Abstract
Fabricating highly efficient and low-cost absorbents to remove organic contaminants in industrial effluents is urgent yet of great importance. Herein, a facile one-pot hydrothermal approach was developed to successfully construct magnesium silicate and bentonite composite (MS-Bt) with uniform multilayer porous structure by using Bt as structural promoter. The specific surface area of MS-Bt can reach up to 392 m2·g−1 along with an average pore diameter of 2.29 nm and a pore volume of 0.22 cm3·g−1. MS-Bt composite exhibits a maximal adsorption capacity of 253.92 mg·g−1 for methylene blue (MB) in model wastewater treatment. Adsorption behavior fitted well with Langmuir isotherm and pseudo-second-order kinetics adsorption equations. The adsorption pathways of MB on MS-Bt mainly include chemical precipitation by Mg-OH, electrostatic attraction and surface hydroxyl functional group capture. This work is anticipated to give a new insight into constructing organic contaminants adsorbent by using clay mineral material as an auxiliary agent.
1. Introduction
Potable water for human beings has suffered a growing shortage for the increased water demand and pollution, which is resulting from the continuous industrialization and global population increase [Citation1–4]. As organic cationic dyes widely employed in the paper and textile industries, methylene blue (MB) may be clearly identified even at extremely low quantities, which makes them unattractive and extremely colorable [Citation5,Citation6]. Therefore, from the viewpoint of environment, it has become an urgent issue to address how to treat wastewater contaminated with dyes such as MB quickly and effectively before it is discharged to groundwater. Various methods have been explored over the past few years, including adsorption [Citation7,Citation8], photodegradation [Citation9,Citation10], ion-exchange [Citation11,Citation12], electrochemical processes [Citation13,Citation14], biological-degradation [Citation15,Citation16], and filtration technology [Citation17,Citation18]. Among them, adsorption is considered to be better to other approaches due to its relatively low cost, ease of operation, availability of various adsorbents, and high efficiency.
Owning to the superior physical properties, such as singular morphologies and rich network of pore channels, multilayer porous structures have drawn a lot of attention to remove the dye by adsorption [Citation19–21]. Particularly, the porous features may supply a huge specific surface area (SA), pore volume (PV) and abundance of adsorption sites, resulting in many routes for solute diffusion and transport [Citation22,Citation23]. As a result, these fundamental characteristics can dramatically boost adsorption performance. Indeed, numerous works have been made to produce innovative porous materials to deal with harmful dyes. A few examples are listed below, aqueous foams with modified sepiolite as a template for porous adsorbents had an excellent performance for MB and Methyl green (MG) [Citation24]. The aromatic-based porous organic polymers were synthesized from octafluoronaphthalene and decafluorobiphenyl as cross-linking agents. The adsorbent material exhibited extraordinary adsorption capacity for cationic dyes such as Rhodamine B, MB and crystalline violet (CV) [Citation25]. Microwave-assisted combustion was used to create zinc aluminum hydroxide (ZAH) as a porous adsorbent. ZAH exhibited excellent adsorption capacity for anionic dyes [Citation26]. However, their applications are somewhat limited because of the drawbacks of these porous adsorbents such as complex synthesis processes, expensive raw materials and reaction instability [Citation27]. As a corollary, the development of easy-to-operate, environmentally friendly and efficient synthetic methods to produce layered porous adsorbents with excellent performance for the treatment of dyed wastewater is of great significance yet remains a challenge.
Bentonite is a kind of non-metallic mineral with montmorillonite as the main component, and Montmorillonite is a layered aluminosilicate mineral containing a small amount of alkali and alkaline earth metals. Its structural layer is a 2:1 type aluminosilicate with water molecules and exchangeable cations in the interlayer [Citation28,Citation29]. It has been widely used in the field of adsorption due to its low cost, easy access, eco-friendliness, large specific surface area, good porosity and strong cation exchange performance [Citation30,Citation31]. However, due to the hydrophilicity of bentonite and the presence of impurity minerals, its adsorption capacity for organic pollutants is not strong. Bentonite is usually modified to improve its adsorption capacity. At present, the commonly used modification methods include inorganic modification, organic modification and high temperature thermal modification. Among them [Citation32–34], inorganic modification has attracted much attention because it can change the interlayer properties of bentonite and increase the specific surface area of bentonite.
In this work, the MS-Bt composites were synthesized by a single-point hydrothermal method and had an excellent adsorption performance for MB. The structure and properties of MS-Bt composites were determined by XRD, FTIR, BET and SEM. The impacting factors of contact time, pH value, initial MB concentration and temperature were analyzed. The adsorption isotherms, kinetics, thermodynamics and adsorption mechanism of MB were all thoroughly examined.
2. Experimental
2.1. Materials
Magnesium sulfate heptahydrate (MgSO4·7H2O) was purchased from Macklin Biochemical Technology Co. Ltd. Sodium silicate (Na2SiO3·9H2O) was offered from Aladdin Biochemical Technology Co. Ltd (Shanghai). MB trihydrate was offered from Adamas Chemical Reagent Co. Ltd. Bt was received from Xinyang Duyu Minerals Co., Ltd. (Xinyang, China). All solutions used in this study were prepared in deionized water.
