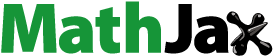
Abstract
Thermo hydraulic analysis of nano-fluid flow phenomena through backward-facing channel has currently gained attention of the researchers, and is applied in a variety of engineering applications. Hydro-thermal properties of water/FMWCNT nano-fluid flow in a backward-facing channel embedded with an obstacle under uniform heat flux have been studied numerically with the variations in the values of Reynolds numbers weight percentage of carbon nano-particle
and differently shaped obstacles. The governing equations (continuity, x-momentum, y-momentum, and energy) have been solved using the finite volume approach, and fluent software has been used to visualize the simulation results. Impact of various forms of obstacle (plane, elliptical, and triangular), the ratio of obstacle height to width (A/B), the ratio of obstacle pitch to width
,
, and the values of
on the different rheological behaviour of nano-fluid flow have been investigated. For all the forms of obstacle configuration, the distributions of velocity streamlines, temperature contours, absolute pressure drop
, average friction factor
, local Nusselt number
, skin friction coefficients
, average Nusselt number (
), and pumping power (
) have been demonstrated for several values of
and
, and
and
. For several values of
and
, generations of frictional entropy
, thermal entropy
, and Bejan number
have been explored. It seems that the presence of obstacle, and the increase in the values of
,
causes the rise of the values of
, and
than the case of smooth channel. Moreover, at
and
, it has been found that the
increase in
becomes
in the case of backward-facing channel with plane obstacle (A/B = 1) as compared to the case of smooth channel. Furthermore, at a constant value of
it has been found that the generation of
decreases but the generation of
entropy increases with the rise in the values of
.
1. Introduction
Fossil fuels and the growing consumption of energy are needed for survival in the modern world. In several countries, this kind of consumption depletes natural resources, the emission of air pollutants, and the creation of greenhouse gases [Citation1]. Efficient processes and industrial HT technologies [Citation2–4] are now employed to minimize environmental deterioration, and advanced technology, in many industries, is adopted for heat exchangers to shrink in size and eventually, to boost energy and fuel efficiency [Citation5–7]. The development of compact heating systems has emerged as an important and difficult goal for enhancing efficiency across a variety of industries in recent years [Citation8–10]. One of the best solutions is use of nano-fluids instead of water. The use of metallic and non-metallic particles in the base fluid is regarded as nano-fluid, and the use of this is one of the most innovative and amazing approaches for improving HT. Furthermore, nano-fluids enable the development of a new generation of industrial applications with better potential and performance. Many policies, including the use of extended surfaces, rough surfaces, corrugated channels, and various types of obstacles and grooves, have been designed to boost the rate of thermal exchange [Citation11–13]. There is a need to boost HT capabilities in existing systems, and nano-fluids composed of metallic and non-metallic particles suspended in a base fluid are being used to do this. Furthermore, the use of nano-fluids has started an age of innovation in the industry. Various authors [Citation1–13] have enhanced the thermal efficiency of flow through channels by using nano-fluids. The inclusion of obstacles on the surfaces of HT systems is a typical approach to saving cost. In comparison to conventional HT methods, nano-fluids provide greater HT at a lower cost or with no reduction in absolute pressure.
The primary machinery components that rely on the simultaneous transmission of mass and heat are heat exchangers. Many manufacturing sectors have been trying to create new and smaller-sized designs that are more effective for transferring heat. A heat exchanger’s thermo-hydrodynamic performances can be enhanced by altering the model’s geometrical characteristics or the physical characteristics of the heating and cooling fluids. The geometry for enhancement indicates that the use of extended surfaces (fins) is an effective approach. Several research projects [Citation1–13] suggest that the use of solid inserts in geometry (like baffles, deflectors, etc.) causes the enhancement of the rate of HT in heat exchangers. The inclusion of these solid blocks causes the flow to become more turbulent and shift from a laminar to a turbulent regime by generating singularities in the flow as well as increasing surface contact. This alteration in flow regime results in a decrease in thermal resistance thickness, which raises the rate of HT. Inclusion of obstacles in the walls of a smooth channel with in-line [Citation14] or staggered manners [Citation15] is one of the most effective passive ways of increasing the convective HT rate, as the obstruction forces a vortex downstream and aids in disrupting the hydrodynamic and thermal boundary layers [Citation16]. Fluid particles are transferred from the hot surface quickly as a result of the vortex’s spinning motion within the flow stream [Citation17]. The literature on this topic is widely apparent because this method of improving thermal transfer is frequently used in various engineering and industrial applications, such as electronic packages [Citation16], solar energy collectors [Citation17], gas turbine cooling systems [Citation18], and shell-and-tube heat exchangers with segmental baffles [Citation19].
A well-known issue is sudden expansion [Citation20], where the flow expands abruptly. Convection flow and HTs through an abruptly expanded channel are commonly seen in various engineering applications and thermal systems, such as cooling tubes of turbine blades, combustors, and heat exchangers. A substantial deal of mixing of high and low-energy fluids occurs in the flow reattachment zone, which has a significant impact on the flow and HT characteristics of these devices. The reattaching flow zone and the reverse flow regions particularly exhibit significant variations in momentum and HT’s. For instance, the immediate vicinity of the reattaching flow region experiences the lowest wall shear stress and the highest HT rate, but the flow at the corner region (where the abrupt change in the flow geometry begins) experiences the lowest HT rate [Citation21]. Backward-facing step flow is an important separation flow model that encompasses free shear flow detachment, vortex evolution, and re-attachment. In the year of 2008, Abu Nada [Citation22] numerically studied the impact of a nano-fluid on fluid flow and HT through a backward-facing channel. He has shown that the value of is enhanced with the enhancement in
. Al-aswadi et al. [Citation23] have studied the flow phenomena of nano-fluid through a backward-facing channel from numerical standpoint. They have found that an increase in the length of recirculation region with the increase in particle density and
. Numerically, Mohammed et al. [Citation24] studied the impact of various types of nano-fluids on fluid flow and HT across a horizontal backward-facing step channel. They found that the profiles of
decrease as the value of
increases. With the variation of
, Kherbeet et al. [Citation25] experimentally and numerically studied the water-Al2O2, and water-SiO2 nanofluid flow phenomena through a backward-facing channel. In a backward-facing channel, Amiri et al., [Citation26] examined the various characteristics of thermo-hydraulic water-ethylene glycol nano-fluid flow phenomena. They noted that the rate of HT enhances with the increment of the value of
. In the year of 2019, Nath et al., [Citation27] numerically performed the hydrothermal flow phenomena of water-Cu nano-fluid flow through a backward-facing channel. Their findings showed that when the
reaches 0–2, the
rises up to 77%. Sadeq et al. [Citation28] investigated the modelling of turbulent heat transmission over a micro scale backward facing channel. They discovered that the mixture of water-ethylene glycol enhanced the rate of HT than the base fluid (water). Following some recent work of renowned researchers, we have demonstrated in Table , which becomes more effective in developing the current field / new era of research.
