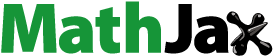
Abstract
Due to distinct photoelectric absorption properties and physiochemical features, high-atomic-element nanomaterials are frequently used as radiosensitizer for radiotherapy, such as gold nanoparticles. which in-turn can increase radiation dose to tumours while minimizing exposure to normal tissues. The aim of this study is to investigate the dose enhancement due to the presence of nanogold particles mixed with breast tissue using a real radiotherapy plan with two photon energies, with and without flattening filter. A fully validated linac head was used to model a complete field-in-field (FIF) radiotherapy breast plan using 4 and 6 MV with a flattening filter (FF) and flattening filter-free (FFF) using BEAMnrc/EGSnrc MC code. The dose distributions were simulated in a female ICRP voxel computational phantom. The dose enhancement ratio (DER) and flattening filter-free enhancement ratio (FFFER) were calculated for a range of nanogold concentrations. The results showed that the DER increased with increasing nanogold concentration. The maximum DER was achieved when FFF was used and the increase was higher for 4 MV than 6 MV. The FFFER was higher for the 4 MV beam than 6 MV when 40 mg/ml nanogold was used. The combination of higher nanogold concentrations, lower photon energies, and FFF resulted in a higher dose enhancement. The implementation of nanogold particles in breast tissue enhanced the dose deposition, especially with FFF, as presented in this study. The results would improve nanogold-enhanced breast radiotherapy and dose delivery with an increase in patient throughput.
1. Introduction
The most frequent malignancy in women is breast cancer. According to the World Health Organization (WHO) statistics from 2020, 2.3 million women were diagnosed with breast cancer, with more than 500,000 fatalities, worldwide [Citation1]. From 2000 to date, there has been a noticeable increase in radiation-treated survivors with breast cancer [Citation2]. Radiotherapy is the most frequently used treatment for breast cancer, and it can be used to treat the disease at practically any stage. Radiotherapy is used to treat early and terminal breast cancers, as well as metastatic tumours and regional lymph nodes [Citation3, Citation4]. The main goal of radiotherapy is to deliver as high as possible a dose to the cancer site while minimizing damage to surrounding healthy tissue [Citation5]. With advances in external breast radiotherapy, better toxicity profiles have been determined for the skin, lung, and heart [Citation6]. However, this treatment still has certain limitations due to toxic side effects such as dose heterogeneity, local discomfort, and long-term exposure to healthy tissue [Citation7].
To enhance the therapeutic effect of radiation, a higher dose to the target organ is required in addition to sparing as much healthy tissue as possible, which is still a challenge for radiation oncologists. Amongst all efforts to improve radiotherapy outcomes, nanoparticle-enhanced radiation represents a promising approach that has seen growing interest from a research perspective over the last decade [Citation8]. Despite there being a large number of published studies in this field, clinical data is still insufficient to justify using this approach to enhance radiation dosage [Citation9]. In order to understand the role of nanoparticles in the dose, their use needs to be fully understood biologically, physically, and clinically.
From a physics point of view, high-atomic-number-element nanomaterials are frequently used as radiosensitizers because of their unique photoelectric decay characteristics, which can be used to increase the dose in the target without effecting normal healthy tissue [Citation10–13]. Commonly used nanoparticles include bismuth (Z = 83), gold (Z = 79), and silver () [Citation14–16]. This is due to their strong attenuation of photons, and ability to increase the deposition of radiation as they have much higher mass-energy absorption coefficients than soft tissues, as well-being biocompatible and having low toxicities [Citation17]. Gold nanoparticles are amongst those the most extensively studied, and are regarded as good radiosensitizers for radiotherapy [Citation9]. From early studies on mice, this interest was initially driven by its ability to increase the dose deposition within target volumes, even at low concentrations [Citation18–20].
For clinical kVp and MV spectral X-ray photons, several studies have demonstrated the dose enhancement effect, be this computationally or experimentally. Computational studies are based mainly on the Monte Carlo (MC) method [Citation21–27], while experimental studies are based on cell survival data or imaging studies showing nanoparticle uptake by tumour and other cells [Citation28–30].
