002/aoc.3265
- Politzer P, Truhlar DG. Chemical applications of atomic and molecular electrostatic potentials:reactivity, structure, scattering, and energetics of organic, inorganic, and biological systems. 1st ed. New York: Springer; 1981.
- Politzer P, Murray JS. The fundamental nature and role of the electrostatic potential in atoms and molecules. Theor Chem Acc. 2002;108(3):134–142. doi:10.1007/s00214-002-0363-9
- Kumar N, Asija S, Deswal Y, et al. Organotin(IV) complexes derived from hydrazone ligands: synthesis, spectral analysis, antimicrobial and molecular docking studies, phosphorus, sulfur, and silicon and the related elements. Phosphorus Sulfur Silicon Relat Elem. 2022;197(9):952–963.
- Deamer DW, Kleinzeller A, Fambrough DM. Membrane permeability 100 years since Ernest Overton, 1st ed. London: Academic Press; 1999.
- Selwin Joseyphus R, Sivasankaran Nair M. Synthesis, characterization and biological studies of some Co(II), Ni(II) and Cu(II) complexes derived from indole-3-carboxaldehyde and glycylglycine as Schiff base ligand. Arab J Chem. 2010;3(4):195–204. doi:10.1016/j.arabjc.2010.05.001
- Devi J, Kumar S, Kumar B, et al. Synthesis, structural analysis, in vitro antioxidant, antimicrobial activity and molecular docking studies of transition metal complexes derived from Schiff base ligands of 4-(benzyloxy)-2-hydroxybenzaldehyde. Res Chem Intermed. 2022;48(4):1541–1576. doi:10.1007/s11164-021-04644-y
- Devi J, Sharma S, Kumar S, et al. Synthesis, characterization, in vitro antimicrobial and cytotoxic studies of Co(II), Ni(II), Cu(II), and Zn(II) complexes obtained from Schiff base ligands of 1, 2, 3, 4-tetrahydro-naphthalen-1-ylamine. Appl Organomet Chem. 2022;36(8):e6760.
- El-saied FA, Shakdofa MME, Al-Hakimi AN, et al. Transition metal complexes derived from N′-(4-fluorobenzylidene)-2-(quinolin-2-yloxy) acetohydrazide: synthesis, structural characterization, and biocidal evaluation. Appl Organomet Chem. 2020;34(11):e5898.
- Shakdofa MME, Selim QM, Shakdofa AME. Ru3+, Mn2+, Co2+, Ni2+, Cu2+, and Zn2+ uni-metallic complexes of 3-(-(1,5-dimethyl-3-oxo-2-phenyl-2,3-dihydro-1H-pyrazol-4-yl) methylene) hydrazono)indolin-2-one, preparation, structure elucidation and antibacterial activity. J Mol Struct. 2021;1246:131194. doi:10.1016/j.molstruc.2021.131194
- Shakdofa MME, Saleem QM, Shakdofa AME. Structure investigation, density functional theory, and biostudy of synthesized dihydrazone incorporating isatin moiety and its homo-bimetallic complexes. Appl Organomet Chem. 2021;35(11):e6369.
- Hassan EA, Ebrahium MM, Ebrahium AM. Metal complexes of hydrazone–oxime derivative as promising in vitro antimicrobial agents against some fungal and bacterial strains. Appl Organomet Chem. 2022;36(5):e6663. doi:10.1002/aoc.6663
- Saroya S, Asija S, Deswal Y, et al. Synthesis, spectral studies, in vitro antimicrobial activity and molecular docking studies of organotin(IV) complexes derived from tridentate Schiff base ligands. Res Chem Intermed. 2022;48(7):2949–2971. doi:10.1007/s11164-022-04731-8