Table 1. Some recent works on backward-facing channel and their obtained results.
After going through the above discussions, it is obvious that nano-fluid plays an important role in the cooling industry for studying the different hydrothermal flow characteristics. Notwithstanding the considerable potential of nano-fluid flow through backward facing channel with various forms of obstacle, not enough research has been done till now. The literature review reveals that the majority of the works have been completed without considering the influence of the presence of obstacle, various shapes of obstacle configuration (plane, elliptical, and triangular), ratio of obstacle height to width, and (FMWCNT nano-fluid flow) on the distributions of the profiles of velocity streamlines, temperature contours,
,
,
,
,
, and
. This present work is the extension or updated form of Alrashed et al. [Citation29], where they have studied the water-FMWCNTs [Citation40–45] nano-fluid flow characteristics in a backward facing channel in absence of obstacle, and without considering the analysis of entropy generation. It seems to us that the unexplored areas of research are still there, as the above mentioned investigations are limited to the consideration of nano-fluid flow in a backward facing channel, which provides us enough confidence to implement the current idea. With all this in mind, here we have numerically studied the various thermo-hydraulic phenomena, and entropy generation of laminar water-FMWCNT nano-fluid flow through a backward facing channel embedded with various forms of obstacle (plane, elliptical, and triangular). The aforementioned major points have not been covered in the previously released publications.
To study the influence of
on various characteristics of thermo-entropy analysis of water-FMWCNT nano-fluid flow phenomena (velocity streamlines, temperature contours,
,
,
,
,
,
,
,
,
and
).
To investigate the impact of
on various characteristics of thermo-entropy analysis of water-FMWCNT nano-fluid flow phenomena (velocity streamlines, temperature contours,
,
,
,
,
,
,
,
,
and
).
To examine the influence of various obstacle shape configuration (plane, elliptical, and triangular) on various characteristics of thermo-hydraulic analysis of water-FMWCNT nano-fluid flow phenomena (velocity streamlines, temperature contours,
,
,
,
,
, and
).
To investigate the influence of the ratio of obstacle (plane shape) height to width (A/B = 1, 2), ratio of obstacle (plane shape) pitch to width (
= 2) on various characteristics of thermo-hydraulic analysis of water-FMWCNT nano-fluid flow phenomena (velocity streamlines, temperature contours,
,
,
,
,
, and
).
A comparative study has been performed to determine which obstacle configuration provides a greater rate of HT by analyzing the Nusselt number profile.
A comparative investigation has been demonstrated to reveal the % increase of HT rate in case of different forms of obstacle in a backward-facing channel vs. smooth channel.
The aforementioned reasons encourage us to accomplish the current work. The findings in this article are particularly beneficial to many technical communities, including those who work in electronics, power stations, development of aerospace and vehicles, and turbo-machinery. Moreover, the current study’s findings will be extremely beneficial in a variety of engineering applications, including glass blowing, fibre spinning, and continuous metal casting, especially in a variety of manufacturing processes like transpiration cooling, fabric cleaning, PV collector, and laser pulse heating. Overall, the in nano-fluid affects the efficiency of PV collectors, which can be seen in the result section that how
obstacles shape, and
influence the hydrothermal phenomena and entropy generation. The authors claim that no previous integrated effort of this nature has been attempted.
2. Computational geometry
Having academic and practical significance, the backward-facing step flow is a common example of separation flow. Fluid flows via expansion configurations are also connected to several engineering applications, such as., food processing, extrusion procedures, mold filling, ceramics, air condition networks, combustor, condenser, flow passing through the engine, and pharmaceuticals. Since the flow via an abrupt enlargement has a layout with one of the most basic geometries, it has been considered a benchmark to facilitate more numerical and experimental investigations on similar flows. This geometric simplicity does not necessarily mean that the flow motion is easy to understand. Moreover, a large number of scientists are interested in elaborate devices that have reattachment, flow separation, and many re-circulating zones of fluid flow. The application of abrupt expansion configurations is growing rapidly. In aerodynamics, separation flows occur, when the fluid passes behind the vehicle, past the spoilers, and airfoil. The existence of an obstruction in the flow geometry acts as a mixture blade. Well mixing of the flow causes an increase in the rate of HT. Because of its importance in various real-life engineering applications, we have taken the backward-facing channel with obstacle for more evolution of research in case nano-fluid flow (water-FMWCNT). The Figure (a-d) present the backward-facing expansion channel (a) with no obstacle and in presence of (b) plane, (c) triangular and (d) elliptical obstacles. The channel consists of four sections, viz., inlet section, through which the flow enters into the system, lower wall, and upper wall and outlet section. A vortex generator in the form of an obstacle has been affixed to the channel wall at a distance of B1 from the channel’s upstream end. The goal of this work is to model the flow of nano-fluids and HT in two-dimensional channel with various forms of obstacle. Through the left side of the channel (inlet), flow enters with a uniform velocity at temperature,. The lower wall is kept under a constant heat flux
and the other walls remain insulated, and the pressure outlet boundary condition has been prescribed at the outlet section. The dimensions of the geometry have been taken from the study of Alrashed et al. [Citation29], as shown in Table . As per the experimental studies of Amrollahi et al. [Citation40], Safaei et al. [Citation41], and numerical studies of Alrashed et al. [Citation29], Nikkah et al. [Citation42], we have taken the thermo-physical characteristics of water-FMWCNT nano-fluid nano-particles, as shown in Table . Amrollahi et al. [Citation40] experimentally measured the heat capacity of nano-fluids, and discovered that it is equal to the heat capacity of water [Citation29,Citation40–42]. They used piecewise linear regression on the experimental data to determine the viscosity and thermal conductivity in the solution domain.