2.2. Synthesis of MS-Bt composite
In a typical procedure, 2.0301 g of Na2SiO3·9H2O (7.14 mmol) and 5.0 g of Bt were dissolved in 15 mL of deionized water. Then, 1.0172 g of MgSO4·7H2O (4.13 mmol) was dissolved in a certain volume of water. Under strong magnetic stirring, MgSO4 solution was added to the Na2SiO3-Bt mixed solution drop by drop. After the mixture was stirred at room temperature for 10 min, the resultant slurry was transferred into 50 mL of Teflon lining, which was then heated to 180 °C and kept at this temperature for 18 h. Finally, the supernatant was removed by centrifuge at 6000 rpm for 1 min. The solid was washed by deionized water until the pH test paper detects neutral, and then dried for use.
The component-modulated synthesis of MS-Bt was performed as follows. Na2SiO3·9H2O and MgSO4·7H2O were added to 15.0 mL of deionized water (Si/Mg molar ratio = 2:1). The dosage ratios of MgSiO3/bentonite were 1:0, 30:1, 10:1, 1:1, and 0:1, respectively.
2.3. Adsorption experiments
Typically, 50.0 mg of adsorbent was added to 50.0 mL of methyl bromide solution in a thermostatic shaker (SHA-B) with a shaker speed of 120 rpm, a temperature of 25 °C, and an adsorption time of 3h. A 0.45 µm filter quickly separated the solution from the adsorbent. By calculating the absorbance of solution at its maximum wavelength of 664 nm using UV-Vis spectroscopy, the concentration of MB solution both before and after adsorption was examined. To study the MB adsorption isotherms, the concentrations of MB were made from 100 to 1500 mg L−1 (pH = 7). To assess the impact of pH values (the initial concentration of MB was controlled at 300 mg L−1) on the adsorption, the pH of MB solution was adjusted from 2 to 7. The following method was used to determine the adsorption kinetics. After being thoroughly contacted with 50 mg of adsorbent for 50 mL of the MB solution (pH = 7, starting concentrations ranging from 100 to 800 mg L−1), the mixture was filtered at various intervals (5–120 min for MB). By using the approach outlined above, it is possible to determine and compute the quantity of MB that the adsorbent will adsorb. The average results of three parallel runs of each experiment are provided.
2.4. Characterizations
In order to determine the phase composition of sample, an X-ray powder diffractometer (XRD) with a Cu-Kα radiation (MiniFlex600, Rigaku, Japan) was employed. The Fourier transform infrared (FTIR) spectrophotometer ranged from 4000 to 400 cm−1 to record the FTIR spectra (Thermo Scientific Nicolet iS20, Thermo Fisher, USA). SEM (ZEISS Gemini 300, Carl Zeiss AG, Germany) and its equipped energy dispersive spectrometer (EDS) have been used to characterize the morphology and quantitative elemental distribution in the samples. The Brunauer Emmett-Teller (BET) of the samples was measured by the N2 adsorption-desorption isotherms (ASAP 2020 HD88, Micrometrics, USA), and were calculated with the PS analyzer and SA. A UV–vis spectrophotometer was used to calculation the dye concentration by recording the absorbance of MB after adsorption (U-T5C, Shanghai Yipu Instrument Manufacturing Co., China).
3. Results and discussion
3.1. Structure and morphology analysis
In a typical preparation process, a facile one-pot hydrothermal approach for the construction of MS-Bt composite was developed by using Bt as structural promoter (). As shown in , the overall lamellar morphology of Bt was confirmed by SEM image, which is consistent with previous literatures [Citation20,Citation35]. After adding silicate, the surface of the composite is relatively smooth. Through a high-temperature hydrothermal treatment, microspheres of uniform size grow on the surface. The surface texture of the MS morphology without the addition of Bt hydrothermal synthesis is dense (Figure S1). Bt is known to be rich in Si, Al and O, with small amounts of Mg and Ca, so these elements can be seen on the elemental mapping (Figure S2a). Since MS is synthesized from sodium metasilicate and magnesium sulfate, the elements Si, Mg and O can be seen in the elemental mapping in Figures S2b and S3a. The STEM image of MS-Bt and the corresponding element mapping are shown in . The elements of Si, Mg, Al, O, Ca can be seen in their distribution profile. The presence of Si, Mg indicates the formation of magnesium silicate in the composites. And the presence of Al and Ca indicates that some Bt remained during the hydrothermal transformation process (Figure S3b). The intermediate product was further investigated by TEM characterization (Figure S4). It can be seen that there are sphere-like particles with sizes around 20 ∼ 50 nm in the lamellae. Next, it is further explored by HAADF-STEM and EDS elemental mapping. It is found that the major elements (such as Si, Mg, Al and O) distributed on the material, indicate that Mg2+ is adsorbed on the material before hydrothermal reaction [Citation36]. The appearing C element distribution shows a striped pattern, which is caused by the mapping of the substrate carbon support film.
3.2. XRD pattern and FT-IR spectrum analysis
As shown in , the XRD diffraction peaks of MS are indexed to the diffraction peaks of the Mg3Si4O10(OH)2 (JCPDS No. 13-0558, a = 5.287 Å, b = 9.158 Å and c = 18.950 Å) [Citation37]. The strength of the diffraction peaks is also comparatively weak, reflecting the low crystallinity of product. The XRD pattern of MS-Bt showed the crystalline form of analcime (NaAlSi2O6·H2O (JCPDS No. 41-1478)) in addition to the generation of well-crystallized MS [Citation38]. In SEM image, the analcime of MS-Bt is found with a clearly shaped structure. The magnification reveals that some of the analcime isn’t completely transformed, and have other substances attached to the surface (Figure S5). Then, an EDX elemental mapping of the analcime is done, and the findings are displayed in Figure S6. The characteristic elements correspond to Na, Al, Si, O. A small amount of Mg is present as adsorbed on the surface of the analcime. Bt contains mainly montmorillonite, and the rest of the square quartz and feldspar are also present.