Initial studies on dose enhancement of nanogold with high -energy MV radiotherapy showed a relatively low ratio dose enhancement compared to low energy kV radiotherapy. However, there several intersecting factors that influence this enhancement that need to be addressed and studied with regard to the effects of beam quality and nanoparticle properties and concentrations. Dose enhancement due to nanoparticles is very sensitive to a number of factors in the case of external radiotherapy such as depth and location [Citation25], X-ray photon energy [Citation21], beam quality [Citation31, Citation32], and the use of flattening filter-free beams [Citation23, Citation25]. These studies showed that low MV photon energies without a flattening filter in place and at a shallower depth give a higher dose enhancement ratio.
These factors need to be studied physically and an understanding of the influence of each on enhancing the dose gained. The MC approach represents a highly accurate method by which to calculate the physical enhancement of doses with nanogold. However, the use of nanogold as radiosensitizer in breast cancer external radiotherapy has still not been studied to date using the MC method.
In this study, the dose enhancement ratio of nanogold implemented within breast cancer treated using an advanced radiotherapy plan was investigated using two different photon energies, 4 and 6 MV. The MC method was used with an ICRP female computational phantom to calculate the dose enhancement with different concentrations of nanogold. In addition, the effect of removing the flattening filter was investigated. This is first study on dose enhancement using nanogold for high energy MV photon beams for breast cancer.
2. Method and materials
2.1. MC linac model
An Elekta Synergy linear accelerator (version 4.5, ElektaTM, Crawley, West Sussex, UK) was used in this study. The linac head was built and modelled using the BEAMnrc/EGSnrc MC code [Citation33]. The model was previously validated [Citation34]. In the BEAMnrc code, most simulation parameters were left at their default values. However, several variance reduction techniques were used to increase the efficiency. Directional bremsstrahlung splitting (DBS) was selected with a splitting number of 15,000 and a splitting field radius that varies according to the field size, as it should encompass the entire field [Citation35]. The rejection plane was defined as being 85 cm. Bremsstrahlung cross-section enhancement (BCSE) was used in combination with DBS. The enhancement constant was set to 20 and the enhancement power was set to 0. In addition, the range rejection technique was used, where 2 MeV was used as an ESAVE value. The cut-off energies for photons and electrons were set to 10 and 700 keV, respectively.
The phase-space (PHSP) files were scored at the bottom of the linac for 4 and 6 MV with a flattening filter (FF) and without a flattening filter, the latter being referred to as flattening filter-free (FFF). The field size was cm
defined at 100 cm and a 90 cm source-surface distance (SSD). Consequently, each PHSP file was analysed via the BEAMdp code to obtain photon fluence, energy fluence, and mean energy [Citation36].
2.2. ICRP voxel computational female phantom
The ICRP female voxel phantom is based on the whole body CT image of a 43-year-old individual who suffered from leukaemia, with 167 cm height and 59 kg weight (Reference female: 167 cm and 60 kg) [Citation37]. The original data consisted of 174 slices with 5 mm thickness for head and trunk, and 43 slices, each with a 20 mm thickness, for legs. Interpolation for the 43 slices was performed to obtain 5 mm slices. The original data after the interpolation consisted of 346 slices of pixels with a voxel volume of 17.6 mm
(5 mm in height and 1.875 mm in-plane). The reconstructed phantom consists of 348 slices of
pixels with a voxel volume of 15.25 mm
(4.84 mm in height and 1.775 mm in-plane). The ICRP female voxel phantom consists of 141 segmented organs with 53 defined media. Small structures were not segmented because of the limited resolution of the images.
In this study, the hands were removed from the ICRP female computational phantom because they were not in a correct clinical position. Using provided atomic compositions in the ICRP report [Citation35] and EGS_gui, the “pegs file” for the female voxel phantom was created. The resultant “egsphant” file was then converted to DICOM images to use it with the treatment planning system (TPS).