Table 2. Geometry dimensions.
Table 3. Experimentally measured [Citation40] thermo-physical characteristics of FMWCNT nano-fluids at T = 303K [Citation40–42].
The followings assumptions have been made
Flow is assumed to be steady, incompressible, and two-dimensional.
The nature of fluid is assumed as laminar and Newtonian.
The uniform axial velocity with
has been maintained at the inlet section.
The body forces, radiation of HT and viscous dissipation are neglected.
3. Mathematical formulations
To analyze the nano-fluid flow phenomena, we consider the below equations, as per studies of [Citation29,Citation42,Citation43].
Equation of continuity:
(1)
(1) Momentum equation of x:
(2)
(2) Momentum equation of y:
(3)
(3) Energy equation:
(4)
(4) with
= (u, v),
The considered parameters have been formalized as per the study of [Citation29,Citation40,Citation44], are as follows:
(5)
(5)
(6)
(6)
(7)
(7)
(8)
(8)
(9)
(9)
(10)
(10)
(11)
(11)
(12)
(12)
3.1. Boundary conditions
The computational domain has been divided into three sections, such as the inlet, outlet, lower and upper walls. The boundary conditions have been taken from the studies of [Citation29] are as follows:
Inlet section: Uniform velocity has been prescribed.
Outlet section: Pressure outlet boundary condition has been used. The static pressure at flow outputs is defined by pressure outlet boundary conditions. When backflow happens during iteration, using a pressure outlet boundary condition rather than an outflow condition frequently leads to a faster rate of convergence.
Lower and upper walls: No slip
and no penetration
boundary conditions have been applied at the walls. The lower wall is kept under a constant heat flux (25000 w/
), and the other walls remain insulated.
3.2. Properties of nano-fluid
As per the study of Ghasemi et al. [Citation45], following mean empirical correlation has been employed to calculate the effective thermal conductivity ().
(13)
(13) Static thermal conductivity [Citation46–48]:
(14)
(14) Brownian thermal conductivity [Citation49]:
(15)
(15) In equation (15), the expressions of modelling function (
), modelling constants
, and Boltzmann constant (
) have been taken from the experimental studies of [Citation49,Citation50]. Brinkman [Citation51] correlation (equation 16) has been used for calculating the
(16)
(16) As per the study of [Citation52], the expression of
, and
have been taken, as follows:
(17)
(17)
(18)
(18)
3.3. Entropy generation
Following the study of [Citation53,Citation54], the entropy generation of nano-fluid flow through the considered geometry, written in terms of and
(19)
(19)
(20)
(20) For a pipe having a square cross section, Bianco et al. [Citation55] found:
(21)
(21)
(22)
(22) For a tube encountering a
, the first law of the energy balance is applied, and
is determined as per the study of [Citation55]:
(23)
(23)
3.4. Computational procedures and grid study
The mathematical equations have been solved using FVM [Citation1,Citation3,Citation4,Citation13,Citation29,Citation56]. Numerical simulations are effective tools for anticipating performance in the industry and laboratory. For solving the computational domain, FVM and the SIMPLEC algorithm [Citation1,Citation3,Citation4,Citation13,Citation29,Citation56] have been employed and for pressure-velocity coupling and discretization the governing equations, the 2nd order upwind scheme [Citation1,Citation3,Citation4,Citation13,Citation29,Citation56] has been used. The FVM is used to formulate the unstructured mesh and to solve the governing equations, which reduces a PDE to a set of algebraic equations. The divergence theorem has been applied to convert triple integrals into double integrals. This technique generates a mesh similar to the finite element method, which is made up of partitions of the domain. Volume refers to the mesh element. We obtain a balanced equation by integrating the PDE over each volume. After that, a set of discrete unknowns is used to discretize the system of balanced equations. The primitive variables (pressure and velocity) of the governing equations are created using the SIMPLEC technique, while convective terms are created using the second-order upwind approach. A residual of [Citation29,Citation57,Citation58] has been taken to ensure accurate iteration numbers and control numerical inaccuracies.
Figure (a-d) present the geometry of mesh configurations in the case of (a) a smooth backward-facing channel, and the same in the presence of (b) the plane obstacle with B/A = 2, (c) the triangular obstacle, and (d) the elliptical obstacle. Figure (a) shows the uniform rectangular mesh arrangement of the channel. Figure (b-d) shows the mesh configurations for the presence of various obstacle. Due to the presence of obstacle, we have observed the inconsistency in grid generation. Here, the grid has been chosen as non-uniform and highly dense near the baffles to obtain the high velocity gradient, pressure, and temperature. Prior to the study, all the geometrical configurations under consideration were subjected to a grid sensitivity test. At five different grid sizes are considered in the case of smooth backward-facing channel (Table ). The grid density has remained higher in the vicinity of the heated wall and obstacles to capture the variations in the flow and temperature fields within the hydraulic and thermal boundary layers. The objective of the grid independence test [Figure (a, b)] is to determine the optimal grid for which the output quality is obtained at the lowest.
Figure 2. Mesh geometry for (a) smooth channel, presence of (b) plane obstacle with B/A = 2, (c) triangular obstacle and (d) elliptical obstacle.

Table 4. Grid distribution in case of backward-facing channel without obstacle at .
