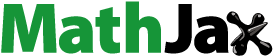
Abstract
This study emphasizes the synthesis of nickel oxide (NiOx + C) nanoparticles with varied graphite (C) proportions (1%, 2.5%, 5%, 7.5%, and 10%) using Aloe-Vera natural extract as a green reducing agent. Prepared NiOx + C nanostructures exhibited consistent X-ray diffraction patterns with no additional C peak, signifying enhanced interlayer spacing. The crystalline size (Dp) decreased from 27 nm to 20 nm with an increase in the amount of carbon (C). The determined bandgap (Eg) was 2 eV higher than bulk material due to formation extra energy level at defect sites. The shoulder peak at 1620 cm−1 agrees with the disorder-induced band from C and second-order bands of C at about 2700 cm−1 for NiOx + C. FESEM images confirm the hexagonal morphology. Not much particle aggregation was observed except for 10% C content. The selected area electron diffraction (SAED) confirms the existence of NiOx crystalline orientations including planes (111), (200), (220), (311), and (222).
1. Introduction
Metal oxides (MOs) of nanosized have comprehensively been engaged intended for numerous key scientific as well as industrial research for getting access towards novel types of efficient resources with unparalleled characteristics and striking uses. In the modern era, MOs semiconductors having extensive optical bandgap (Eg) are being immensely considered to be cast off for numerous applications such as gas or chemical detectors, and magnetic elements by the virtue of their outstanding photosensitivity, mechanical robustness, enhanced electrical, magnetic and opto-catalyst attributes [Citation1–6]. Transitional metal-based oxides are very important in optoelectronic devices [Citation7,Citation8]. Among many MOs, NiOx is a semiconductor with p-type electrical conductivity and a comprehensive optical band gap (Eg of 3.65–4.0 eV) following FCC configuration [Citation9,Citation10]. The NiOx nanoparticle (NP) in conjunction with transparent conducting oxides (TCOs) exhibits excellent electrical properties, making it ideal for photo-responsive devices [Citation11,Citation12]. NiOx nanostructures have been engaged as hole conductors in hetero junction (p-n) devices of oxide (e.g. rectifiers [Citation13], light emitting diodes [Citation14], gas sensing [Citation15]). Earth-abundant NiOx has been reasonably used in further remarkable technological engagement in recent electronic devices like energy storage devices [Citation16] with high energy density, Li-ion batteries [Citation17], and electrochromic phenomena [Citation18]. NiOx NPs hold prospective usages for their remarkable individualities [Citation19]. It has its footprint in surface coating technology for [Citation20] environments, transistors based on thin film [Citation21], arrays in extremely high density for data collection usages [Citation22], temperature sensors, tuned circuits, nickel cermet, etc. [Citation23]. It is mandatory to synthesize pure and excellence crystallite NiOx nanomaterials to get the required features regarding their optoelectronic and morphology for previously mentioned uses. These features seemed to be diligently associated towards the synthesis methods. Governing the crystallographic coordination besides surface smoothness remains very significant while using NiOx nanomaterials or films. Both solution route such as spin coating [Citation24], spray pyrolysis [Citation25], electrochemical deposition [Citation20], sol–gel [Citation26], chemical co-precipitation [Citation27], micro-emulsion [Citation28], solvothermal [Citation29] and vapour deposition techniques like sputtering [Citation30], electron-beam [Citation31], chemical vapour [Citation32], microwave-assisted synthesis [Citation33] and thermal evaporation [Citation34] are available to synthesis high-grade NiOx. Nevertheless, selected approaches involve high vacuum and processing temperatures, severe growth environments, costly investigational arrangements, and long elimination times of solid and lenient residual substances or biological additives. Consequently, emerging an easy method aimed at cost-effective, low heat for processing, commercial-scale manufacturing, and precise growth naturally to formulate NiOx nanoparticles remains a prodigious contest for material technologists. Among these methods, the sol–gel process is a widely adopted technique for nanoparticle synthesis, offering inherent advantages such as minimal requirement for processing heat, ensuring high purity, and consistent compound properties [Citation35–38]. These attributes of sol–gel method permits stress-free incapacitating of other elements on solution phase, suggest an enhanced elemental arrangement mechanism in a less costly manner [Citation39]. That's why the sol–gel process is preferred here for preparing the precursor for NiOx nanoparticle synthesis, facilitating the investigation of alloying with carbon (C), and enabling further exploration of the material's properties[Citation40–43]. Meanwhile, the inferior actual specific capacitance originates comparatively deprived electronic conductivity in addition of poor effective surface sites in NiOx nanoparticles. Many attempts are considered by this time to improve the optoelectronic plus morphological factors of different NiOx forms [Citation44]. On the contrary, insertion of hetero-atom in their different concentrations into intrinsic MO matrix along with different synthesis protocol/parameter has been proven as a suitable alternative to tune optoelectronic and morphological properties of pristine one. Lin et al. showed that N doping could offer better crystalline structure and greater superficial irregularity for NiOx which had been agreed by other research groups as well [Citation45]. Here we proposed insertion of graphite (C) into NiOx nanoparticle to formulate NiOx + C matrix by sol–gel technique with different concentrations of C element to perform a brief study on the effect of C content into the mentioned matrix. Therefore, the central point of the present analysis remains examining the significant part of C in green synthesized NiOx nanoparticles referring to photo-physical and morphological attributes.