Nanogold-tissue mixture will be used in the ICRP female phantom to model microscopically the implementation of nanogold in breast tissue. The PEGS/EGSnrc code was used to generate the material data library of nanogold particles with different concentrations in the MC calculations. In this study, different concentrations of nanogold were mixed with the breast tissue. These concentrations were 3, 7, 18, 30, and 40 mg/ml (by mass), which were chosen based on small-animal experiments in nanoparticle-enhanced radiotherapy [Citation19]. Using higher concentration, toxicity may be observed [Citation19].
2.3. Field-in-field plan
A breast field-in-field (FIF, some may call it IMRT) plan was initially created in treatment planning software using the DICOM of the ICRP phantom. The plan combined two tangential open fields with two conformal fields, as shown in Figure . The field sizes were ,
,
, and
cm
with a 95.9 and 92 cm SSD. The prescribed dose is 40 Gy in 15 fractions over 3 weeks (2.67 Gy/fraction). The beam weights were manually adjusted to optimize the target coverage and minimize the dose to the ipsilateral lung. Then, the created treatment plan was simulated using the BEAMnrc code according to give field size, beam angle, MLC position, and monitor units for each field using 4 and 6 MV beam energies with and without FF.
Figure 1. (a) A 3D view of an ICRP computational female phantom without hands, and (b) a transverse view with MC dose distribution of the FIF plan normalized at the isocentre.

Finally, the dose distribution within the ICRP of each FIF plan was simulated using the DOSXYZnrc user code [Citation38]. A Linux Ubuntu PC with a 3.4 GHz 16-processor CPU and 32 Gb of RAM was used to run all the simulations, with each simulation split into 32 parallel jobs. The statistical uncertainty was maintained at about 1% across the field for each beam in each plan. For each nanogold concentration, the resultant MC dose distribution files (3ddose) of each plan were imported into MATLAB and then combined and analysed.
2.3.1. DER and FFFER
The dose enhancement ratio (DER) and flattening filter-free enhancement ratio (FFFER) were calculated in order to analyse the effect of nanogold implementation as well as the effect of removing flattening filter. These ratios were calculated for a complete FIF plan for each nanogold concentration using 4 and 6 MV photon beams with FF and FFF. The DER was calculated using the formula below [Citation25]:
(1)
(1) and the FFFER was calculated using the following formula [Citation25]:
(2)
(2)
3. Results and discussion
In this study, the nanogold particles were evenly distributed within the breast tissue for all concentrations. Thus, the nanogold size and shape were not considered, and consequently all the MC simulations were carried out according to the macroscopic approach. The effect of considering the nanogold shape and size is not as significant as the total mass percentage of nanogold particles [Citation22, Citation25].
Figure shows the photon fluence and energy fluence for 4 and 6 MV photon beams with FF and FFF. Both photon fluence and energy fluence are normalized by number of initial primary source particles, in this case, electrons. For the 4 MV photon beam, as expected, the number of low energy photons was remarkably increased when the FF was removed, as there is no beam hardening. However, the mean energy well inside the field (≤6 cm) of the 4 MV FFF was 1.02 MeV, while with FF the mean energy was 1.21 MeV. For 6 MV photon beam, similar observations were made where the mean energies were 1.39 and 1.79 MeV for FF and FFF, respectively.
Figure 2. (a) Photon fluence, and (b) energy fluence for 4 and 6 MV photon beams with flattening filter, and when flattening filter-free (FFF).

3.1. DER comparison
Figure shows the DER for the FIF plan using a 4 MV photon beam with FF and FFF for different concentrations of nanogold (3, 7, 18, 30, and 40 mg/ml) mixed with the breast tissue. In general, it can be clearly seen that the DER increased with increasing nanogold concentration. The minimum DER was found when 3 mg/ml nanogold was used where the DER was 1.0103, as shown in Table . On the other hand, the maximum DER was found when 40 mg/ml nanogold was used where the DER was 1.0453. This means that the dose was enhanced by about 4.5%. Compared with the FF beam, the FFF beam resulted in significantly increased DER values. For the FFF beam, the minimum and maximum DERs were 1.0313 and 1.1011 when 3 and 40 mg/ml nanogold were used, respectively.