The working procedure of SIMPLEC algorithm has been described in flowchart 1:
possible computational cost. The selected grid number in this case has been assessed between 5,000 and 75,000. The values of (Figure a) and
(Figure b) are calculated at the lower wall of the channel for various numbers of meshes to ensure that the effects of hydrothermal phenomena depend on the grid. After 29,995 no of grids, it has been found that both the profiles of F and
become linear. Therefore, we have selected 29,995 no grids for the case of smooth channel. In addition, 32350, 30895, and 29524 numbers of grids are selected for other considered cases (Figure b–d). FLUENT, commercial CFD software has been used to simulate the temperature and fluid flow fields.
3.5. Validation of code
Before going to present the important outcomes of this work, we need to make sure that the numerical code used in the analysis is accurate. To evaluate the accuracy of the numerical solution technique and to control the degree of error in the numerical scheme, the current study has been validated by numerical work [Citation29] and experimental work [Citation59]. Using the SIMPLEC algorithm and the FVM, Alrashed et al. [Citation29] numerically studied the water-FMWCNTs nano-fluid flow characteristics in a backward-facing channel in absence of obstacle and without considering the entropy generation. They have revealed that the increase in or
causes an increase in the values of
and
, as shown in Figure (a, b). We have taken the same geometric configuration for validation purposes. By comparing the profiles of
and
for various values of
, we have found a strong agreement between results of the work [Citation29] and the present work, as shown in Figure (a, b). In addition, for more justification, we have validated our results with the experimental study of [Citation59] by considering the plot of
Abedalh et al. [Citation59] experimentally investigated the mixed convection of water-Al2O3-TiO2 hybrid nano-fluid flow through a smooth backward-facing channel. At
they have stated that an increase in the value of
causes an enhancement in
, as shown in the Figure (c). Moreover, we have studied the % errors between the results of present work and the works [Citation29,Citation59] as shown in Tables . From the Tables , it has been found that % error values become
in all the considered cases for validation (Figure a–c). We have adequate trust to continue with the current study because the Figure (a)–(c) and Tables demonstrate the strong agreement between the results of our work with those of the results of [Citation29,Citation59].
Table 5. % error analysis of vs.
for
(Figure a).
Table 6. % error analysis of vs.
for
(Figure b).
Table 7. % error analysis of vs.
for
(Figure c).
4. Results and discussions
Here, we have investigated the impact of using different operating circumstances, such as shape of the obstacles (plane, triangular and elliptical), and
on various rheological phenomena (velocity vectors, velocity streamlines, temperature contours,
,
,
,
,
,
,
,
, and
) of water-FMWCNT nano-fluids flow through a backward-facing channel.
4.1. Impact of obstacle, 
, and 
on hydro-thermal flow phenomena
In the case of smooth channel, Figure (a–d) show the distribution of velocity streamlines at several values of , and
Thermal conductivity rises as nano-particle concentration increases, resulting in enhancement in the thermal transfer of the fluid within the channel. It has been observed that an increase in the values of
, and
leads to an improvement in velocity values and the length of recirculation zone. The zone of recirculation has been found behind the step expansion. At Re = 1 [Figure (a-f)] and Re = 150 [Figure (a-f)], the profiles of velocity streamline have been presented for different considered configurations, viz., (a) smooth channel, in presence of plane obstacle with (b) A/B = 1, (c) A/B = 2, (d)
, (e) triangular obstacle and (f) elliptical obstacle. As the inflow velocity rises, we observe that non-viscous core penetration has a greater impact in the region close to the expansion section as the pressure decreases there. One or two distinct flow regions form next to the backward-facing step based on the value of
and the thickness of the momentum boundary layer at the step. It has been seen that one recirculation zone is formed at the upstream of the step and one recirculation zone develops at the downstream of the step. As the flow passes through the channel expansion, some vortices are found to form at the low-pressure regions for the increase in the inlet velocity. The vortexes are strengthened and moves toward the channel outlet as the inlet velocity increases. From the Figure and 7, it is noted that the weak zone formed in the step corner is consistent with the flow. The weak zone is compressed due to the presence of obstacle in the backward facing step and two other weak zones are formed at both sides of the base of the obstacle for which the length of the weak zone adjacent to the step corner decreases. However, the inertial effect surrounding the obstacle becomes dominant as the flow rate decreases at the corner wall of the channel, which causes a decrease in the reattachment length of the circulation zone. From the Figure and 7, it has been observed that a recirculation zone appears behind the step as
increases, and the flow splits at the step’s edge. As the
rises, the recirculation zone’s size initially reduces in between the expansion zone and obstacle, but after crossing the obstacle the length of the recirculation zone increases. It has been found that the profile of velocity streamlines becomes more pronounced in the case of plane obstacle than in the other considered cases. It has also been found that the trend of re-separation of the main flow stream from the top wall of the channel becomes high for the plane obstacle for having a large cross-section (A/B = 1), as it has the steepest contraction and expansion among all the obstacles. In this case, the recirculation zone in the downstream becomes prominent.
In the Figures and , we have demonstrated the influence of , and obstacle shape on the hydro phenomena by presenting the temperature contours. From Figures and , we have seen that the decrease in the gradients of temperature at the lower wall is induced by the increase in the velocity. As the momentum of cooling fluid is strengthened at a higher value of
, temperature decreases around the expansion part. Amount of the heat transfer depends on the velocity field and temperature field. In addition, it has been found that as
increases, the rate of heat transmission increases and the thickness of the thermal boundary layer in hot regions considerably decrease. It has been observed that the presence of a weak zone adversely affects the convection of heat transfer. Moreover, it has been found that the use of a plane obstacle with A/B = 1, in the backward facing channel reduces temperature highly. Furthermore, it has been deduced that the isotherms in the downstream region of the channel with plane obstacle for A/B = 1 deviate away from the hot wall due to the presence of weak zone there. It is evident that the wall temperature appears to be distributed disproportionately as the fluid flow moves towards downstream. After the step, when the recirculation zone forms, temperature is seen to decrease progressively until it attains its minimum value and then begins to increase along the downstream wall again.