2. Experimental details
2.1 Materials
Materials: Graphite (C) (powder <20 µm, synthetic), Nickel nitrate hexahydrate [Ni(NO3)2.6H2O] (Purum p.a., crystalized ≥ 98.5%), isopropyl alcohol (IPA) (natural, ≥ 98%, FG) were purchased from Sigma Aldrich Lt., and Aloe-Vera (locally collected), it's water extract (AE) was prepared as the following description.
2.2 Methodology
2.2.1 Collection of aloe-Vera (AE) extraction
As shown in Figure , collected Aloe-Vera stems were washed several times by DI (Distilled water) water, dried in normal conditions, and made into slices. Each 15 g Aloe-Vera slice was added to 100 mL DI water in a glass beaker and was stirred at 60°C for 60 min on a hot plate. After the extraction, the mixture was filtered, and the filtrate was stored in the refrigerator to use as a chelating or complexing agent for the synthesis process.
2.2.2 Preparation of NiOx nanoparticles
In order to prepare NiOx using the green approaching sol–gel method, 14.54 g of Ni(NO3)2. 6H2O (0.05 mol) and 100 mL of AE were taken in a 500 mL beaker. The beaker was set on a hotplate and continuously stirred at a temperature 120°C. When the mixture finally formed a gel and got denser, it dried at 150°C for 12 h before being ground into the final NiOx.
2.3 Preparation of NiOx + C nanoparticles
Different proportions of graphite and green synthesized NiOx were used to develop the composite materials. Figure illustrates the preparation of NiOx + C nanoparticles. First, a 500 mL beaker containing 1 g of NiOx, 0.1 g (10%) of commercial C, and 50 mL of Isopropyl alcohol (IPA) were filled. The mixture was gently agitated before being dried at 100°C in a vacuum oven, followed by calcination at 500°C (6 h). Following a similar process, five more composites were prepared, using C amounts of 0.01 g (1%), 0.025 g (2.5%), 0.05 g (5.0%), 0.075 g (7.5%) and 0.1 g (10%). All calcined samples were subsequently collected for characterisation.
3. Results and discussions
3.1. XRD Exploration
Figure displays XRD analysis of NiOx + C complex containing 1%, 2.5%, 5%, 7.5% and 10% C in the configuration. Altogether, the deflection peaks seemed at angles about 37.25°, 43.29°, 62.90°, 75.65° and 79.72° which stayed indexed to (111), (200), (220), (311) and (222) orientations, respectively (JCPDS 00-044-1159, Rhombohedral, R-3m) [Citation46,Citation47] of NiOx. The non-stoichiometric 200 crowning is the leading main peck overhead of all aimed at individual portions of C content. Non-stoichiometric elemental composition in a semiconductor, for example, oxygen vacancies due to carbon insertion, may be responsible for influencing p-type semiconductor attributes of NiOx [Citation48]. The formation of such defect states might initiate a quality decrease in the NiOx films’ crystalline at higher C content, which could be validated in crystallite size reduction from Table . Calculated lattice constants (a) of all prepared different NiOx + C matrix expressed well treaty JCPDS No. 00-047-1049. Ahmed et al. [Citation49] and Jassim et al. [Citation50] reported such phenomena, which are acknowledged by the current work. The film crystalline parameters, such as lattice constant (a), crystallite size (Dp), dislocation density (δ) and full-width half maximum (FWHM), were determined from the XRD profile data. Brag's law and Scherrer equation were engaged to determine the lattice parameter “a” and particle size of the corresponding plane (200) for the prepared NiOx and NiOx + C nanoparticles using Equations 1, 2 and 3 [Citation31]. Bragg's Law for X-ray diffraction is:
(1)
(1) The equation for calculating the lattice parameter “a” for a crystal lattice based on the Miller indices (hkl) is given by:
(2)
(2)
(3)
(3) The Scherrer equation estimates the average size of crystallites from the following equation:
(4)
(4)
Figure 3. XRD pattern (a–e) and shift (f) of different percentages of C in NiOx + C composite materials.