Figure 3. Dose enhancement ratio for different concentrations of nanogold using a 4 MV beam with flattening filter (FF) and when flattening filter-free (FFF).

Table 1. The DER values of the FIF breast plan using 4 and 6 MV photon beams with FF and FFF.
Figure shows the DER for the FIF plan using a 6 MV photon beam with FF and FFF for different concentrations of nanogold. Similar to the 4 MV photon beam results, the DER increased with increasing nanogold concentration. However, in general, the increase in the DER was less than the DER values when using the 4 MV photon beam with FF or FFF.
Figure 4. Dose enhancement ratio for different concentrations of nanogold using a 6 MV beam with flattening filter (FF) and without flattening filter (FFF).

For both the 4 and 6 MV photon beams, the reason for the increase in the DER values with increasing nanogold concentration was due to the fact that the photoelectric effect cross-section increases with the atomic number for low-energy photons [Citation23]. Thus, the more the nanogold in the breast tissue, the higher the cross-section of the photoelectric effect. In addition, the FF photon beam was flattened, thus providing a more uniform profile and homogenous dose distribution. Consequently, the number of low energy photons is decreased and the mean energy increased. On the other hand, the FFF photon beam contains more low energy photons, thus has a lower mean energy. The lower the mean energy, the higher the attenuation of the low energy photons due to the photoelectric effect interactions, leading to increased secondary particles production, consequently more dose is deposited [Citation23, Citation25]. Furthermore, for the same beam type (FF or FFF beam), the 4 MV photon beam resulted in a greater dose enhancement for the 6 MV photon beam as the 4 MV beam, in general, contains more low-energy photons [Citation25]. As a result, these factors affect the dose enhancement within the nanogold particles mixed with the breast tissue.
Martelli and Chow [Citation23] investigated the DER using 6 and 10 MV photon beams with FF and FFF using different nanogold concentrations. Their study was carried out using an oval-shaped water phantom to mimic a patient's pelvis with different prostate size irradiated via a single field ( cm
). The results showed that the 6 MV photon beam was more suitable for maximizing the DER compared with the 10 MV photon beam with FF and FFF. The smaller the prostate size, the greater the DER; however, the FFF photon beam resulted in a greater DER when compared with the FF photon beam for all prostate sizes. The DER values of the 6 MV beam with 40 mg/ml nanogold mixed with 5 cm of prostate material (water) were about 1.08 and 1.13 using FF and FFF, respectively, when using a 40 mg/ml nanogold concentration. In the current study, the DER values for the 6 MV beam were 1.0326 and 1.0856 for FF and FFF, respectively. The apparent deviation might be due to the beam variables or phantom geometry as the breast size was greater than 5 cm and the dose point (in one field of the plan) was beneath 0.5 cm of skin, 4.5 cm of adipose tissue, and about 3 cm of breast tissue mixed with nanogold.
3.2. FFFER comparison
The effect of removing the flattening filter on the dose enhancement by the nanogold particles was investigated. Figure shows the FFFER for the FIF breast plan using 4 and 6 MV photon beams. The FFFER, the DER with FF divided by the DER with FFF using the same energy, increased with increasing nanogold concentration. For all nanogold concentrations, the dose enhancement increased with the FF removed compared to when it was employed for both 4 and 6 MV photon beams. However, the DER was increased more significantly for the 4 MV photon beam than the 6 MV photon beam.
Figure 5. The flattening filter-free enhancement ratio (FFFER) for the 4 and 6 MV photon beams using different nanogold concentrations.