To further verify the chemical composition and determine which the role of Bt, FT-IR spectral data are measured in the range from 4000 to 400 cm−1. As demonstrated by , bands at 3628, 3434, 1642, 1212, 659 and 464 cm−1 are in consistent with Mg3Si4O10(OH)2 [Citation39], firmly demonstrating the production of Lizardite. The peak at 3628 cm−1 is caused by -OH stretching vibrations in the octahedron. The stretching and bending vibrations of hydroxyl groups on the surface of the compounds are represented by the absorption bands at 3434 cm−1 and 1642 cm−1 [Citation39]. Si-O tetrahedron stretching vibrations can be ascribed to the bands at 1212 cm−1 [Citation40]. The bands at 659 cm−1 indicate the vibrations of -OH lattice and Mg-O out-of-plane. The shows that the characteristic peak of Bt still exists in the FT-IR spectra of MS-Bt, which indicates the main ingredient in bentonite, that is no change.
To gain more insight into the test samples, nitrogen adsorption-desorption analysis is used (). It is found that MS, MS-Bt and Bt all belong to type IV isotherms with irregular surface pore structures. MS and MS-Bt belong to H2 hysteresis lines of ink bottle pores, but the hysteresis curve of MS-Bt is more obvious. Bt belongs to the H3 type hysteresis loop, which indicates the irregular surface pore structure. The pore size (PS) contribution curves are originated from the desorption data. The PS distribution of MS belongs to the micropore region, which is mainly concentrated below 2 nm. While, the MS-Bt is concentrated between 1 and 5 nm, and there is a multi-level pore structure of micropores and mesopores. Bt structure intermediate pores and macropores dominate the PV. The samples’ pore structure characteristics are exhibited in Table S1, where the exterior SA and PV of MS-Bt are larger than those of MS and Bt at 255.60 m2 g−1 and 0.225 cm3 g−1, respectively. It is well known that the larger the specific surface area of the adsorbent the more corresponding adsorption sites. The porous structure in the adsorbent may allow for faster adsorption and transfer of molecules to the adsorbent [Citation41]. Therefore, MS-Bt has a huge SA and a matchless microporous-mesoporous structure, showing the potential application as an adsorbent, etc.
3.4. Effect of Bt, temperature and time on the hydrothermal products
3.4.1. Effect of Bt on the hydrothermal products
Different Bt additions, hydrothermal time and hydrothermal temperature affect the formation of MS-Bt. These variables are controlled separately, and the consequences of the specific influence study are exhibited in . shows the XRD pattern of the composites with different Bt addition ratios (0, 3.2%, 9.1%, 50% and 100%). illustrates the crystallinity of the distinctive peaks of the composites at 2θ = 19.94, 35.2 and 60.42°. With the increase of the Bt addition percentage, the peaks (JCPDS standard card 13-0558, Mg3Si4O10(OH)2) increase gradually. When the Bt content is 50%, those peaks are hidden and the Clinoptilolite and Cristobalite peaks in the Bt clearly appear (according to standard JCPDS data card numbers 25–1349 and 39–1425). In addition, characteristic peaks of analcime are found at 2θ = 15.97, 26.12 and 30.70° (according to standard JCPDS data card No. 41-1478), and its crystalline shape was more obvious.
Figure 4. Adsorption properties and XRD patterns of the products prepared by (a,b) different addition ratios of Bt, (c,d) different hydrothermal time and (e,f) different hydrothermal temperature.
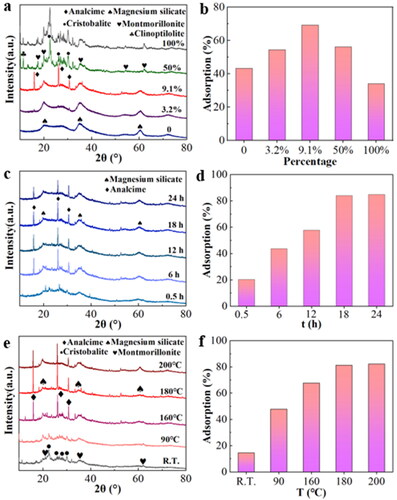
The presence of Bt is discovered to be necessary for the consistent morphology of MS-Bt in the usual procedure. The proportion of Bt in MS-Bt is altered from 0 to 100%. As shown in Figure S7a, the MS would possess few irregular particles through a hydrothermal process in absence of Bt, which should be mainly due to the tendency of silicate and magnesium ions to generate agglomerates during hydrothermal processes [Citation42]. Noticeably, it is noteworthy that with slight change of Bt feeding amount, the morphology of the product shows significant changes (Figure S7b). When the Bt content was 9.1%, microspheres of uneven size and some discontinuous irregular particles were formed (Figure S7c). Interestingly, as the Bt content increased to 50%, uniform microsphere particles were obtained, as shown earlier in Figure S7d. However, as the Bt content is further increased to 100%, the substrate’s lamellar structure is revealed (Figure S7e). It is clear that the presence and content of Bt is crucial for the hydrothermal establishment of consistent Mg3Si4O10(OH)2. Among these different Bt addition ratios, the removal of adsorbed MB peaked at 9.1%, and the adsorption rate of 69.28%, the adsorption effect is significantly better than MS and Bt alone samples (). Subsequently, the composites are prepared using 9.1% Bt addition ratio.