Table 1. XRD data analysis of NiOx + C composite containing different C content considering 200 as primary crystal orientation.
The microstrain (ε) can be calculated using the formula:
(5)
(5) The equation for estimating dislocation density (δ) based on XRD data is:
(6)
(6) Where “λ” signifies the X-ray wavelength (0.15406 nm), “d” is atomic interplanar space, K is the shape factor, “θ” is Brag’s angle between approaching X-ray and scattering planes, “a” refers to lattice constant, and β is FWHM at 2θ of diffraction peak. Highly pure NiOx + C composite realization might be endorsed since any supplementary diffractogram was absent. The comparatively overextended peaks represented the formation of a small Dp [Citation51].
The XRD configurations, as illustrated in Figure f, depict a noticeable change in the diffractogram at the (200) peak position. The C insertion into NiOx might initiate internal mechanical stress, causing the peak position to be shifted marginally [Citation52]. The 200 crystal orientation maxima negatively shifted towards lower angles (from 43.3° to 43.15°), proposing stretched interplanar spaces amongst highly crystallized NiOx + C parallel crystallographic planes [Citation53]. A feeble and wide C diffractogram at 26.5° is witnessed in NiOx + C examples, as illustrated in Figure . This endorses the presence of unstructured C within a specific matrix [Citation9]. The analysis indicates that the lattice parameters remain nearly constant despite increasing carbon content. However, the average particle size demonstrates sensitivity to the variation in carbon content. Specifically, as the carbon content increases, the particle size initially increases up to 5%, followed by a decrease. It can be observed that initially, FWHM gets reduced to 5% C content, providing better crystallite values.
Further increment of the C content (7.5% and 10%) produces increased FWHM values, leading to the decay of the crystallite size of nanoparticles. The highest and lowest Dp values, 27 and 20.35 nm were achieved for 5% and 10% C, respectively. Reduced Dp might be due to excess C in the lattice. This trend suggests that higher carbon content contributes to the formation of smaller particle sizes within the NiOx. The δ and ϵ values likewise reached the lowest 1.4 × 1011 cm−2 and 3.51 × 1011 cm−2, respectively, for high Dp values (5% C), indicating that insertion of higher C in NiOx matrix caused Dp reduction also increased the flaws in the nanoparticle atmosphere [Citation54].
Moreover, the observed strain and dislocation density fluctuations coincide with higher carbon percentages. This tendency implies a potential rise in lattice strain and structural irregularities within the composite material as the carbon concentration increases. Overall, these findings suggest that higher carbon content not only influences particle size but also affects the structural integrity of the NiOx, leading to potential lattice distortions and increased dislocation density.