Spina and Chow [Citation25] studied the effects of FF and nanogold particles on depth of dose using 6 and 10 MV photon beams, and calculated the associated FFFER. The study used a pure water phantom and the water was mixed with different nanogold concentrations irradiated by a single field ( cm
). The study found that the FFFER decreased with increasing depth in the phantom, and that the maximum FFFER of the 6 MV photon beam was 1.057 when using a 40 mg/ml concentration of nanogold. The nanogold particles were distributed evenly within the tumour, which is similar to the current study where the maximum FFFER value of the 6 MV photon beam was 1.053. However, even though the study used a single field with a water phantom, the result, in general, was in a good agreement with the current study, a real radiotherapy breast plan with an ICRP female phantom.
Finally, as a result, the combination of a higher nanogold concentration, lower photon beam energy, and FFF resulted in higher dose enhancement within the breast tissue. This would increase the dose rate, consequently reducing treatment time. In addition, head scatter, leakage, and out-of-field dose were reduced, therefore minimizing normal tissue exposure [Citation25, Citation39, Citation40]. This would result in better patient throughput and treatments.
4. Conclusion
Nanogold, as dose enhancement agent for breast radiotherapy, was investigated using the Monte Carlo approach and an ICRP female computational phantom with two different energies, 4 and 6 MV, with FF and FFF. For both energies, the maximum DER was found when a 40 mg/ml nanogold concentration was used. The increase in DER was higher with FFF than with FF, as there is no beam hardening. The FFFER was higher for the lower photon beam energy. The higher the nanogold concentration with the lower photon energy and the use of FFF resulted in an increased dose enhancement. As presented in this study, using a real breast treatment plan with an ICRP female voxel phantom can justify dose enhancement due to the addition of nanogold particles with regard to real patient shape and beam settings. Therefore, it has the potential to improve nanogold-enhanced breast radiotherapy plans and lead to better patient outcomes.
Acknowledgement
The authors gratefully acknowledge the help from staff of the Department of Medical Physics and Clinical Engineering, SBU HB (Swansea Bay University Health Board) for their assistance in this study.
Disclosure statement
No potential conflict of interest was reported by the author(s).
Correction Statement
This article has been corrected with minor changes. These changes do not impact the academic content of the article.
References
- Breast cancer. World Health Organization; [cited 2023 Jul 31]. Available from: https://www.who.int/news-room/fact-sheets/detail/breast-cancer#::text=In%202020%2C%20there%20were%2023,the%20worlds%20most%20prevalent%20cancer.
- Bryant AK, Banegas MP, Martinez ME, et al. Trends in radiation therapy among cancer survivors in the United States, 2000–2030. Cancer Epidemiol Biomarkers Prev. 2017;26(6):963–970. doi: 10.1158/1055-9965.epi-16-1023
- Wang W. Radiotherapy in the management of early breast cancer. J Med Radiat Sci. 2013;60(1):40–46. doi: 10.1002/jmrs.1
- Treatment of breast cancer stages I–III — American Cancer Society [Internet]; [cited 2023 Jul 31]. Available from: https://www.cancer.org/cancer/types/breast-cancer/treatment/treatment-of-breast-cancer-by-stage/treatment-of-breast-cancer-stages-i-iii.html.
- Washington CM, Leaver DT. Principles and practice of radiation therapy-e-book. New York: Elsevier Health Sciences; 2015.
- Karpf D, Sakka M, Metzger M, et al. Left breast irradiation with tangential intensity modulated radiotherapy (t-IMRT) versus tangential volumetric modulated arc therapy (t-VMAT): trade-offs between secondary cancer induction risk and optimal target coverage. Radiat Oncol. 2019;14(1). doi: 10.1186/s13014-019-1363-4
- Hinkelbein W, Bruggmoser G, Frommhold H, et al. Acute and long-term side-effects of radiotherapy: biological basis and clinical relevance. Vol. 130. Berlin, Heidelberg: Springer Science & Business Media; 2012.