3.4.2. Effect of hydrothermal time on the hydrothermal products
As shown in , time-dependent tests are conducted to track the hydrothermal development of the mesoporous MS-Bt. At the beginning of the hydrothermal reaction, the Bt base is covered with non-crystalline silica, and the surface is relatively smooth. When treated for 0.5 h, the colloidal microspheres have disintegrated in part, and few low crystallinity Mg3Si4O10(OH)2 are formed (Figure S8a). After 6 h, the initially formed spherical Mg3Si4O10(OH)2 microstructures made of nanosheets appear alongside some colloidal microspheres with somewhat rough surfaces and some nanosheet fragments that have been randomly deposited on the surfaces (Figure S8b). As the time further increases to 24 h, the product of the microsphere morphology disappeared and generated a small number of vertical lamellar structures (Figure S8c). In addition, the material synthesized by hydrothermal for different times to adsorb MB for performance testing found that its adsorption reached its peak when the hydrothermal time was 18 h (). Last but not least, as previously stated, more and more porous Mg3Si4O10(OH)2 microspheres (higher crystallinity) are generated at the expense of colloidal microspheres when hydrothermally treated for a longer period of time, such as 18 h (Figure S8d). As seen in , the Al element in MS-Bt is shown in the sample profile, which indicates that the role of the doped Bt is the skeleton support.
3.4.3. Effect of hydrothermal-temperature on the hydrothermal products
Temperature-dependent experiments were conducted to track the growth of the MS-Bt, as shown in . With the composite temperature ranging from room temperature to 200 °C, the main products are Mg3Si4O10(OH)2, and the crystallinity of the products get higher and higher at the same time. However, the morphology varies substantially from one another. When the hydrothermal temperature is at R.T., silica is deposited in the Bt lamellar structure and Mg2+ are adsorbed on the surface (Figure S9a). Therefore, the surface shape of MS-Bt is smoother than that of Bt at this moment. When heating temperature at 90 °C, Mg3Si4O10(OH)2 microspheres agglomerate heavily and coexist with a considerable Bt (Figure S9b). At high temperature, alkaline conditions promote the formation of silicate-ion groups [Citation39]. increasing the temperature promotes the creation of silicate structures. A small amount of Mg3Si4O10(OH)2 microspheres are formed on the Bt sheet layer at 160 °C (Figure S9c). When hydrothermal treatment was performed at 180 °C, Mg3Si4O10(OH)2 microspheres of homogeneous size and distribution were produced as described above (Figure S9d). However, when the temperature was raised to 200 °C, some more compact and even hefty particles formed due to microsphere overgrowth at high temperatures (Figure S9e). At the same time, the hydrothermal synthesis of materials at different temperatures to adsorb MB performance test found that the hydrothermal temperature at 180 °C its best adsorption performance (). As a result, the proper temperature for the homogeneous mesoporous Mg3Si4O10(OH)2 microspheres is set as 180 °C.
3.5. Bt-assisted construction of magnesium-silicate-based MS-Bt composite
After stirring Na2SiO3·9H2O, MgSO4·7H2O and Bt into a suspension, amorphous SiO2 precipitated in the Bt voids at R.T. As the reaction temperature increased, the SiO2 was gradually corroded with OH− ions and reacted with Mg2+ to grow the Mg3Si4O10(OH)2. In addition, a small amount of Bt was converted to analcime. The present invention uses Bt as the growth skeleton to produce uniform Mg3Si4O10(OH)2 microspheres by a simple and easy hydrothermal synthesis method, which solves the phenomenon that magnesium silicate tends to agglomerate during hydrothermal growth.
3.6. MS-Bt as adsorbents for MB removal
The obtained adsorbents were used for the removal of cationic dyes MB from simulated wastewater, and the variables analyzed were the adsorption contact time, the beginning concentration of the contaminant, the pH of the solution, and reaction temperature (). represents the variation of the unit adsorption mass of the adsorbent for the adsorbed pollutant with the contact time. Bt is the first to reach adsorption equilibrium at 10 min, which is mainly due to its mainly macroporous structure that can adsorb pollutants in a short time and reach equilibrium quickly. MS-Bt and MS are mainly microporous structures that lead to delayed adsorption equilibrium time, but MS-Bt doped with Bt pore structure is mesoporous plus microporous structure, so it is better than MS in adsorption of pollutants. The final adsorption capacity of MS-Bt adsorbed MB was 213.16 mg g−1 within 180 min, which was higher than that of Bt and MS. depicts the influence of varied MB concentrations on the equilibrium adsorption capacity. The equilibrium capacity of those for their adsorption as increases as MB concentration. Bt was the first to attain adsorption equilibrium at 150 mg g−1. When the solubility was 300 mg g−1, MS-Bt and MS also reached the equilibrium, where the adsorption capacity of MS-Bt was the largest with 253.92 mg g−1. However, when the concentration reached 1000 mg g−1, all adsorbents displayed a decreasing trend. For the reason, the changes were inhibited by the pollutants in the high-concentration environment and the adsorption effect became poor.