For all the considered cases, Figure (a-c) present the variation of the values of at various values of
and
It has been noticed that the presence of obstacle causes an increase in the values of
. It has also been found that the values of
increases as the value of
increases. It is well known that Δp is mostly dependent of vortexes and the secondary flows. Fluid momentum is dampened and kinetic energy is lost, when obstacles are in the path of the fluid’s flow. When the fluid interacts with an obstruction, the fluid velocity decreases and the Δp increases, which forms pressure gradients. In the Figure (a-c), it has been demonstrated that for
, the values of Δp become almost same at all the values of
The graph shows how much Δp is greatly impacted by the presence of obstacle as compared to the same for smooth channel [Citation29]. With the increase in
, the value of Δp changes significantly because of higher viscosity and density of the nano-fluid. Therefore, momentum decreases and Δp increases as the amount of fluid depletion along the channel or the velocity of the fluid in the indirect pathways increases. It has been found that the presence of plane obstacle;
causes greatest pressure loss as compared to other considered cases (Figure a, b, c). In addition, due to a quick change in pressure over the top of the obstacle, the plane obstacle,
experiences the lowest value of Δp. In case of plane obstacle,
and
, it has been found that the value of Δp becomes approximately 1.173 times as compared to that for smooth channel [Citation29], without considering the obstacle. In case of plane obstacle (A/ B = 1), Figure (d) presents the plots of
at various values of
, and
An increase in
, and wt.% causes an increase in the flow rate and in Δp. It needs powerful pumps to achieve fluid velocity. An increase in the values of
, density and the adhesiveness of cooling fluid to the surface causes the improvement in HT. At
it has been found that the value of
becomes approximately 1.1 times that for
Figure (a–b) depict the profiles of
on the lower wall at
(Figure a) and
(Figure b) in the case of plane obstacle i.e. for
. It has been noticed that the variations in velocity along the channel have desirable effects on the behaviour of
at small values of
and
attains its maximum value at R
. In the backward-facing and shrinking channel, transition of flow through the narrow section of the channel to the thicker one reduces
. However, such sudden changes are not found at higher values of
. With the increase in axial velocity causes the shear stress becomes higher, i.e.
increases. The fluid momentum is enhanced as the fluid velocity rises, which results in a decrease in the vertical velocity gradients at the channel’s intake. Figure (a-b) present that the reduction in
occurred at the expanded part of the channel due to an increase in the fluid velocity. To study the effect of obstacle on the thermal characteristics, the graphs of
along the lower wall of the channel are presented in the Figure (c) at
and
. The highest Nu(x) peak has been seen at the baffle wall, when the flow passed through the recirculation zone. At higher
, it is seen that the increase in
of the fluid induces the increase in
. Because of that, the temperature distribution becomes homogeneous, and the HT rate between wall and fluid increases. Owing to decrease in the boundary layer thickness, the rate of HT between the wall and fluid increases after the inlet segment of the channel, and the rate of HT and
decreases at the downstream. Therefore, at the inlet of the channel, the rate of HT reduces significantly and after that, a steady downward trend is observed as the fluid moves towards the obstacle. As a result,
rises after the expansion region up to a certain length, and then decreases in the downstream. Again, larger cross-sectional area of plane obstacle (A/B = 1) provides highest inertia force due to the decrease in the flow at cross-sectional area, which results in a higher rate of HT. Obstacles are another component that elevates the
and generates a sharp spike in obstacle-roughened areas. The fundamental source of this increase is the enhanced mixing of fluid layers between hot and cool regions. When the fluid travels past the obstacles the thermal barrier layer is broken and altered, which eventually increases the rate of heat transmission. For all the forms of the channel, Figure (a, b) show the plots of
at several values of
It has been found that the increase of the fluid velocity (values of
) causes the enhancement of the values of
. As the value of
increases, the value of
increases due to the improvement in HT mechanism (nano-fluid’s increased thermal conductivity). In the systems associated with nano-fluids, the cooling fluid can be used at a higher value of
Use of nano-fluid in the creep flow regime has no particular effect on its heat transfer performance due to the formation of weak cells near the lower heated wall as the step in the channel disrupts the heat flow. It has been found that as the value of
rises, an increase in the recirculation cell is created within the weak zone. It has been demonstrated that the presence of obstacle causes the enhancement of the values of
. The temperature difference increases between the channel wall and the nano-fluid as the value of
rises. In addition, it has also been revealed that in the case of plane obstacle (A/B = 1), the value of
become more pronounced than the other considered cases. The Figure (c, d) indicate the variations in
at several values of
, and
. An increase in the values of
causes an enhancement of the boundary layer thickness, as a result the value of F decreases. In fact, for lower values of
, the cooling fluid comes close to the channel surfaces. As the flow velocity increases, the flow starts to rotate along the wall of non-viscous region. An increase in
enhances nano-fluid viscosity, and causes loss in the momentum of thicker fluid. In addition, for higher values of
, the particles concentrate in the channel wall as the value of F changes significantly. Moreover, it has been revealed that in case of the plane obstacle (A/B = 1), the value of
and
becomes approximately 1.473, and 1.11 times of those of the case of smooth channel [Citation29].