3.2. Optical property study
UV-Vis spectra of green synthesized NiOx + C complex were logged within 300–900 nm wavelength, as shown in Figure . The compounds were homogeneously dispersed in distilled water by ultra-sonication for 15 min before running the spectrophotometer. The green synthesized NiOx + C NPs show absorption edge shifting of 256, 248, 254, 246 and 236 nm for 1%, 2.5%, 5%, 7.5% and 10% C content, respectively. This phenomenon could be consigned to the captivation of light at inherent Eg. These peaks confirm electronic transitions occurring in the presence of light spectrum moving from the valence band (VB) of O(p) to conduction band (CB) of Ni d() [Citation55,Citation56]. The Eg was designed utilizing the Tauc relation:
(5)
(5) Where α denotes the absorption coefficient, “A” stands for constant, and the “n” exponent rests on photosensitive transition. The plot concerning (αhυ)2 vs photon energy (hυ) had been designed for NiOx + C compounds shown in Figure on account of direct band transition. The extrapolation of the linear portion of the curve in the higher energy zone, intercepting the X-axis, provides the bandgap energy (Eg) of the NiOx + C matrix. An increase in absorption towards the higher energy range may suggest the emergence of a direct bandgap. Although NiOx typically exhibits an indirect bandgap, graphene alloying significantly alters the band structure, resulting in the formation of a direct bandgap. A clear linear extrapolation at higher energies in the Tauc plot also indicates a direct bandgap. This method helps estimate the bandgap energy and infer the nature of the bandgap (direct or indirect) [Citation57,Citation58]. The Eg obtained are 5.35, 5.43, 5.63, 5.4, and 5.33 eV with increasing the C content (Figure ). The extended Eg of NiOx + C is higher than the bulk (3.65-4.00 eV) on account of quantum confinement impact with further Eg of ∼2 eV [Citation59] also from chemical imperfections or vacancies occurring laterally at crystal or grain boundaries of metal oxide matrix creating different energy level to rise Eg [Citation60–64].
3.3. Raman analysis
Vibrational spectroscopy has been nominated as a super-efficient measure in categorizing the phase along with detecting C in a complex [Citation65]. The Raman spectroscopy of samples within the 200–2700 cm−1 wavenumber range was conducted. The Raman spectra of NiOx + C nanoparticles with various C concentrations are shown in Figure . Only the TO (Transverse Optical) and LO (longitudinal-optical) modes in Raman spectra have been detected; this may be because of the deprived foundation of Dp sizes. The presence of nickel defects, i.e. Ni3+ ions in the NiOx + C complex, is indicated by the 1st order slanting mode TO located on 480 cm−1 [Citation66]. A substantial collaboration amid nickel and oxygen bonding is revealed by the tougher 2nd order transverse mode at 1100 cm−1, represented by a 2LO (two-longitudinal-optical) [Citation67]. In this research, we have seen that there is a slight shift in the spectra towards higher wavelength caused by the existence of strain within NiOx + C complex. The strain may be developed due to the insertion of C-atoms in the films. The 1620 cm−1 shoulder peak resembles an added disorder-related band originating from graphite. A 2nd order band of C near 2700 cm−1 was observed for NiOx + C [Citation68].
3.4. EDX and FESEM investigation
Figure (a) illustrates the EDX analysis depicting individual component mapping and confirming the presence of carbon (C) in the NiOx + 5%C composites. The EDX spectrum displayed a carbon (C) peak within the NiOx sample, with the inset depicting their respective elemental compositions. Therefore, Figure (b) demonstrates EDX mapping of elements as performed. It expresses regular carbon dissemination in NiOx + C complexes, reflecting the findings from XRD. The empirical formula from the EDX analysis is calculated as NiO0.67C0.17.
The morphology of NiOx + C carrying at different C quantities is illustrated in Figure (a–e). All concentrations of C in NiOx + C were quite able to form the morphology of nanocrystals, but lower (1%) and higher (10%) C produced a bit of nanoparticle aggregation observed in Figure (a,e), which disappear with intermediate C content [Figure (b–d)]. Again, higher elements seem identical and regular profiles with marginally confined size scattering. The particle size distribution curve shows that most of the nanoparticles range from 30–40 nm (Figure ). However, the morphology was not affected radically by the increase in the concentration of C from 1% to 10% by restricting the nanoparticle's individuality. This study is directed towards preparing NiOx + C composite using natural reducing agents for innovative usages such as photocatalysts and air purifiers by withdrawing volatile organic compounds, decontaminating, and separating natural and industrial gases.
3.5. Morphological studies by TEM
The morphology of the synthesized NiOx + C complex was additionally analyzed through TEM. The nanoparticles seem to be accumulated; nonetheless, every element possesses an external coating (Figure a). At the early crystallisation stage, the C element acts as a coating agent to cover the particles, restricting them from taking the place of Ostwald coarsening. As a result, the particle size exhibits minimal deviation for each change in C content. The TEM image determined an average particle size of approximately 23 nm. Selected area electron diffraction (SAED) pattern of NiOx + C matrix is displayed in Figure b. The occurrence of (111), (200), (220), (311) and (222) crystalline orientation matching to FCC conformation and polycrystalline nature of NiOx was confirmed harmonizing with mentioned the XRD findings.