Figure 5. Plot of adsorption amount with (a) adsorption time, (b) solution concentration, (c) solution pH and (d) reaction temperature.
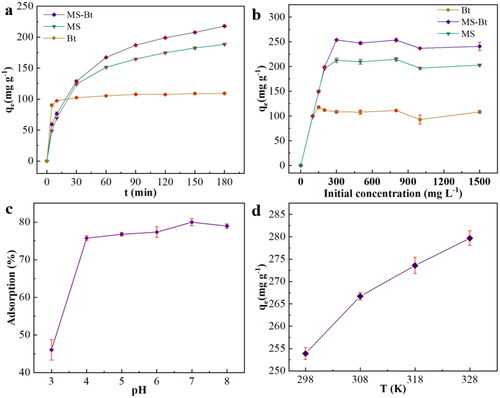
Since the pH can influence the surface charge, the effect of pH on the adsorption of MB by MS-Bt and the dependence of the removal rate of MB on pH is investigated in . The removal rate was only about 46% at an initial pH of 3. When the pH raised, the removal effect showed a step increase. This is because at this pH, the zeta potential of MS-Bt > 0 (Figure S10), which indicates that it is positively charged, while MB is a cationic pollutant, so the two are repelled by the same charge, which contributes to its low performance. When pH = 4 the zeta potential of MS-Bt is <0, at this moment the two are oppositely charged and the adsorption rate increases drastically.
The adsorption temperature was set to 25–55 °C. As can be seen from , the performance of MS-Bt increased gradually with the growing temperature, and the adsorption capacity increased by 10.14% at 55 °C compared with that at 25 °C. The unit adsorption capacity of MS-Bt at 55 °C was 279.68 mg g−1. In summary, it can be seen clearly that MS-Bt is affected by temperature during the adsorption of MB, and the whole adsorption is a spontaneous heat absorption process.
3.7. Adsorption model analysis
3.7.1. Adsorption isotherm analysis
The Langmuir and Freundlich model are both used to assess the equilibrium results from experiments for the adsorption of MB on the MS-Bt (). The adsorption experiments were calculated by the aforementioned two isotherm models. As shown in , the R2 = 0.9107 (Langmuir) > R2 = 0.9098 (Freundlich). It can be demonstrated that the Langmuir isotherm model can match the equilibrium experimental data more accurately than the Freundlich isotherm model from the perspective of the correlation coefficient. In other words, the MS-Bt surface, which shows monolayer adsorption and has a uniform distribution of adsorption sites, is where the majority of the adsorption takes place. On the other hand, the RL value fluctuates between 0 and 1, showing that the MS-Bt is successful to adsorb the organic dye. The Langmuir isotherm model was used to calculate the qm of MS-Bt for MB, which was determined to be 241.29 mg g−1.
Table 1. Corresponding fitting parameters for the adsorption of MB on the MS-Bt by using Langmuir and Freundlich isotherm models.
3.7.2 Adsorption kinetics analysis
As shown in , the pseudo-first-order (PFO) and pseudo-second-order (PSO) plots for MB adsorption on MS-Bt were fitted by using nonlinear regression of the experimental data prior to equilibration. The fitting results show that the PFO kinetics are not well fitted, and the qep differs from the qm (). Despite the fact that PFO has a superior R2, the process might not follow it. However, the PSO kinetic model is very well suited to describe the adsorption process of that (R2 = 0.998), and the qep is also reasonably close to the qm that was determined experimentally. Therefore, the pseudo-second-order kinetic equation is followed by the adsorption of MB by MS-Bt.
Table 2. Kinetic fitting parameters for the adsorption of MB on the MS-Bt by using PFO and PSO models.
3.7.3 Thermodynamic analysis
The slope and intercept of lnKd and 1/T after linear fitting yield ΔH and ΔS (Figure S11). The adsorption process ΔG at different temperatures is smaller than zero, as demonstrated in , illustrating that the adsorption process is self-motivated and easily adsorb MB. ΔG drops as temperature rises, signifying that is aided by the rise in temperature. Moreover, ΔH > 0 indicates that is endothermic, whereas a positive value of ΔS indicates that the disorder of the solid-solution system increases during MB adsorption [Citation43].
Table 3. Adsorption of MB onto sodium Bt: thermodynamic parameters.
shows the XRD patterns and FT-IR spectra of MS-Bt after adsorption. From , the diffraction peak of magnesium silicate becomes weaker after the adsorption of MB on MS-Bt, which may be the result of the MS-Bt surface after the adsorption of MB. The weakening of the absorption peaks at 3628, 3434, 659 and 464 cm−1 is due to the look-at interaction of the -OH attached to Si and Mg in the structure with the MB molecule () [Citation44]. After the adsorption of MB, new spectral bands appear at 1493, 1395 and 1340 cm−1, which are caused by the methylene group from MB, which had evidenced that the adsorption process have been on the surface of MS-Bt [Citation45].
The MS-Bt after the adsorption of MB was further investigated by EDS characterization. In Figure S12, the presence of N elements on MS-Bt after adsorption of MB can be found, which indicates that the adsorbent successfully adsorbed on MB. To assess the removal performance of MS- Bt, the qm values and removal rates for MB are matched with literature results, according to . As shown, the MS-Bt composites exhibit comparatively higher maximum adsorption capacity than that of most of the referenced adsorbents, such as the Sep-AAM, MgSiFM and Philippines Bt. In addition, the MS-Bt composites have the highest removal rate.