- Lee J, Chatterjee DK, Lee MH, et al. Gold nanoparticles in breast cancer treatment: promise and potential pitfalls. Cancer Lett. 2014;347(1):46–53. doi: 10.1016/j.canlet.2014.02.006
- Sarria GR, Berenguer Francés MÁ, Linares Galiana I. Enhancing radiotherapy effect in breast cancer with nanoparticles: a review. Rep Practical Oncol Radiotherapy. 2019;24(1):65–67. doi: 10.1016/j.rpor.2018.10.003
- Kwatra D, Venugopal A, Anant S. Nanoparticles in radiation therapy: a summary of various approaches to enhance radiosensitization in cancer. Transl Cancer Res. 2013;2(4):330–342. doi: 10.3978/j.issn.2218-676X.2013.08.06.
- Song G, Cheng L, Chao Y, et al. Emerging nanotechnology and advanced materials for cancer radiation therapy. Adv Mater. 2017;29(32):1700996. doi: 10.1002/adma.201700996
- Chen Q, Chen J, Yang Z, et al. Nanoparticle-enhancedradiotherapy to trigger robust cancer immunotherapy. Adv Mater. 2019;31(10):1802228. doi: 10.1002/adma.-201802228
- Pallares RM, Abergel RJ. Nanoparticles for targeted cancer radiotherapy. Nano Res. 2020;13(11):2887–2897. doi: 10.1007/s12274-020-2957-8
- Shahbazi–Gahrouei D, Choghazardi Y, Kazemzadeh A, et al. A review of bismuth-based nanoparticles and their applications in radiosensitising and dose enhancement for cancer radiation therapy. IET Nanobiotechnol. 2023;17(4):302–311. doi: 10.1049/nbt2.v17.4
- Her S, Jaffray DA, Allen C. Gold nanoparticles for applications in cancer radiotherapy: mechanisms and recent advancements. Adv Drug Deliv Rev. 2017;109:84–101. doi: 10.1016/j.addr.2015.12.012
- Liu PD, Jin H, Guo Z, et al. Silver nanoparticles outperform gold nanoparticles in radiosensitizing U251 cells in vitro and in an intracranial mouse model of glioma. Int J Nanomed. 2016;11:5003–5014. doi: 10.2147/ijn.s11-5473
- Liu Y, Zhang P, Li F, et al. Metal-based nanoenhancers for future radiotherapy: radiosensitizing and synergisticeffects on tumor cells. Theranostics. 2018;8(7):1824–1849. doi: 10.7150/thno.22172
- Schuemann J, Berbeco R, Chithrani DB, et al. Roadmap to clinical use of gold nanoparticles for radiation sensitization. Int J Radiat Oncol Biol Phys. 2016;94(1):189–205. doi: 10.1016/j.ijrobp.2015.09.032
- Hainfeld JF, Slatkin DN, Smilowitz HM. The use of gold nanoparticles to enhance radiotherapy in mice. Phys Med Biol. 2004;49(18):N309–N315. doi: 10.1088/0031-9155/49/18/N03
- Hainfeld JF, Dilmanian FA, Zhong Z, et al. Gold nanoparticles enhance the radiation therapy of a murine squamous cell carcinoma. Phys Med Biol. 2010;55(11):3045–3059. doi: 10.1088/0031-9155/55/11/004
- Hwang C, Kim JM, Kim J. Influence of concentration, nanoparticle size, beam energy, and material on dose enhancement in radiation therapy. J Radiat Res. 2017;58(4):405–411. doi: 10.1093/jrr/rrx009
- Sheeraz Z, Chow JCL. Evaluation of dose enhancement with gold nanoparticles in kilovoltage radiotherapy using the new EGS geometry library in Monte Carlo simulation. AIMS Biophys. 2021;8(4):337–345. doi: 10.3934/biophy.2021027
- Martelli S, Chow JCL. Dose enhancement for the flattening-filter-free and flattening-filter photon beams innanoparticle-enhanced radiotherapy: a Monte Carlophantom study. Nanomaterials. 2020;10(4):637. doi: 10.3390/nano10040637