4. Conclusions
Nickel oxide (NiO) is a notable semiconductor material extensively investigated for its diverse applications in electronics, energy storage, and catalysis due to its unique electronic, and optical properties. Graphite (C) incorporated NiOx nanocomposite were effectively attained via a simple, cost-effective liquid-based sol–gel route, providing an advantageous approach for scalable production. There were no obvious C individual peaks seen from XRD analysis, except at 26.5° for the highest 10% C content relating to the association of the free C presence in NiOx + C structure. The determined Dp values fall within the 21–27 nm range. FTIR spectroscopy exploration similarly established the NiOx segment and survival of C. Moreover, FESEM and EDX plotting were performed for 5% C content sample, validating the findings related to XRD analysis. FESEM assured morphology to hexagonal nanocrystal structure without much noticeable particle agglomeration with slight variation in particle size distribution. The Eg rested with 5.3–5.65 eV through an added Eg∼1.5 eV elevation from bulk values. While pristine NiOx possesses an indirect bandgap, modifications through doping or alloying are known to alter its band structure, potentially transitioning it to a direct bandgap semiconductor. Disorder-induced bands from carbon and second-order carbon bands at 1620 and 2700 cm−1 were observed. EDX confirmed the presence of carbon, with FESEM images showing hexagonal morphology and minimal particle aggregation except at 10% C. SAED confirmed NiOx crystalline orientations. The investigation aims to shed light on the impact of carbon doping on the resulting material's electronic structure and potential applications in advanced electronic devices and energy-related technologies.
Acknowledgements
The authors wish to express gratitude for the financial support provided by The National University of Malaysia via the DIP-2021-026 research grant. The authors would also like to express their sincere appreciation to the Researchers Supporting Project number (RSPD2024R579) at King Saud University, Riyadh, Saudi Arabia.
Disclosure statement
No potential conflict of interest was reported by the author(s).
References
- Hossain MI, et al. Electrical and optical properties of nickel-oxide films for efficient perovskite solar cells. Small Meth. 2020;4(9):2000454), doi:10.1002/smtd.202000454
- van de Krol R, Liang Y, Schoonman J. Solar hydrogen production with nanostructured metal oxides. J Mater Chem. 2008;18(20):2311–2320. doi:10.1039/b718969a
- Wu HB, et al. Nanostructured metal oxide-based materials as advanced anodes for lithium-ion batteries. Nanoscale. 2012;4(8):2526–2542. doi:10.1039/c2nr11966h
- Sajjad L, et al. Facile synthesis and electrochemical study of NiMnO3/CNT/PANI ternary hybrid as a high-performance supercapacitor electrode material. Journal of Energy Storage. 2023;72:108351, doi:10.1016/j.est.2023.108351
- Mahmood K, et al. An electrochemical investigation of methanol oxidation on thin films of nickel oxide and Its composites with zirconium and yttrium oxides. Crystals (Basel). 2022;12(4):534), doi:10.3390/cryst12040534
- Ahmed S, et al. Semiconducting composite oxide Y2 CuO4–5CuO thin films for investigation of photoelectrochemical properties. Dalton Trans. 2014;43(22):8523–8529. doi:10.1039/C4DT00719K
- Sagadevan S, Podder J. Investigations on structural, optical, morphological and electrical properties of nickel oxide nanoparticles. Intern J Nanopartic. 2015;8(3-4):289–301.
- Hasan AM, et al. Air-stable perovskite photovoltaic cells with low temperature deposited NiOx as an efficient hole-transporting material. Opt Mater Express. 2020;10(8):1801–1816. doi:10.1364/OME.391321
- Anandan K, Rajendran V. Structural, optical and magnetic properties of well-dispersed NiO nanoparticles synthesized by CTAB assisted solvothermal process. Nanosci Nanotechnol Int J. 2012;2(4):24–29.
