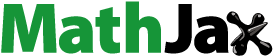
Abstract
Nanofluids have garnered significant interest as a potential solution to address overheating challenges across diverse industries. Researchers are actively exploring different types of nanofluids to mitigate these issues. In this study, a numerical analysis was conducted using ANSYS software to examine the fluid flow and heat transfer characteristics of nanofluids (ranging from 0 to 4 volume fractions) in a corrugated wavy channel within the turbulent range. Various nanoparticles, including Al2O3, CuO, SiO2, and ZnO, were employed and dispersed in different base fluids such as ethylene glycol, glycerin, and water. The particle size of the nanoparticles ranged from 20 to 70 nm. The results revealed that SiO2/water-based nanofluids provide better heat transfer than all the water-based nanofluids. The maximum average Nusselt number and pressure drop of 200 and 115 Pa, respectively, were observed for SiO2 nanofluid (volume fraction of 0.04) with a particle size of 20 nm.
Highlights
Al2O3, CuO, SiO2 and ZnO considered in this work.
Corrugated plates with three different corrugated angles of 20° to 60° are tested.
Eexperiments are performed for different heat flux in turbulent range.
Maximum heat transfer obtained for SiO2/water nanofluid.
Nomenclature
A | = | Area, |
a | = | Component of Vector |
Al | = | Aluminum |
= | Aluminum Oxide | |
CuO | = | Copper Oxide |
= | Turbulent Model Constant | |
= | Turbulent Model Constant | |
= | Specific Heat, kJ/kg.K | |
= | Skin – Friction Coefficient | |
CFD | = | Computational Fluid Dynamics |
CNT | = | Carbon Nanotube |
= | Hydraulic Diameter, mm; Dh = Hmax + Hmin | |
= | Diameter of Nanofluid Particles | |
= | Equivalent Diameter of a Base Fluid Molecule | |
E | = | Enhancement Ratio |
E | = | Total Energy, J, |
EG | = | Ethylene Glycol |
f | = | Friction Factor |
fa | = | Face |
FVM | = | Finite Volume Method |
h | = | Heat Transfer Coefficient, W/ |
H | = | Height of Channel, mm |
Hmin | = | Height of the Bottom Channel, mm |
Hmax | = | Height of the Top Channel, mm |
Hw | = | Height of Wavy, mm |
I | = | Turbulent Intensity |
J | = | Colburn Factor |
k | = | Thermal Conductivity of the Fluid, W/m.K |
k | = | Turbulent Kinetic Energy |
= | Turbulence Characteristics Length = 0.07(Dh/2) | |
M | = | Molecular Weight of Base Fluid |
n | = | Direction Normal to the Surface |
N | = | Avogadro Number = 6.022 * |
Nu | = | Nusselt Number = |
np | = | Adjacent Cells |
= | Pressure Drop, Pa | |
p | = | Wetted Perimeter, m |
= | Difference Between the Correct Pressure Field | |
Pr | = | Prandtl Number, Pr = |
Q | = | Heat Transfer Rate, kW |
q | = | Heat Flux, W/ |
Re | = | Reynolds Number, Re = |
= | Silicon Dioxide | |
T | = | Temperature, K |
= | Reference Temperature, 293K | |
u | = | Velocity Component at x-Direction |
U | = | Mean Velocity Distribution at x-Direction |
= | Velocity Fluctuation | |
= | The SIMPLE Algorithm Pseudo – Velocity = | |
v | = | Velocity Component at y-Direction |
V | = | Mean Velocity Distribution at y-Direction |
= | The SIMPLE Algorithm Pseudo – Velocity = | |
= | Velocity Fluctuation Components | |
X | = | Distance Along the x- Coordinate |
ZnO | = | Zinc Oxide |
Greek Symbols
= | Fraction of Liquid Volume Travelling with a Particle | |
= | Dynamic Viscosity of the Fluid (pas) | |
= | Density, kg/ | |
= | Wavy Angle | |
= | Length of Corrugated, mm | |
= | Length of Domain, mm | |
= | Particle Volume Fraction (%) | |
= | Scalar Variable | |
= | Dissipation Kinetic Energy, | |
= | Diffusion Prandtl Number for k | |
= | Wall Shear Stress | |
κ | = | Boltzmann Constant, 1.3807* |
ϕ | = | Cell |
Subscripts
cr | = | Cross Section |
corr | = | Corrugated Channel |
s | = | Start Point of Corrugated Wall |
stra | = | Straight Channel |
in | = | Inlet |
ave | = | Average |
out | = | Outlet |
e | = | End Point of Corrugated Wall |
eff | = | Effective |
f | = | Base Fluid |
nf | = | Nanofluid |
min | = | Minimum |
max | = | Maximum |
m | = | Mean |
p | = | Particles |
w | = | Wall |
x | = | Local Value |
b | = | Bulk |
i,j | = | Sub Index |
Superscript
* | = | Initial Value |
1. Introduction
A significant and pervasive industrial challenge involves enhancing thermal performance and heat transfer rates, mainly due to the limited thermal conductivity of conventional fluids like ethylene glycol (EG), oil, and water. This limitation imposes constraints on improving heat transfer. Recently, researchers have explored a longstanding topic by integrating solid particles, such as Ag, CuO, Al2O3, and TiO2 at the nano-scale, into traditional base fluids, resulting in what is widely known as nanofluid. Additionally, an alternative method for boosting the heat transfer rate involves modifying the pipe's surface through the introduction of corrugations.