4.2. Influence of 
, 
on the generation of entropy
In this subsection, we have discussed the impact of and
on the generation of entropy in the case of the plane obstacle, A/B = 1. For several values of
Figure (a) presents the variation in the generations of
and
entropy at
. With the increase in the effective viscosity, it has been noticed that the variation in the generations of
and
entropy exhibit opposite trends, as the value of
increases. However, as the
rises, the viscosity and density of nano-fluid both increases, as a result the shear stress value increases. For having opposite trends in the variations in the generations of
and
entropy with
, the value of
entropy becomes minimum, as seen in the Figure (a). The highest value of
occurs at
, whereas
provides the lowest value of thermal entropy. This may be due to that highest thermal conductivity for all the nano-particles considered in this work occurred at
. Equation 21 demonstrates that the thermal entropy generation changes inversely with effective thermal conductivity, and it demonstrates that
generation is linearly proportional to the effective density of nano-fluid. The rise in the nano-fluid’s viscosity and effective thermal conductivity leads to an irreversible increase in fluid friction and an augmentation of HT, as a result the
generation decreases as the
increases. In the Figure (b–d), we have graphically demonstrated the influence of
on the generation of entropy in terms of
,
,
entropy and Be number for several values of
. As the value of
decreases, it has been found from the Figure (b–d) that the profiles of
increases (Figure b), and
decreases. In addition, it has been demonstrated that the increase in the values of
causes an enhancement in the profile of
To comprehend this pattern; we have to understand that the purpose of including nano-particles is to increase the rate of heat transfer by enhancing the thermal conductivity of the nano-fluid. Reduced temperature gradients within the fluid because of increased thermal conductivity lessen the entropy produced by heat transfer. Conversely, a rise in the volume fraction also causes the nano-fluid’s viscosity to increase, which in turn increases the entropy produced by fluid friction. At several values of
, the Figure (c) depicts the generations of
entropy. An increase in the
generation is caused due to the enhancement of F, as seen from the equation 21. It has been noticed that the profiles are small and almost same for all the considered
. However, with the increase in
, and
the generation of
entropy attains its highest value. Variations in the generation of
entropy with
are depicted in the Figure (
) for several values of
. In addition, as the values of
and
enhanced, the generation of entropy, due to loss in friction, becomes significant. It has been found that at
, the total entropy generation for
becomes about
times of that at
. For different values of
, how the
number changes with the variations in
, is depicted in the Figure (e). The first finding is that, for all the parameters taken into consideration, the Be number is very close to 1. This leads to the conclusion that regardless of
, the entropy generated owing to HT is excessively high for a mildly viscous base fluid, like water. Additionally, we note that
number falls as
increases. Two main causes of this are the rise in entropy produced by fluid friction at high
and the fall in entropy produced by HT because of the fluid’s decreasing temperature gradients. It has been found that an increase in
causes an enhancement of thermal conductivity of nano-fluid to increase. As a result, the fluid’s temperature gradients become lesser, which reduces the entropy produced by HT. Furthermore, a rise in viscosity results in an increase in the entropy produced by fluid friction. For both occurrences, the
value diminishes.
Conclusions
In the present work, the hydro-thermal properties of water/FMWCNT nano-fluid flow, and the characteristics of entropy generation through a backward facing channel embedded with obstacle under uniform heat flux have been studied numerically with the variations in the values of ,
, and forms of the obstacles. This present work is the extension or updated work of Alrashed et al. [Citation29], where they studied the water-FMWCNTs nano-fluid flow characteristics in a backward facing smooth channel, and without considering the analysis of entropy generation. The governing equations (continuity, x-momentum, y-momentum, and energy) have been solved using FVM, and fluent software has been used to visualize the simulation results. The impact of various forms of obstacle (plane, elliptical, and triangular), ratio of obstacle height to width (A/B), ratio of obstacle pitch to width
,
, and the values of
on the different rheological behaviour of nano-fluid flow phenomena have been investigated through graphs. In addition, for several values of
, and
, the generations of
,
, and
have been explored. The concluding remarks are as follows:
It has been found that an increase in the values of
, and
leads to an improvement in velocity values, and the length of recirculation zone. As the value of
rises, the recirculation zone’s size initially reduces in between the expansion zone and obstacle, but after crossing the obstacle zone the length of the recirculation zone increases. It has also been found that the profile of velocity streamlines becomes more pronounced in case of plane obstacle (A/B = 1) than that of the other considered cases.
It has been revealed that the presence of obstacle causes an increase in the values of
. In case of plane obstacle,
and
, it has been concluded that the value of Δp become approximately 1.173, 1.154, 1.136, 1.105, and 1.051 times of the value of Δp in the case of smooth channel (without obstacle), plane (
= 2), plane (A/B = 2), triangular, elliptic shape obstacles respectively.
In case of plane obstacle,
and
it has been found that the value of
become approximately 1.1 times of the sane at
It has been demonstrated that the presence of obstacle causes the enhancement of the values of
.
An increase in the values of
causes an enhancement in the boundary layer thickness, as a result the value of F decreases. Moreover, it has been revealed that in the case of the plane obstacle (A/B = 1), the value of
and
becomes approximately 1.473, and 1.11 times of those in the case of smooth channel.
With the increase in the effective viscosity, it has been revealed that the variation in the generations of
and
entropy exhibits opposite trends, as the value of
and
increases. In addition, the rise in the nano-fluid’s viscosity and effective thermal conductivity leads to an irreversible increase in fluid friction and an augmentation of HT, as a result the
generation decreases as the
increases.
Frictional thermal entropy generation becomes maximum near the lower heated wall and found minimum near the obstacle. It has been found that at
, the total entropy generation for
becomes about
times of that at
.
Furthermore, in the case of the improved heat transmission in terms of the value of
, performance of obstacles in comparison to smooth channel, we have found as below order:
plane shape obstacle (A/B = 1)>elliptical shape obstacle >triangular shape obstacle >plane shape obstacle (A/B = 2) >plane shape obstacle (
) > smooth channel (without considering obstacle).
Based on these observations, future research can create more sophisticated and environmentally friendly energy systems. The current study’s findings will be extremely beneficial in a variety of engineering applications, including smart grid systems, glass blowing, fibre spinning, and continuous metal casting, especially in a variety of manufacturing processes like transpiration cooling, fabric cleaning, and laser pulse heating.
Acknowledgment
We really appreciate all of the reviewers’ insightful comments, which have helped to elevate the calibre of the work.
Disclosure statement
No potential conflict of interest was reported by the author(s).
References
- Akbari OA, Toghraie D, Karimipour A, et al. Investigation of Rib’s height effect on heat transfer and flow parameters of laminar water-Al2O3 nanofluid in a two dimensional rib-micro-channel. Appl Math Comput. 2016; 290:135–153.