Table 4. Comparison of the removal rates and maximum adsorption for MB on different adsorbents.
4. Conclusion
In summary, uniform multilayer porous MS-Bt microspheres are successfully created using Bt as templates in a straightforward and environmentally friendly one-pot hydrothermal approach. The MS-Bt have a high SA of 392 m2 g−1, the PV of 0.22 cm3 g−1, and the PS of 2.29 nm. Based on the effects of hydrothermal conditions on the compounds, a skeleton-assisted production mechanism of Bt is proposed. The MS-Bt exhibits satisfactory qm of 253.92 mg g−1 when applied as adsorbents to remove the cationic dyes MB. Given the ease of preparation and environmental friendliness, the as-obtained MS-Bt is undeniably demonstrated as a remarkable competitive adsorbent alternative to remove MB.
Supplemental Material
Download MS Word (4.7 MB)Disclosure statement
No potential conflict of interest was reported by the author(s).
Data availability statement
The data that support the findings of this study are available from the corresponding author.
Additional information
Funding
Notes on contributors
Zhong Li
Zhong Li is a lecturer at the College of Chemistry and Chemical Engineering, Tarim University. The research field is Industrial adsorbents, industrial catalysts.
Feipeng Cheng
Feipeng Cheng is a master candidate at the College of Chemical Engineering, Beijing University of Chemical Technology. His research directions are the Clay mineral materials.
Yajun Li
Yajun Li is a master candidate at the College of Chemical Engineering, Beijing University of Chemical Technology. His research directions are the Clay mineral materials.
Dengfeng Wu
Dengfeng Wu is a lecturer at the College of Chemical Engineering, Beijing University of Chemical Technology. The research field is the Clay mineral materials.
Dongliang Yang
Dongliang Yang is a master candidate at the College of Chemical Engineering, Beijing University of Chemical Technology. His research directions are the Clay mineral materials.
Dan Sun
Dan Sun is a lecturer at the College of Chemistry and Chemical Engineering, Tarim University. The research field is molecular sieves and their applications.
Daojian Cheng
Daojian Cheng is a Chaired Professor Tarim University. He is interested in the design, preparation and application of metal nano catalysts in the chemical industry.
Weiliang Tian
Weiliang Tian is a Professor at the College of Chemistry and Chemical Engineering, Tarim University. The research field is nanocomposite materials.
References
- Han Y, Li H, Liu M, et al. Purification treatment of dyes wastewater with a novel micro-electrolysis reactor. Sep Purif Technol. 2016;170:1–16. doi: 10.1016/j.seppur.2016.06.058.
- Issabayeva G, Hang SY, Wong MC, et al. A review on the adsorption of phenols from wastewater onto diverse groups of adsorbents. Rev Chem Eng. 2018;34(6):855–873. doi: 10.1515/revce-2017-0007.
- Malakootian M, Heidari MR. Removal of phenol from steel wastewater by combined electrocoagulation with photo-Fenton. Water Sci Technol. 2018;78(5–6):1260–1267. doi: 10.2166/wst.2018.376.
- Vilar VJP, Moreira FC, Ferreira ACC, et al. Biodegradability enhancement of a pesticide-containing bio-treated wastewater using a solar photo-Fenton treatment step followed by a biological oxidation process. Water Res. 2012;46(15):4599–4613. doi: 10.1016/j.watres.2012.06.038.
- Al-Tohamy R, Ali SS, Li F, et al. A critical review on the treatment of dye-containing wastewater: ecotoxicological and health concerns of textile dyes and possible remediation approaches for environmental safety. Ecotoxicol Environ Saf. 2022;231:113160. doi: 10.1016/j.ecoenv.2021.113160.
- Tkaczyk A, Mitrowska K, Posyniak A. Synthetic organic dyes as contaminants of the aquatic environment and their implications for ecosystems: a review. Sci Total Environ. 2020;717:137222. doi: 10.1016/j.scitotenv.2020.137222.
- Pandey S, Makhado E, Kim S, et al. Recent developments of polysaccharide based superabsorbent nanocomposite for organic dye contamination removal from wastewater—a review. Environ Res. 2023;217:114909. doi: 10.1016/j.envres.2022.114909.
- Yang D, Cheng F, Chang L, et al. Sodium modification of low quality natural bentonite as enhanced lead ion adsorbent. Colloids Surf, A. 2022;651:129753. doi: 10.1016/j.colsurfa.2022.129753.
- Abdel-Moniem SM, El-Liethy MA, Ibrahim HS, et al. Innovative green/non-toxic Bi2S3@g-C3N4 nanosheets for dark antimicrobial activity and photocatalytic depollution: turnover assessment. Ecotoxicol Environ Saf. 2021;226:112808. doi: 10.1016/j.ecoenv.2021.112808.
- Li H, Xue S, Cao Y, et al. Photocatalytic reduction of Cr(VI) by WO3@PVP with elevated conduction band level and improved charge carrier separation property. Environ Sci Ecotechnol. 2020;3:100034. doi: 10.1016/j.ese.2020.100034.
- Saruchi, Kumar V, Kaith BS, Jindal, R. Synthesis of hybrid ion exchanger for rhodamine B dye removal: equilibrium, kinetic and thermodynamic studies. Ind Eng Chem Res. 2016;55(39):10492–10499. doi: 10.1021/acs.iecr.6b01690.