Numerous articles have addressed the crucial topic, utilizing both experimental measurements and numerical simulations. Esmaeili et al. [Citation1] demonstrated Al2O3 flow within a wavy conduit through a numerical approach, employing the control volume scheme to investigate the effects of the conduit's aspect ratio and dimensionless Reynolds number (Re). Their findings indicated an enhancement in heat transfer rate with the addition of nanoparticles. Elshafei et al. [Citation2] conducted experimental research to assess the influence of phase shift and corrugated spacing in corrugated channels, focusing on Re ranging from 3220 to 9420. Their study emphasized the effects on pressure drop and heat transfer, revealing that corrugated channels outperformed flat channels in heat transfer enhancement. Ahmed et al. [Citation3] utilized the finite difference approach to explore the pressure drop and heat transfer characteristics of CuO nanofluid in a corrugated pipe across varying Re and volume fractions. Their results suggested an increase in heat transfer rate with higher Re and volume fractions, accompanied by a slight rise in pressure drop. El-Sebaii et al. [Citation4] conducted a combined numerical and experimental study on the V-type corrugated pipe of a solar air heater, demonstrating superior thermal-hydraulic efficiency compared to a flat pipe.
Studies on fluid flows within straight channels have been extensively explored. For instance, [Citation5] investigated the impact of different nanoparticles (CuO, Al2O3, and TiO2) on heat transfer enhancement, highlighting CuO's superior performance in increasing the Nusselt number (Nu). In a related study, [Citation6] employed a single and two-phase nanofluid model using the finite volume formulation to analyze the turbulent flow of Al2O3/water in a circular pipe under applied heat flux. A comparative analysis of flat and wavy channels, specifically under laminar flow conditions, was conducted [Citation7], presenting various corrugation profiles and contributing valuable insights to the scientific community.
Yin et al. [Citation8] simulated turbulent flow within a wavy channel using the k-epsilon turbulence model, emphasizing the phase shift of the wavy plate. Greig et al. [Citation9] investigated airflow in a complex corrugated channel under different Re, revealing that the corrugated surface induced more turbulence, positively impacting internal particle collision and interaction, thereby enhancing thermal performance. Forooghi and Hooman [Citation10] explored the impact of buoyancy on turbulent flow within corrugated pipes at various Re using FLUENT.
Ahmed et al. [Citation11] conducted a numerical investigation on nanofluid forced convection within a trapezoidal corrugated pipe, revealing a reduction in pressure drop and an increase in heat transfer with decreasing wavelength of the corrugated channel. Mohammed et al. [Citation12] used a finite volume scheme to analyze the impact of a corrugated channel's tilt angle, channel height, and various wavy heights on heat transfer rate, finding a significant increase compared to a traditional flat surface.
Khoshvaght-Aliabadi [Citation13] employed the finite volume scheme to study the fluid flow of Al2O3 within a sinusoidal wavy tube, proposing correlations for Nu and friction factor based on their findings. Ahmed et al. [Citation14] comprehensively studied the impact of SiO2 on heat transfer within a trapezoidal corrugated pipe, reporting the highest enhancement in heat transfer for the trapezoidal configuration, followed by sinusoidal, with the flat surface exhibiting the lowest enhancement rate.
Handoyo and Ichsani [Citation15] investigated three different obstacles attached to the corrugated channel of a solar air heater, comparing their impact with no obstacles present. Rashidi et al. [Citation16] numerically demonstrated entropy generation and heat transfer in turbulent fluid flow within a wavy corrugated channel, observing higher entropy generation at high Re compared to heat transfer. Feng et al. [Citation17] explored the positions of a hot wire inserted into a rectangular channel under steady-state conditions, developing correlations for Nu and friction factor.