- Akbari O, Marzban A, Ahmadi G. Evaluation of supply boiler repowering of anexisting natural gas-red steam power plant. Appl Therm Eng. 2017;124:897–910.
- Behnampour A, Akbari OA, Safaei MR, et al. Analysis of heat transfer and nanofluid fluid flow in micro-channels with trapezoidal, rectangular and triangular shaped ribs. Physica. 2017;91:15–31.
- Bahmani MH, Sheikhzadeh G, Zarringhalam M, et al. Investigation of turbulent heat transfer and nanofluid flow in a double pipe heat exchanger. Adv Powder Technol. 2018;29:273–282.
- Chamkha AJ, Dogonchi AS, Ganji DD. Magneto-hydrodynamic nano-fluid natural convection in a cavity under thermal radiation and shape factor of nanoparticles impacts: a numerical study using CVFEM. Appl Sci. 2018;8(12):2396.
- Ghasemi K, Siavashi M. Three-dimensional analysis of magneto hydrodynamic transverse mixed convection of nano-fluid inside a lid-driven enclosure using MRT-LBM. Int J Mech Sci. 2020;165:105–125.
- Jino L, Kumar VA. MHD natural convection of hybrid nanofluid in a porous cavity heated with a sinusoidal temperature distribution. Comput Therm Sci. 2021;13:83–99.
- Lv J, Huang Q, Yu X, et al. Hydrothermal performance improvement of micro-scale backward-facing step channel by employing vortex generators and nano-fluids. Comput Therm Sci. 2022;12:289–303.
- Parsaiemehr M, Pourfattah F, Akbari OA, et al. Turbulent flow and heat transfer of water/Al2O3 nanouid inside a rectangular ribbed channel. Phys E Low-dimension Syst Nano Struct. 2018;96:73–84.
- Mashayekhi R, Khodabandeh E, Bahiraei M, et al. Application of a novel conical strip insert to improve the efficiency of water-Ag nanofluid for utilization in thermal systems: a two-phase simulation. Energy Convers Manag. 2017;151:573–586.
- Revathi G, Saikrishnan P, Revathy M, et al. Numerical study of Darcy Forchheimer unsteady mixed convection flow of nanofluid with an exponential decreasing free-stream velocity distribution. Comput Therm Sci. 2020;12:289–303.
- Ushachew EG, Sharma MK, Makinde OD. Numerical study of MHD heat convection of nanofluid in an open enclosure with internal with internal heated objects and sinusoidal heated bottom. Comput Therm Sci. 2021;13:1–16.
- Toghraie D, Abdollah MMD, Pourfattah F, et al. Numerical investigation of flow and heat transfer characteristics in smooth, sinusoidal and zigzag-shaped micro-channel with and without nano-fluid. J Therm Anal Calorim. 2018;131:1757–1766.
- Mashaei PR, Taheri-Ghazvini M, Moghadam RS, et al. Smart role of Al2O3-water suspension on laminar heat transfer in entrance region of a channel with transverse in-line baffles. Appl Therm Eng. 2017;112:450–463.
- Mousavi SS, Hooman K. Heat and fluid flow in entrance region of a channel with staggered baffles. Energy Convers Manage. 2016;47(15):2011–2019.
- Sripattanapipat S, Promvonge P. Numerical analysis of laminar heat transfer in a channel with diamond-shaped baffles. Int Comm: Heat Mass Transfer. 2009;36(1):32–38.
- Siddiqui MK. Heat transfer augmentation in a heat exchanger tube using a baffle. Int J Heat Fluid Flow. 2007;28(2):318–328.
- Bouchenafa R, Saim R. Effect of position and height of a shield on convective heat transfer performances of plate fin heat sink. Int J Numer Methods Heat Fluid Flow. 2015;25(5):1047–1063.
- Torii K, Kwak KM, Nishino K. Heat transfer enhancement accompanying pressure-loss reduction with winglet-type vortex generators for fin-tube heat exchangers. Int J Heat Mass Trans. 2002;45:3795–3801.
- Boudiaf A, Danane F, Benkahla YK, et al. Heat transfer analysis of nanofluid flow through backward facing step. MATEC Web Conf; 2020; 307: 01-10.
- Nie JY, Chen TY, et al. Effects of a baffle on separated convection flow adjacent to backward-facing step. Int J Therm Sci. 2009;48(3):618–625.
- Abu-Nada E. Application of nanofluids for heat transfer enhancement of separated flows encountered in a backward facing step. Int J Heat Fluid Flow. 2008;29:242–249.
- Al-Aswadi AA, Mohammed HA, Shuaib NH, et al. Laminar forced convection flow over a backward facing step using nanofluids. Int Commun Heat Mass Transfer. 2010;37:950–957.
- Mohammed HA, Al-aswadi AA, Abu-Mulaweh HI, et al. Influence of nanofluids on mixed convective heat transfer over a horizontal backward-facing step. Heat Transf Asian Res. 2011; 40(4):287–307.
- Kherbeet AS, Mohammed HA, Salman BH, et al. Experimental study of nanofluid flow and heat transfer over microscale backward and forward-facing steps. Exp Thermal Fluid Sci. 2015;65:13–21.
- Amiri A, Arzani HK, Kazi SN, et al. Backward-facing step heat transfer of the turbulent regime for functionalized grapheme nanoplatelets based water–ethylene glycol nanofluids. Int J Heat Mass Transf. 2016;97:538–546.
- Nath R, Krishnan M. Numerical study of double diffusive mixed convection in a backward facing step channel filled with Cu-water nanofluid. Int J Mech Sci. 2018;153:48–63.
- Salman S, Hilo A, Nfawa SR, et al. Numerical study on the turbulent mixed convection heat transfer over 2D microsclae backward facing step. CFD Lett. 2019;11:31–45.
- Alrashed AA, Akbari OA, Heydari A, et al. The numerical modeling of water/FMWCNT nanofluid flow and heat transfer in a backward-facing contracting channel. Phys B. 2018;537:176–183.