- Niedermeier CA, et al. Band gap bowing in NixMg1− xO. Sci Rep. 2016;6(1):1–9. doi:10.1038/s41598-016-0001-8
- Wu QN, et al. Bioinspired sea-sponge nanostructure design of Ni/Ni (HCO3) 2-on-C for a supercapacitor with a superior anti-fading capacity. J Mater Chem A. 2018;6(32):15781–15788. doi:10.1039/C8TA05303K
- Sheikh F, et al. Fabrication of a nickel sulfide/nickel oxide heterostructure for efficient electrochemical oxidation of methanol. New J Chem. 2023;47(38):17970–17983. doi:10.1039/D3NJ02855K
- Li J-S, et al. Temperature dependence of on–off ratio and reverse recovery time in NiO/β-Ga2O3 heterojunction rectifiers. J Vacuum Sci Technol A Vacuum Surf Films. 2022;40(6):063407), doi:10.1116/6.0002186
- Adhikary A, et al. Light intensity and efficiency enhancement of n-ZnO/NiO/p-GaN heterojunction-based white light-emitting diodes using micro-pillar array. J Opt. 2022: 1–12.
- Drozdowska K, et al. Combined chemoresistive and in situ FTIR spectroscopy study of nanoporous NiO films for light-activated nitrogen dioxide and acetone gas sensing. Sens Actuat B Chem. 2022;353:131125), doi:10.1016/j.snb.2021.131125
- Otun KO, et al. Double linker MOF-derived NiO and NiO/Ni supercapacitor electrodes for enhanced energy storage. Colloids Surf A Physicochem Eng Aspects. 2022;634:128019.
- Iftikhar M, et al. Current advances and prospects in NiO-based lithium-ion battery anodes. Sustain Energy Technol Assess. 2022;53:102376), doi:10.1016/j.seta.2022.102376
- Tao X, et al. High performance NiOx nanoplatelet based films by a scrape-coating method for bifunctional electrochromic and energy storage devices. Mater Adv. 2022;3(21):7881–7893.
- Carbone M. NiO-Based electronic flexible devices. Appl Sci. 2022;12(6):2839), doi:10.3390/app12062839
- Zhou G, et al. Progress of NiO-based anodes for high-performance Li-Ion batteries. Chem Rec. 2022;22(10):e202200111), doi:10.1002/tcr.202200111
- Ouyang Z, et al. Research progress of p-type oxide thin-film transistors. Materials (Basel). 2022;15(14):4781), doi:10.3390/ma15144781
- Nandy S, et al. Current transport mechanism at metal–semiconductor nanoscale interfaces based on ultrahigh density arrays of p-type NiO nano-pillars. Nanoscale. 2013;5(23):11699–11709.
- Danjumma SG, Abubakar Y, Suleiman S. Nickel oxide (NiO) devices and applications: a review. Int J Eng Res Technol. 2019;8:12–21.
- Aswathy N, et al. Structural, optical, and magnetic properties of Mn-doped NiO thin films prepared by sol-gel spin coating. Mater Chem Phys. 2022;282:125916), doi:10.1016/j.matchemphys.2022.125916
- Aftab M, et al. Optical and electrical properties of NiO and Cu-doped NiO thin films synthesized by spray pyrolysis. Opt Mater (Amst). 2021;119:111369), doi:10.1016/j.optmat.2021.111369
- Dhas SD, et al. Sol-gel synthesized nickel oxide nanostructures on nickel foam and nickel mesh for a targeted energy storage application. J Energy Storage. 2022;47:103658), doi:10.1016/j.est.2021.103658
- Khalaj M, Golkhatmi SZ, Sedghi A. High-performance supercapacitor electrode materials based on chemical co-precipitation synthesis of nickel oxide (NiO)/cobalt oxide (Co3O4)-intercalated graphene nanosheets binary nanocomposites. Diam Relat Mater. 2021;114:108313, doi:10.1016/j.diamond.2021.108313
- Narender SS, et al. Nickel oxide nanoparticles: A brief review of their synthesis, characterization, and applications. Chem Eng Technol. 2022;45(3):397–409. doi:10.1002/ceat.202100442
- Kumar V, et al. Solvothermal growth of ultrathin nonporous nickel oxide nanosheets for ethanol sensing. J Mater Sci Mater Electron. 2021;32(1):818–826. doi:10.1007/s10854-020-04860-z
- Elmassi S, et al. Effect of annealing on structural, optical and electrical properties of nickel oxide thin films synthesized by the reactive radio frequency sputtering. Phys B Condens Matter. 2022;639:413980), doi:10.1016/j.physb.2022.413980
- Hasan AM, et al. Optoelectronic properties of electron beam-deposited NiOx thin films for solar cell application. Resul Phys. 2020;17:103122), doi:10.1016/j.rinp.2020.103122
- Kang J-K, Rhee S-W. Chemical vapor deposition of nickel oxide films from Ni (C5H5) 2/O2. Thin Solid Films. 2001;391(1):57–61. doi:10.1016/S0040-6090(01)00962-2
- Prasad N, Veillon F, Prellier W. Raman spectroscopic and magnetic properties of Europium doped nickel oxide nanoparticles prepared by microwave-assisted hydrothermal method. J Alloys Compd. 2021;858:157639), doi:10.1016/j.jallcom.2020.157639
- Mudgal S, et al. Carrier transport mechanisms of nickel oxide-based carrier selective contact silicon heterojunction solar cells: Role of wet chemical silicon oxide passivation interlayer. Solid State Commun. 2021;334:114391.