Nguyen et al. [Citation18] studied the impact of heat transfer in a magnetic field and entropy generation within a rectangular channel featuring a triangular corrugated surface. Kanti et al. [Citation19–21] experimentally and numerically examined the fluid flow of fly ash-Cu hybrid nanofluid in a tube, considering entropy generation and pressure drop. Azadi et al. [Citation20] investigated the influence of different fin geometries attached to the bottom of a rectangular channel. In a separate investigation, Babar and Ali [Citation21] studied an airfoil pin fin. Sadegh Moghanlou et al. [Citation22] experimentally investigated a microchannel with a rectangular shape, finding better heat transfer with Al2O3 nanoparticles than TiO2. Al-Mohsen et al. [Citation23] compared circular and corrugated heat sinks in the centre of an outer insulated cylinder filled with nanofluid, concluding that the corrugated surface provided better heat transfer augmentation. Liu et al. [Citation24] employed FLUENT to investigate fluid flow in a rectangular channel with various shapes of cylindrical grooves under turbulent flow conditions, suggesting grooves with a rounded transition shape for enhanced heat transfer with minimal pressure drop.
Ionescu and Neagu [Citation25] used the COMSOL programme for finite element analysis of heat transfer within a corrugated channel of a heat exchanger. Obalalu [Citation26], Elmaboud et al. [Citation27], and Rashidi et al. [Citation28] investigated the heat transfer and pressure drop of nanofluids flowing in different structures under constant heat flux conditions. Ajeel et al. [Citation29,Citation30] conducted a numerical analysis using FLUENT and designed an experimental rig to study the impact of different corrugated channels, reporting that the trapezoidal shape exhibited the best performance for improving the heat transfer rate.
Karim et al. [Citation31] performed a numerical investigation on the thermal efficiency of a flat plate solar collector with various nanofluids, reporting an upward trend in the Nu with Re but a decline at elevated volume concentrations. In a review article, Bhuiyan et al. [Citation32] extensively examined various aspects of nanofluids, covering energy efficiency, exergy efficiency, overall efficiency, heat transfer coefficient, daily yield, power generation, entropy production, exergy loss, and temperature drop in hybrid PV/T collectors. In a separate study, Bhuiyan et al. [Citation33] investigated the retrofitting of existing coal-fired power plants in Bangladesh with carbon capture and storage technology, projecting an escalation in oxidizing elements due to enriched oxygen levels as cases transitioned from air-firing.
The existing literature primarily focuses on investigating the impact of nanofluids and channel shapes on thermohydraulic performance. However, a gap exists in comprehensive studies considering the collective effects of base fluid, nanoparticle type and size, and channel shape on both heat transfer and pressure drop. This suggests an opportunity for further research to delve into a more integrated analysis, considering multiple variables simultaneously.
In this study, we investigate the impact of diverse base fluids (ethylene glycol, glycerin, and water-based nanofluids with Al2O3, CuO, SiO2, and ZnO nanoparticles) with volume fractions from 0.01–0.04 on thermohydraulic performance within a corrugated channel.
2. Methodology
2.1. Governing equations
Figure illustrates the schematic representation of the computational domain for the current model, comprising two corrugated plates with insulated sections before and after them. The analysis is conducted in a two-dimensional framework, utilizing ANSYS FLUENT for solving and analyzing the governing equations of mass, momentum, and energy. The software is also employed for mesh generation studies and simulations. The dimensions of the physical domain are outlined in Table .
Figure 1. (a) Schematic representation of the rectangular 2D corrugated channel from its bottom Wall and (b) a wavy angle diagram.

Table 1. Specification of the domain.
The turbulence in the system is modelled using the standard form of k-ε model [Citation19,Citation34]. This model is a two-equation model in which transport equations are solved for the turbulent kinetic energy (k) and dissipation rate (ε) [Citation34]. In many applications, this model is better than other turbulence models [Citation19–21,Citation34].
Conservation of mass under steady-state conditions can be written as indicated below [Citation34];
(1)
(1) The second equation used in the present work is the conservation of momentum which can be written as inserted below:
(2)
(2) Additionally, the conservation of energy is:
(3)
(3) k-ε turbulent model equations had to be used in the present work as below;
Turbulent kinetic energy (k) equation:
(4)
(4) Turbulent kinetic energy dissipation (
equation:
(5)
(5) The empirical constants are based on experimental observation
2.2. Assumptions and boundary conditions of physical domain
No–slip conditions are considered for the walls of the corrugated shape.