- Zhang F, Zhao Z, Tan R, et al. Selective and effective adsorption of methyl blue by barium phosphate nano-flake. J Colloid Interface Sci. 2012;386(1):277–284. doi: 10.1016/j.jcis.2012.07.034.
- Palas B, Ersöz G, Atalay S. Bioinspired metal oxide particles as efficient wet air oxidation and photocatalytic oxidation catalysts for the degradation of acetaminophen in aqueous phase. Ecotoxicol Environ Saf. 2019;182:109367. doi: 10.1016/j.ecoenv.2019.109367.
- Yadav KK, Kumar S, Pham QB, et al. Fluoride contamination, health problems and remediation methods in Asian groundwater: a comprehensive review. Ecotoxicol Environ Saf. 2019;182:109362. doi: 10.1016/j.ecoenv.2019.06.045.
- Ali SS, Al-Tohamy R, Sun J. Performance of Meyerozyma caribbica as a novel manganese peroxidase-producing yeast inhabiting wood-feeding termite gut symbionts for azo dye decolorization and detoxification. Sci Total Environ. 2022;806(Pt 3):150665. doi: 10.1016/j.scitotenv.2021.150665.
- Ali SS, Al-Tohamy R, Xie R, et al. Construction of a new lipase- and xylanase-producing oleaginous yeast consortium capable of reactive azo dye degradation and detoxification. Bioresour Technol. 2020;313:123631. doi: 10.1016/j.biortech.2020.123631.
- Behera M, Nayak J, Banerjee S, et al. A review on the treatment of textile industry waste effluents towards the development of efficient mitigation strategy: an integrated system design approach. J Environ Chem Eng. 2021;9(4):105277. doi: 10.1016/j.jece.2021.105277.
- Dasgupta J, Singh M, Sikder J, et al. Response surface-optimized removal of reactive red 120 dye from its aqueous solutions using polyethyleneimine enhanced ultrafiltration. Ecotoxicol Environ Saf. 2015;121:271–278. doi: 10.1016/j.ecoenv.2014.12.041.
- Samsami S, Mohamadizaniani M, Sarrafzadeh M-H, et al. Recent advances in the treatment of dye-containing wastewater from textile industries: overview and perspectives. Process Saf Environ Prot. 2020;143:138–163. doi: 10.1016/j.psep.2020.05.034.
- Zhang Z, Zhang H, Zhu L, et al. Hierarchical porous Ca(BO2)2 microspheres: hydrothermal–thermal conversion synthesis and their applications in heavy metal ions adsorption and solvent-free oxidation of benzyl alcohol. Chem Eng J. 2016;283:1273–1284. doi: 10.1016/j.cej.2015.08.073.
- Zhang X, You H, Hou J, et al. Green and scalable narrow-gap exfoliation of high-quality two-dimensional vermiculite nanosheets as poly (vinyl chloride) thermal stabilizers. J Mater Res Technol. 2023;24:3804–3814. doi: 10.1016/j.jmrt.2023.04.002.
- Lahori AH, Zhang Z, Shaheen SM, et al. Mono-and co-applications of Ca-bentonite with zeolite, Ca-hydroxide, and tobacco biochar affect phytoavailability and uptake of copper and lead in a gold mine-polluted soil. J Hazard Mater. 2019;374:401–411. doi: 10.1016/j.jhazmat.2019.04.057.
- Wei T, Zhou Y, Sun C, et al. An intermittent lithium deposition model based on CuMn-bimetallic MOF derivatives for composite lithium anode with ultrahigh areal capacity and current densities. Nano Res. 2023; doi: 10.1007/s12274-023-6187-8.
- Yu H, Zhu Y, Xu J, et al. Fabrication porous adsorbents templated from modified sepiolite-stabilized aqueous foams for high-efficient removal of cationic dyes. Chemosphere. 2020;259:126949. doi: 10.1016/j.chemosphere.2020.126949.
- Zhou S, Jin L, Gu P, et al. Novel calixarene-based porous organic polymers with superfast removal rate and ultrahigh adsorption capacity for selective separation of cationic dyes. Chem Eng J. 2022;433:134442. doi: 10.1016/j.cej.2021.134442.
- Mahmoodi NM, Masrouri O, Arabi AM. Synthesis of porous adsorbent using microwave assisted combustion method and dye removal. J Alloys Compd. 2014;602:210–220. doi: 10.1016/j.jallcom.2014.02.155.
- Yang D, Li Y, Zhao L, et al. Constructing ZIF-8-decorated montmorillonite composite with charge neutralization effect and pore structure optimization for enhanced Pb2+ capture from water. Chem Eng J. 2023;466:143014. doi: 10.1016/j.cej.2023.143014.
- Guo M, Yang G, Zhang S, et al. Co-modification of bentonite by CTAB and silane and its performance in Oil-Based drilling mud. Clays Clay Miner. 2020;68(6):646–655. doi: 10.1007/s42860-020-00093-7.
- Yaghmaeiyan N, Mirzaei M, Delghavi R. Montmorillonite clay: introduction and evaluation of its applications in different organic syntheses as catalyst: a review. Results Chem. 2022;4:100549. doi: 10.1016/j.rechem.2022.100549.
- Ewis D, Ba-Abbad MM, Benamor A, et al. Adsorption of organic water pollutants by clays and clay minerals composites: a comprehensive review. Appl Clay Sci. 2022;229:106686. doi: 10.1016/j.clay.2022.106686.