- Aisida SO, et al. Biogenic synthesis of zinc oxide nanorods for biomedical applications and photodegradation of Rhodamine B. Mater Today Commun. 2022;33:104660), doi:10.1016/j.mtcomm.2022.104660
- Arinzechukwu CE, et al. Polyethylene glycol capped nickel–zinc ferrite nanocomposites: structural, optical and magnetic properties suitable for hyperthermia applications. Appl Phys A. 2022;128(12):1088), doi:10.1007/s00339-022-06248-8
- Akpojevwa TN, et al. In-vitro biosynthesis of concentration-induced nickel oxide nanoparticles for antibacterial applications. Hybr Adv. 2023: 100054.
- Nwabunwanne C, et al. Green biosynthesis of silver nanoparticles from ocimum gratissimum for bactericidal applications. BioNanoScience. 2023: 1–11.
- Abothu IR, et al. Low-cost embedded capacitor technology with hydrothermal and sol-gel processes. In 9th international symposium on advanced packaging materials: processes, properties and interfaces (IEEE Cat. No. 04TH8742). 2004 Proceedings. 2004. IEEE.
- Onyedikachi OA, et al. Zinc ferrite nanoparticles capped with Gongronema latifolium for moderate hyperthermia applications. Appl Phys A. 2022;128(2):95, doi:10.1007/s00339-021-05244-8
- Aisida SO, et al. Biogenic synthesis enhanced structural, morphological, magnetic and optical properties of zinc ferrite nanoparticles for moderate hyperthermia applications. J Nanopart Res. 2021;23:1–14. doi:10.1007/s11051-020-05135-8
- Aisida SO, et al. Calcination induced PEG-Ni-ZnO nanorod composite and its biomedical applications. Mater Chem Phys. 2020;255:123603, doi:10.1016/j.matchemphys.2020.123603
- Aisida SO, et al. Synthesis of intrinsic, Manganese and magnesium doped cobalt ferrite nanoparticles: Physical properties for antibacterial activities. Hybr Adv. 2023: 100049.
- Yamauchi Y, et al. Erratum:“Study of Novel Floating-Gate Oxide Semiconductor Memory Using Indium–Gallium–Zinc Oxide for Low-Power System-on-Panel Applications”. Jpn J Appl Phys. 2014;53(8):089201, doi:10.7567/JJAP.53.089201
- Liu T, et al. Hierarchical flower-like C/NiO composite hollow microspheres and its excellent supercapacitor performance. J Power Sources. 2017;359:371–378. doi:10.1016/j.jpowsour.2017.05.100
- Qiao H, et al. Preparation and characterization of NiO nanoparticles by anodic arc plasma method. J Nanomater. 2009.