Constant heat flux is applied over the entire corrugated length.
At the inlet, there is an inlet upstream velocity and inlet temperature
At the outlet, pressure is set to be equal to zero.
Fluid is a Newtonian, steady, and incompressible fluid [Citation19]
Thermophysical properties of nanofluids were constant [Citation20].
The convergence criteria for the solutions are met when the residuals reach a level lower than 10−6. The numerical solution employs the Semi-Implicit Method for Pressure-Linked Equations (SIMPLE) algorithm [Citation21], chosen for its robustness and efficiency in coupling velocity and pressure compared to other algorithm groups. Turbulent intensity (I) is determined as follows
(6)
(6)
2.3. Numerical calculations
For the corrugated pipe the heat transfer analysis and the heat transfer coefficient may be predicted theoretically from the equation inserted below [Citation35];
(7)
(7) The important parameter in the heat transfer analysis is Nu which can simply be evaluated using the equation inserted below;
(8)
(8) where L is the half distance of the channel height, k is the thermal conductivity of fluid, X is the distance from the leading edge of the corrugated plate and r is the distance from the leading edge of the corrugated plate along the corrugated surface The heat transfer in its local value can be predicted from the mathematical formula inserted below;
(9)
(9)
The upstream nanofluid velocity may be obtained using
(10)
(10) The hydraulic diameter may be elevated by
(11)
(11) The friction coefficient in its average value can be determined as below;
(12)
(12) The friction factor, fanning factor, and pressure drop may be estimated from
(13)
(13)
(14)
(14)
(15)
(15)
2.4. Thermophysical properties
The following equations are used to estimate the thermophysical properties required for this study. Tables and present the β values for various nanoparticles and thermophysical properties of the considered fluids in this work.
Table 2. Values of β for various nanoparticles [Citation38,Citation39].
Table 3. Thermophysical properties of the base fluid and the nanoparticles [Citation37–39].
Density [Citation36],
(16)
(16) Specific heat [Citation37],
(17)
(17) Thermal conductivity [Citation38,Citation39],
(18)
(18)
(19)
(19)
(20)
(20)
Effective viscosity [Citation40]:
(21)
(21)
(22)
(22)
3. Results and discussion
3.1. Validation
To assess the impact of grid size on numerical outcomes, this study employed various mesh sizes (Mesh 1–4) within the computational domain. Figure (a) depicts the meshed model of the computation domain. The accuracy of the numerical results are important and also the CPU limitations memories is another problem which leads to increase the time of numerical iterations so that, the mesh independent study and presented in Figure (b) in terms of Nu. The grid dependency analysis included mesh distributions of 24,000, 35,150, 48,000, and 88,000. Consequently, the third mesh, containing 48,000 elements, is selected for further analysis.
Figure 2. (a): Mesh generation for the computation domain. (b): Grid independence in terms of average Nusselt number.

The data obtained in the present study is compared with the findings of Naphon [Citation41], as reported in the literature. The comparison includes average corrugated plate temperature, average Nu at a wavy angle of 40 degrees, and average Nu for different wavy angles under various conditions for each mesh size. Figure (a), (b), and (c) illustrate these comparisons for further validation purposes. It is noteworthy that the maximum deviation observed across all graphs is within the acceptable limit of ±8% for the mesh elements of 48,000. However, this deviation is higher for other mesh sizes. Consequently, the mesh with 48,000 elements is selected for further simulation.
Figure 3. Comparison of (a) average corrugated plate temperature (b) average Nu for validation (c) average Nu of present work with that of Naphon [Citation41] for different wavy angle.
![Figure 3. Comparison of (a) average corrugated plate temperature (b) average Nu for validation (c) average Nu of present work with that of Naphon [Citation41] for different wavy angle.](/cms/asset/14f44334-1610-4b10-a17f-15bdc6be71cd/tusc_a_2306671_f0003_ob.jpg)
3.2. Average Nusselt number
In Figure , the variation of average Nu for nanofluids and the base fluid is depicted at different Re, with a nanoparticle diameter and volume fraction fixed at 20 nm and 0.04, respectively. The results illustrate an increase in the average Nu of nanofluids with increasing Re. Additionally, the Nu values for the considered nanofluids surpass those of water. Particularly, among the nanofluids, SiO2 and CuO exhibit the highest and lowest enhancements in Nu compared to the base fluid. Specifically, SiO2 nanofluid demonstrates a maximum Nu enhancement of 9.3%, while CuO nanofluid exhibits a minimum enhancement of 3.3% compared to water. A higher Prandtl number may be the possible reason for this enhancement [Citation42].