- Chow JCL. Depth dose enhancement on flattening-filter-free photon beam: a Monte Carlo study in nanoparticle-enhanced radiotherapy. Appl Sci. 2020;10(20):7052. doi: 10.3390/app10207052
- Spina A, Chow JCL. Dosimetric impact on the flattening filter and addition of gold nanoparticles in radiotherapy: a Monte Carlo study on depth dose using the 6 and 10 MV FFF photon beams. Materials. 2022;15(20):7194. doi: 10.3390/ma15207194
- Vlastou E, Diamantopoulos S, Efstathopoulos EP. Monte Carlo studies in gold nanoparticles enhanced radiotherapy: the impact of modelled parameters in dose enhancement. Phys Med. 2020;80:57–64. doi: 10.1016/j.ejmp.2020.09.022
- Lin Y, McMahon SJ, Scarpelli M, et al. Comparing gold nano-particle enhanced radiotherapy with protons, megavoltage photons and kilovoltage photons: a Monte Carlo simulation. Phys Med Biol. 2014;59(24):7675–7689. doi: 10.1088/0031-9155/59/24/7675
- Zheng Q, Yang H, Wei J, et al. The role and mechanisms of nanoparticles to enhance radiosensitivity in hepatocellular cell. Biomed Pharmacother. 2013;67(7):569–575. doi: 10.1016/j.biopha.2013.04.003
- Butterworth KT, McMahon SJ, Currell FJ, et al. Physical basis and biological mechanisms of gold nanoparticle radiosensitization. Nanoscale. 2012;4(16):4830. doi: 10.1039/-c2nr31227a
- Jain S, Coulter JA, Hounsell AR, et al. Cell-specific radiosensitization by gold nanoparticles at megavoltage radiation energies. Int J Radiat Oncol Biol Phys. 2011;79(2):531–539. doi: 10.1016/j.ijrobp.2010.08.044
- Tsiamas P, Liu B, Cifter F, et al. Impact of beam quality on megavoltage radiotherapy treatment techniques utilizing gold nanoparticles for dose enhancement. Phys Med Biol. 2013;58(3):451–464. doi: 10.1088/0031-9155/58/3/451
- Berbeco RI, Detappe A, Tsiamas P, et al. Low Z target switching to increase tumor endothelial cell dose enhancement during gold nanoparticle-aided radiation therapy. Med Phys. 2015;43(1):436–442. doi: 10.1118/1.4938410
- Rogers DWO, Walters B, Kawrakow I. BEAMnrc users manual; 2009. (NRC Rep., PIRS, 509).
- Al-Saleh WM, Hugtenburg RP. Monte Carlo modelling of a 6 MV Elekta linear accelerator for in-field and out-of-field dosimetry. Radiat Phys Chem. 2023;203: 110584. doi: 10.1016/j.radphyschem.2-022.110584
- Almatani T. Optimisation of variance reduction techniques in EGSnrc Monte Carlo for a 6 MV photon beam of an Elekta synergy linear accelerator. J King Saud Univ Sci. 2021;33(4):101421. doi: 10.1016/j.jksus.2021.101421
- Ma CM, Rogers DWO. BEAMDP as a general-purpose utility; 2004. (NRC Rep., PIRS, 509e (rev A)).
- ICRP. Adult reference computational phantoms. ICRP Publication 110. Ann. ICRP 39; 2009.
- Walters B, Kawrakow I, Rogers D. DOSXYZnrc users manual; 2013. (NRC Rep., PIRS, 794).
- Vassiliev ON, Titt U, Pönisch F, et al. Dosimetric properties of photon beams from a flattening filter free clinical accelerator. Phys Med Biol. 2006;51(7):1907–1917. doi: 10.1088/0031-9155/51/7/019
- Chow JCL, Owrangi AM. Mucosal dosimetry on unflattened photon beams: a Monte Carlo phantom study. Biomed Phys Eng Express. 2018;5(1):015007. doi: 10.1088/2057-1976/aaeaaa