- Talebian N, Kheiri M. Sol–gel derived nanostructured nickel oxide films: effect of solvent on crystallographic orientations. Solid State Sci. 2014;27:79–83. doi:10.1016/j.solidstatesciences.2013.11.010
- Pizzini S, Morlotti R. Thermodynamic and transport properties of stoichiometric and nonstoichiometric nickel oxide. J Electrochem Soc. 1967;114(11):1179, doi:10.1149/1.2426441
- Ahmed AA, Devarajan M, Afzal N. Effects of substrate temperature on the degradation of RF sputtered NiO properties. Mater Sci Semicond Process. 2017;63:137–141. doi:10.1016/j.mssp.2017.02.017
- Jassim SA-J, Zumaila AARA, Al Waly GAA. Influence of substrate temperature on the structural, optical and electrical properties of CdS thin films deposited by thermal evaporation. Resul Phys. 2013;3:173–178. doi:10.1016/j.rinp.2013.08.003
- Comini E, et al. Stable and highly sensitive gas sensors based on semiconducting oxide nanobelts. Appl Phys Lett. 2002;81(10):1869–1871. doi:10.1063/1.1504867
- Addonizio M, Diletto C. Doping influence on intrinsic stress and carrier mobility of LP-MOCVD-deposited ZnO: B thin films. Solar Energy Mater Solar Cells. 2008;92(11):1488–1494. doi:10.1016/j.solmat.2008.06.013
- Zheng Y-Z, et al. Iodine-doped ZnO nanocrystalline aggregates for improved dye-sensitized solar cells. Chem Mater. 2011;23(1):3–5. doi:10.1021/cm101525p
- Shkir M, et al. A facile one-step flash combustion synthesis and characterization on C doped NiO nanostructures. Mater Sci Semicond Process. 2019;100:106–112. doi:10.1016/j.mssp.2019.04.038
- Kumar V, et al. Tunable and white emission from ZnO: Tb3 + nanophosphors for solid state lighting applications. Chem Eng J. 2014;255:541–552. doi:10.1016/j.cej.2014.06.027
- Lenglet M, et al. Investigation of the chemical bonding in 3d8 nickel (II) charge transfer insulators (NiO, oxidic spinels) from ligand-field spectroscopy, Ni 2p XPS and X-ray absorption spectroscopy. Solid State Commun. 1997;104(12):793–798
- Munawar K, et al. Effect of deposition temperature on topography and electrochemical water oxidation of NiO thin films. Thin Solid Films. 2023;782:140031, doi:10.1016/j.tsf.2023.140031.
- Vijaya Kumar P, Ahamed AJ, Karthikeyan M. Synthesis and characterization of NiO nanoparticles by chemical as well as green routes and their comparisons with respect to cytotoxic effect and toxicity studies in microbial and MCF-7 cancer cell models. SN Appl Sci. 2019;1:1–15.
- Roduner E. Size matters: why nanomaterials are different. Chem Soc Rev. 2006;35(7):583–592. doi:10.1039/b502142c
- Soriano L, et al. The electronic structure of mesoscopic NiO particles. Chem Phys Lett. 1993;208(5-6):460–464. doi:10.1016/0009-2614(93)87173-Z
- Chandekar KV, et al. Facile synthesis of Mn-doped ZnO nanoparticles by flash combustion route and their characterizations for optoelectronic applications. J Mater Sci Mater Electron. 2022: 1–21.
- Trabelsi ABG, et al. A comprehensive study on Co-doped CdS nanostructured films fit for optoelectronic applications. J Mater Res Technol. 2022;21:3982–4001. doi:10.1016/j.jmrt.2022.11.002
- Chandekar KV, et al. Significant and systematic impact of yttrium doping on physical properties of nickel oxide nanoparticles for optoelectronics applications. J Mater Res Technol. 2021;15:2584–2600. doi:10.1016/j.jmrt.2021.09.072
- Chandekar KV, et al. A noticeable consistent improvement in photocatalytic efficiency of hazardous textile dye through facile flash combustion synthesized Li-doped ZnO nanoparticles. J Mater Sci Mater Electron. 2021;32:3437–3450. doi:10.1007/s10854-020-05090-z
- Ye X, et al. The effect of nitrogen incorporation on the magnetic properties of carbon-doped ZnO. J Phys D Appl Phys. 2008;41(15):155005), doi:10.1088/0022-3727/41/15/155005
- Marciuš M, et al. Formation and microstructure of nickel oxide films. J Alloys Compd. 2012;541:238–243. doi:10.1016/j.jallcom.2012.07.021
- Salunkhe P, AV MA, Kekuda D. Investigation on tailoring physical properties of Nickel Oxide thin films grown by dc magnetron sputtering. Mater Res Express. 2020;7(1):016427), doi:10.1088/2053-1591/ab69c5
- Pimenta M, et al. Studying disorder in graphite-based systems by Raman spectroscopy. Phys Chem Chem Phys. 2007;9(11):1276–1290. doi:10.1039/B613962K