In Figure , the variation in the average Nu of SiO2 nanofluid (volume fraction of 0.04) with different particle diameters at various Re is illustrated. The results indicate that SiO2 nanofluids with particle diameters of 20 nm and 70 nm exhibit the highest and lowest average Nu, respectively, within the considered range of Re. This phenomenon is attributed to the fact that smaller particles possess a greater surface area, facilitating heat transfer at a higher rate [Citation43]. Furthermore, the use of smaller particle sizes, such as a diameter of 20 nm, is advantageous for better mixing with the fluid molecules, attachment, and avoidance of gravitational settling.
Figure depicts the variation in average Nu for SiO2 nanofluids with Re at different volume fractions. The results showcase an increase in average Nu with both volume fraction and Re [Citation36]. The maximum average Nu is observed for SiO2 nanofluid with a volume fraction of 0.04 at the highest Re. The possible reason for this is the addition of numerous nanoparticles to the base fluids, leading to an augmentation in the Brownian motion of the nanoparticles in the base fluid and consequently resulting in enhanced thermal conductivity of the nanofluids [Citation19–21]. Specifically, the maximum and minimum average Nu values are 200 and 133, respectively, for SiO2 nanofluid at volume fractions of 0.04 and 0.01, corresponding to the highest and lowest Re.
In Figure , the relationship between the average Nu and Re for SiO2 nanoparticles in different base fluid nanofluids is depicted. The average Nu for various base fluid nanofluids increases with Re, highlighting the positive impact of adding SiO2 nanoparticles on thermal conductivity and overall thermal performance. The results indicate that glycerin and water-based SiO2 nanofluids exhibit higher and lower average Nu, respectively. This difference is attributed to the synergistic effects of combining SiO2 nanoparticles with glycerin, leading to enhanced heat conduction. Glycerin's properties further contribute to improved stability and dispersion of the nanoparticles in the fluid, preventing agglomeration.
3.3. Pressure drop
In Figure , the changes in pressure drop for nanofluids at a concentration of 0.04 and a particle diameter of 20 nm as a function of Re are illustrated. The results reveal a consistent increase in pressure drop with increasing Re [Citation20]. Across all nanofluids, there is a noticeable escalation in pressure drop compared to the base fluid. Notably, the SiO2 nanofluid stands out, displaying a maximum pressure drop of 115 Pa at the specified volume concentration, surpassing that of water at a constant Re. This heightened pressure drop in the SiO2 nanofluid can be attributed to its greater viscosity relative to all other fluids [Citation21].
In Figure , the variation in pressure drop for SiO2 nanofluid (volume fraction of 0.04) with different particle diameters at various Re is illustrated. The findings reveal that SiO2 nanofluids with particle diameters of 20 nm and 70 nm display the maximum and minimum pressure drop, respectively, within the considered range of Re. This difference is attributed to the fact that nanofluids with smaller particle sizes exhibit greater viscosity compared to larger particles [Citation43]. The smaller particles in the nanofluid resist the flow, impeding the movement of the fluid and leading to increased shear force, which contributes to the observed increase in pressure drop.
In Figure , the variations in pressure drop for SiO2 nanofluids at different volume fractions and Re are illustrated. Notably, the pressure drop of SiO2 nanofluid at all volume fractions is higher than that of water [Citation20,Citation36]. The maximum and minimum pressure drop values, 115 and 9 Pa, respectively, are observed at volume fractions of 0.04 and 0.01, corresponding to the highest and lowest Re. This phenomenon can be attributed to the increased viscosity of nanofluids resulting from the addition of a higher number of nanoparticles [Citation36]. However, it's crucial to note that an excessively high viscosity is undesirable. Therefore, when considering nanofluids for any application, an optimal volume concentration should be carefully chosen to balance enhanced properties with practical usability.
In Figure , the correlation between pressure drop and Re for SiO2 nanoparticles in various base fluids is illustrated. The pressure drop in different base fluid nanofluids rises with increasing Re [Citation21]. The findings reveal that glycerin-based SiO2 nanofluids display higher pressure drops compared to water-based ones. This discrepancy can be attributed to the inherent higher viscosity of glycerin in comparison to water, impacting the convective heat transfer characteristics of the nanofluid.