- Hilo AK, Iborra AA, Sultan MTH, et al. Experimental study of nanofluids flow and heat transfer over a backward-facing step channel. Powder Technol. 2020;372: 497–505.
- Abdulrazzaq T, Togun H, Alsulami H, et al. Heat transfer improvement in a double backward-facing expanding channel using different working fluids. Symmetry (Basel). 2020;12:1088.
- Ma Y, Mohebbi R, Rashidi MM, et al. Baffle and geometry effects on nanofluid forced convection over forward- and backward-facing steps channel by means of lattice Boltzmann method. Physica A. 2020;554:124696.
- Abedalh AS, Shaalan ZA, Yassien HNS. Mixed convective of hybrid nanofluids flow in a backward facing step. Case Stud Therm Eng. 2021;25:100868.
- Geridönmez BP, Öztop HF. Effects of inlet velocity profiles of hybrid nanofluid flow on mixed convection through a backward facing step channel under partial magnetic field. Chem Phys. 2021;540:111010.
- Wu WC, Kumar A. Numerical investigation of nanofluid flow over a backward facing step. Aerospace. 2022;9(9):499.
- Klazly M, Bognar G. Heat transfer enhancement for nanofluid flows over a microscale backward-facing step. Alex Eng J. 2022;61:8161–8176.
- Klazly M, Mahabaleshwar US. Comparison of single-phase Newtonian and non-Newtonian nanofluid and two-phase models for convective heat transfer of nanofluid flow in backward-facing step. J Mol Liq. 2022;361:119607.
- Klazly M, Bognar G. CFD investigation of backward-facing step nanofluid flow. MMCTSE 2020. J. Phys. Conf. Ser; 2020; 1564; 012010.
- Memon AA, Usman WAK. Numerical investigation of photovoltaic thermal energy efficiency improvement using the backward step containing Cu-Al2O3 hybrid nanofluid. Alexandria Eng J. 2023;75:391–406.
- Amrollahi A, Rashidi AM, Lotfi R, et al. Convection heat transfer of functionalized MWNT in aqueous fluids in laminar and turbulent flow at the entrance region. Int Commun Heat Mass Transfer. 2010;37:717–723.
- Safaei MR, Togun H, Vafai K, et al. Investigation of heat transfer enchantment in a forward-facing contracting channel using FMWCNT nanofluids, Numer. Heat Transfer – Part A. 2014;66:1321–1340.
- Nikkhah Z, Karimipour A, Safaei MR, et al. Forced convective heat transfer of water/functionalized multi-walled carbon nanotube nanofluids in a microchannel with oscillating heat flux and slip boundary condition. Int Commun Heat Mass Tran. 2015;68:69–77.
- Gholami MR, Akbari OA, Marzban A, et al. The effect of rib shape on the behavior of laminar flow of oil/MWCNT nanofluid in a rectangular microchannel. J Therm Anal Calorim. 2018;134:1611–1628.
- Karimipour A, Alipour H, Akbari OA, et al. Studying the effect of indentation on flow parameters and slow heat transfer of water-silver nanofluid with varying volume fraction in a rectangular two- dimensional micro-channel. Ind J Sci Tech. 2015;8:51–70.
- Ghasemi B, Aminossadati MS. Brownian motion of nanoparticles in a triangular enclosure with natural convection. Int J Therm Sci. 2010;49:931–940.
- Oztop FH, Abu-Nada E. Numerical study of natural convection in partially heated rectangular enclosures filled with nanofluids. Int J Heat Fluid Flow. 2008;29(5):1326–1336.
- Palm S, Roy G. Heat transfer enhancement with the use of nanofluids in a radial flow cooling system considering temperature dependent properties. Appl Therm Eng. 2006;26:2209–2218.
- Akbarinia A, Behzadmehr A. Numerical study of laminar mixed convection of a nanofluid in horizontal curved tubes. Appl Therm Eng. 2007;27:1327–1337.
- Koo J, Kleinstreuer C. A new thermal conductivity model for nanofluids. J Nanopart Res. 2004;6(6):577–588.
- Vajjha SR, Das KD. Experimental determination of thermal conductivity of three nanofluids and development of new correlations. Int J Heat Mass Transf. 2009;52(21):4675–4682.
- Brinkman HC. The viscosity of concentrated suspensions and solution. J Chem Phys. 1952;20:571–581.
- Tiwari MK, Das T. Heat transfer augmentation in a two-sided lid-driven differentially heated square cavity utilizing nanofluids. Int J Heat Mass Transf. 2002;50(9):2002–2018.
- Bejan A. Entropy generation minimization: the method of thermodynamic optimization of finite-size systems and finite-time processes. New York (NY): CRC Press; 1995.
- Bianco V, Nardini S. Enhancement of heat transfer and entropy generation analysis of nanofluids turbulent convection flow in square section tubes. Nanoscale Res Lett. 2011;6:1–12.
- Kalinin M, Kononogov S. Boltzmann’s constant, the energy meaning of temperature, and thermodynamic irreversibility. Meas Tech. 2005;48(7):632–636.
- Akbari AO, Toghraie D. Impact of ribs on flow parameters and laminar heat transfer of water–aluminum oxide nanofluid with different nanoparticle volume fractions in a three-dimensional rectangular microchannel. Adv Mech Eng. 2015;7(11):1–11.
- Alipour H, Karimipour A, Safaei MR, et al. Influence of T-semi attached rib on turbulent flow and heat transfer parameters of a silver-water nanofluid with different volume fractions in a three-dimensional trapezoidal microchannel. Phys E Low-dimens Syst Nanostruct. 2017;88:60–76.
- Hosseinnezhad R, Akbari OA, Hassanzadeh Afrouzi H, et al. The numerical study of heat transfer of turbulent nanofluid flow in a tubular heat exchanger with twin twisted-tapes inserts. J Therm Anal Calorim. 2018;132:741–759.
- Ayad SA, Shaalan AZ. Mixed convective of hybrid nanofluids flow in a backward-facing step. Case Studies Therm Eng. 2021;25:100868.