3.3. Impact of geometry
In Figure , the variation in local Nu with the corrugated wall at different Re for SiO2 nanofluid (volume fraction of 0.04) is depicted. The local Nu increases with the corrugated wall length. This improvement can be ascribed to several factors, including enhanced turbulence, increased surface area, and secondary flow effects. The irregular surface of corrugated channels disrupts the boundary layer, fostering convective heat transfer. Longer corrugated channels provide an extended path for fluid to exchange heat with the walls, leading to enhanced convective heat transfer and higher local Nu [Citation35]. The maximum local Nu of 470 is observed at Re of 20000 for the SiO2 nanofluid.
The influence of Re on fluid flow streamlines and isotherms for Al2O3 nanofluid (volume fraction of 0.04) with a heat flux of 1.1 kW is presented in Figure (a) and (b), respectively. It is observed that with an increase in Re, both fluid flow and temperature distribution (isotherms) exhibit noticeable changes. The elevation in Re results in the development of more pronounced eddies between the triangular ribs, signifying an augmentation in the turbulent characteristics of the flow. Furthermore, the upward trend in Re corresponds to a temperature increase, implying that higher Re plays a role in improving heat transfer within the system.
Figure 13. (a) streamlines (b) temperature contour for different Re at volume fraction 4% of Al2O3 nanofluid and heat flux of 1.1 kW.

Figure (a) compares the average Nu between a wavy channel (angle 20o) and a straight channel at various Re. The results indicate that the wavy channel consistently exhibits superior heat transfer compared to the straight channel across different Re. The possible reason for the enhancement in the case of the wavy angle is improved turbulence, increased surface area, secondary flow, and boundary layer disruption. Furthermore, Figure (b) illustrates the impact of the wavy angle on average Nu with Re. The average Nu improves with an increase in the wavy angle. This improvement is attributed to the higher fluid flow intensity associated with the increasing wavy angle.
4. Conclusions
The numerical investigation in this study focused on the forced convection of nanofluids based on different base fluids (EG, Glycerin, and water) (containing Al2O3, CuO, SiO2, and ZnO nanoparticles) flowing in a corrugated triangular channel under turbulent conditions. The key conclusions can be summarized as follows:
The average Nusselt number increases as the particle size decreases. Specifically, SiO2 nanofluid with a particle size of 20 nm exhibits the maximum average Nusselt number of 200, while that with a particle size of 70 nm shows the minimum value of 132.
The maximum average Nusselt number for SiO2 nanofluid at a volume fraction of 0.04 is 5.8% higher than that of CuO nanofluid under similar conditions.
SiO2 nanofluid (volume fraction of 0.04) experiences a maximum pressure drop enhancement of 187.5% at the highest Reynolds number compared to water.
The highest and lowest pressure drop values of 115 and 6 Pa are observed for SiO2 nanofluid (volume fraction of 0.04) with particle sizes of 20 nm and 70 nm, respectively, at the highest and lowest Reynolds numbers.
SiO2-glycerin-based nanofluid shows a greater pressure drop compared to EG and water-based nanofluids.
The local Nusselt number ranges from 45 to 470 for SiO2 nanofluid (volume fraction of 0.04) over Reynolds numbers of 8000 to 20000.
The average Nusselt number for a wavy angle of 60o is 125% higher compared to that of a 20o wavy angle.
This study is limited to numerical analysis using mono nanofluids within the turbulent range. As a promising direction for future research, the insights gained from this work could provide a basis for experimental investigations. Furthermore, researchers may consider exploring the use of hybrid nanofluids to examine the fluid flow and heat transfer characteristics in a corrugated wavy channel, covering both laminar and turbulent ranges.
Authors’ contribution
“All authors contributed equally in the preparation of this manuscript.”
Acknowledgments
The authors extend their appreciation to the Deanship of Scientific Research at King Khalid University for funding this work through large group research project under grant number RGP.2/7/44.
Disclosure statement
No potential conflict of interest was reported by the author(s).
Additional information
Funding
References
- Esmaeili M, Sadeghy K, Moghaddami M. Heat transfer enhancement of wavy channels using Al2O3 nanoparticles. J Enhanced Heat Transfer. 2010;17(2):139–151.
- Elshafei EAM, Awad MM, El-Negiry E, et al. Heat transfer and pressure drop in corrugated channels. Energy. 2010;35(1):101–110.
- Ahmed MA, Shuaib NH, Yusoff MZ, et al. Numerical investigations of flow and heat transfer enhancement in a corrugated channel using nanofluid. Int Commun Heat Mass Transfer. 2011;38(10):1368–1375.
- El-Sebaii AA, Aboul-Enein S, Ramadan MRI, et al. Investigation of thermal performance of-double pass-flat and v-corrugated plate solar air heaters. Energy. 2011;36(2):1076–1086.
- Rostamani M, Hosseinizadeh SF, Gorji M, et al. Numerical study of turbulent forced convection flow of nanofluids in a long horizontal duct considering variable properties. Int Commun Heat Mass Transfer. 2010;37(10):1426–1431.
- Bianco V, Manca O, Nardini S. Numerical investigation on nanofluids turbulent convection heat transfer inside a circular tube. Int J Therm Sci. 2011;50(3):341–349.
- Fabbri G. Heat transfer optimization in corrugated wall channels. Int J Heat Mass Transfer. 2000;43(23):4299–4310.
- Yin J, Yang G, Li Y. The effects of wavy plate phase shift on flow and heat transfer characteristics in corrugated channel. Energy Procedia. 2012;14:1566–1573.
- Greig D, Siddiqui K, Karava P. An experimental investigation of the flow structure over a corrugated waveform in a transpired air collector. Int J Heat Fluid Flow. 2012;38:133–144.
- Forooghi P, Hooman K. Effect of buoyancy on turbulent convection heat transfer in corrugated channels–a numerical study. Int J Heat Mass Transfer. 2013;64:850–862.
- Ahmed M, Yusoff MZ, Shuaib N. Effects of geometrical parameters on the flow and heat transfer characteristics in trapezoidal-corrugated channel using nanofluid. Int Commun Heat Mass Transfer. 2013;42:69–74.
- Mohammed H, Abed AM, Wahid M. The effects of geometrical parameters of a corrugated channel with in out-of-phase arrangement. Int Commun Heat Mass Transfer. 2013;40:47–57.
- Khoshvaght-Aliabadi M. Influence of different design parameters and Al2O3-water nanofluid flow on heat transfer and flow characteristics of sinusoidal-corrugated channels. Energy Convers Manage. 2014;88:96–105.
- Ahmed MA, Yusoff MZ, Ng KC, et al. Numerical and experimental investigations on the heat transfer enhancement in corrugated channels using SiO2–water nanofluid. Case Stud Therm Eng. 2015;6:77–92.
- Handoyo EA, Ichsani D. Numerical studies on the effect of delta-shaped obstacles’ spacing on the heat transfer and pressure drop in v-corrugated channel of solar air heater. Sol Energy. 2016;131:47–60.
- Rashidi S, Akbarzadeh M, Masoodi R, et al. Thermal-hydraulic and entropy generation analysis for turbulent flow inside a corrugated channel. Int J Heat Mass Transfer. 2017;109:812–823.
- Feng Z, Luo X, Guo F, et al. Numerical investigation on laminar flow and heat transfer in rectangular microchannel heat sink with wire coil inserts. Appl Therm Eng. 2017;116:597–609.
- Nguyen Q, Bahrami D, Kalbasi R, et al. Nanofluid flow through microchannel with a triangular corrugated wall: heat transfer enhancement against entropy generation intensification. Math Methods Appl Sci. 2020: 1–14.
- Kanti PK, Sharma KV, Minea AA, et al. Experimental and computational determination of heat transfer, entropy generation and pressure drop under turbulent flow in a tube with fly ash-Cu hybrid nanofluid. Int J Therm Sci. 2021;167:107016.
- Kanti P, Sharma K, Said Z, et al. Entropy generation and friction factor analysis of fly ash nanofluids flowing in a horizontal tube: experimental and numerical study. Int J Therm Sci. 2021;166:106972, doi:10.1016/j.ijthermalsci.2021.106972
- Kanti P, Sharma KV, Said Z, et al. Numerical study on the thermo-hydraulic performance analysis of fly ash nanofluid. J Therm Anal Calorim. 2022;147:2101–2113. doi:10.1007/s10973-020-10533-0
- Azadi M, Hosseinirad E, Hormozi F, et al. Second law analysis for nanofluid flow in mini-channel heat sink with finned surface: a study on fin geometries. J Therm Anal Calorim. 2020;140(4):1883–1895.
- Babar H, Ali HM. Airfoil shaped pin-fin heat sink: potential evaluation of ferric oxide and titania nanofluids. Energy Convers Manage. 2019;202:112194.
- Farhad SM, Noorzadeh S, Ataei M, et al. Experimental investigation of heat transfer and pressure drop in a minichannel heat sink using Al2O3 and TiO2–water nanofluids. J Braz Soc Mech Sci Eng. 2020;42(6):1–11.
- Al-Mohsen SAA, Abed IM, Ali FH. A numerical comparison of circular and corrugation heat sink for laminar CuO–water nano-fluid flow and heat transfer enhancement. Appl Nanosci. 2023;13:2739–2766.
- Liu J, Xie G, Simon TW. Turbulent flow and heat transfer enhancement in rectangular channels with novel cylindrical grooves. Int J Heat Mass Transfer. 2015;81:563–577.
- Ionescu V, Neagu A-A. Numerical modelling of fluid flow and heat transfer in a corrugated channel for heat exchanger applications. Procedia Manuf. 2018;22:634–641.
- Obalalu AM, Wahaab FA, Adebayo LL. Heat transfer in an unsteady vertical porous channel with injection/suction in the presence of heat generation. J Taibah Univ Sci. 2020;14(1):541–548. doi:10.1080/16583655.2020.1748844
- Abd Elmaboud Y. Two-layered electroosmotic flow through a vertical microchannel with fractional Cattaneo heat flux. J Taibah Univ Sci. 2021;15(1):1038–1053. doi:10.1080/16583655.2021.2016162
- Rashidi MM, Akolade MT, Awad MM, et al. Second law analysis of magnetized Casson nanofluid flow in squeezing geometry with porous medium and thermophysical influence. J Taibah Univ Sci. 2021;15(1):1013–1026. doi:10.1080/16583655.2021.2014691
- Dagdevir T, Keklikcioglu O, Ozceyhan V. Thermo-hydraulic performance analyses of water based CuO-SiO2 hybrid nanofluid flow in a horizontal straight tube. Res Eng Struct Mat. 2018;4(2):91–102.
- Kanti P, Sharma KV, Sekhar YR. Influence of particle size on thermal conductivity and dynamic viscosity of water-based Indian coal fly ash nanofluid. Heat Transfer. 2021;51(1):413–433. doi:10.1002/htj.22313
- Nuhash MM, Alam MI, Zihad A, et al. Enhancing energy harvesting performance of a flat plate solar collector through integrated carbon-based and metal-based nanofluids. Results Eng. 2023;19:101276, ISSN 2590-1230, doi:10.1016/j.rineng.2023.101276
- Ajeel RK, Salim WI, Sopian K, et al. Turbulent convective heat transfer of silica oxide nanofluid through corrugated channels: an experimental and numerical study. Int J Heat Mass Transfer. 2019;145:118806.
- Ajeel RK, Salim W-I, Hasnan K. Thermal performance comparison of various corrugated channels using nanofluid: numerical study. Alexandria Eng J. 2019;58(1):75–87.
- Versteeg HK, Malalasekera W. An introduction to computational fluid dynamics: the finite volume method. Pearson education Limited; 2007.
- Elbadawy I, Alali F, Derakhshandeh JF, et al. Effect of Al2O3, SiO2, and ZnO nanoparticle concentrations mixed with EG–water on the heat transfer characteristics through a microchannel. Processes. 2023;11(7):2015, doi:10.3390/pr11072015.
- H B M, Kanti PK, Prakash SB, et al. Investigation of entropy generation and thermohydraulic characteristics of Al2O3–CuO hybrid nanofluid flow in a pipe at different inlet fluid temperatures. Int J Therm Sci. 2023;193:108541, doi:10.1016/j.ijthermalsci.2023.108541
- Marulasiddeshi HB, Kanti PK, Jamei M, et al. Experimental study on the thermal properties of Al2O3-CuO/water hybrid nanofluids: Development of an artificial intelligence model. Int J Energy Res. 2022;46(15):21066–21083. doi:10.1002/er.8739
- Vajjha RS, Das DK. Experimental determination of thermal conductivity of three nanofluids and development of new correlations. Int J Heat Mass Transfer. 2009;52(21-22):4675–4682.
- Vajjha RS, Das DK, Kulkarni DP. Development of new correlations for convective heat transfer and friction factor in turbulent regime for nanofluids. Int J Heat Mass Transfer. 2010;53(21-22):4607–4618.
- Corcione M. Heat transfer features of buoyancy-driven nanofluids inside rectangular enclosures differentially heated at the sidewalls. Int J Therm Sci. 2010;49(9):1536–1546.
- Naphon P. Heat transfer characteristics and pressure drop in channel with V corrugated upper and lower plates. Energy Convers Manage. 2007;48(5):1516–1524.