- Li Y, Yang D, Cheng F, et al. Regulating interlayer and surface properties of montmorillonite by dodecyl dimethyl betaine for enhanced lead ion capture. Surf Interfaces. 2023;42:103348. doi: 10.1016/j.surfin.2023.103348.
- Fu L, Li J, Wang G, et al. Adsorption behavior of organic pollutants on microplastics. Ecotoxicol Environ Saf. 2021;217:112207. doi: 10.1016/j.ecoenv.2021.112207.
- Cheng N, Wang B, Wu P, et al. Adsorption of emerging contaminants from water and wastewater by modified biochar: a review. Environ Pollut. 2021;273:116448. doi: 10.1016/j.envpol.2021.116448.
- Qu J, Meng Q, Peng W, et al. Application of functionalized biochar for adsorption of organic pollutants from environmental media: synthesis strategies, removal mechanisms and outlook. J Cleaner Prod. 2023;423:138690. doi: 10.1016/j.jclepro.2023.138690.
- Lan D, Zhu H, Zhang J, et al. Adsorptive removal of organic dyes via porous materials for wastewater treatment in recent decades: a review on species, mechanisms and perspectives. Chemosphere. 2022;293:133464. doi: 10.1016/j.chemosphere.2021.133464.
- Sun P, Xu L, Li J, et al. Hydrothermal synthesis of mesoporous Mg3Si2O5(OH)4 microspheres as high-performance adsorbents for dye removal. Chem Eng J. 2018;334:377–388. doi: 10.1016/j.cej.2017.09.120.
- Sun P, Xu L, Jiang X, et al. Facile and green one-pot hydrothermal formation of hierarchical porous magnesium silicate microspheres as excellent adsorbents for anionic organic dye removal. Ind Eng Chem Res. 2019;58(8):2945–2957. doi: 10.1021/acs.iecr.8b04841.
- Jin Y, Liu Z, Han L, et al. Synthesis of coal-analcime composite from coal gangue and its adsorption performance on heavy metal ions. J Hazard Mater. 2022;423:127027. doi: 10.1016/j.jhazmat.2021.127027.
- Hegyesi N, Simon N, Pukánszky B. Silane modification of layered silicates and the mechanism of network formation from exfoliated layers. Appl Clay Sci. 2019;171:74–81. doi: 10.1016/j.clay.2019.01.023.
- Cho E-B, Mandal M, Jaroniec M. Periodic mesoporous benzene − silicas prepared using boric acid as catalyst. Chem Mater. 2011;23(7):1971–1976. doi: 10.1021/cm200166f.
- Ding S, Liu N, Li X, et al. Silica nanotubes and their assembly assisted by boric acid to hierarchical mesostructures. Langmuir. 2010;26(7):4572–4575. doi: 10.1021/la904851r.
- Huang R, Wu M, Zhang T, et al. Template-free synthesis of large-pore-size porous magnesium silicate hierarchical nanostructures for high-Efficiency removal of heavy metal ions. ACS Sustainable Chem Eng. 2017;5(3):2774–2780. doi: 10.1021/acssuschemeng.7b00140.
- Mahmoodi NM, Taghizadeh M, Taghizadeh A. Mesoporous activated carbons of low-cost agricultural bio-wastes with high adsorption capacity: preparation and artificial neural network modeling of dye removal from single and multicomponent (binary and ternary) systems. J Mol Liq. 2018;269:217–228. doi: 10.1016/j.molliq.2018.07.108.
- Wang W, Tian G, Zhang Z, et al. A simple hydrothermal approach to modify palygorskite for high-efficient adsorption of methylene blue and Cu(II) ions. Chem Eng J. 2015;265:228–238. doi: 10.1016/j.cej.2014.11.135.
- İşçi S. Intercalation of vermiculite in presence of surfactants. Appl Clay Sci. 2017;146:7–13. doi: 10.1016/j.clay.2017.05.030.
- Kaya-Özkiper K, Uzun A, Soyer-Uzun S. A novel alkali activated magnesium silicate as an effective and mechanically strong adsorbent for methylene blue removal. J Hazard Mater. 2022;424(Pt A):127256. doi: 10.1016/j.jhazmat.2021.127256.
- Zhang J, Dang L, Zhang M, et al. Characterization of mesoporous magnesium silicate with hierarchical structure and its adsorption performance for dye and lead ion. Surf Interfaces. 2017;8:112–118. doi: 10.1016/j.surfin.2017.05.005.
- Huang R, He L, Zhang T, et al. Fabrication and adsorption behavior of magnesium silicate hydrate nanoparticles towards methylene blue. Nanomaterials. 2018;8(5):271. doi: 10.3390/nano8050271.
- Que A, Zhu T, Zheng Y. Highly efficient removal of methylene blue via hollow graphene-based magnesium silicate. J Mater Sci. 2021;56(29):16351–16361. doi: 10.1007/s10853-021-06299-x.
- Zhao R, Li Y, Sun B, et al. Highly flexible magnesium silicate nanofibrous membranes for effective removal of methylene blue from aqueous solution. Chem Eng J. 2019;359:1603–1616. doi: 10.1016/j.cej.2018.11.011.
- Gui C-X, Wang Q-Q, Hao S-M, et al. Sandwichlike magnesium silicate/reduced graphene oxide nanocomposite for enhanced Pb2+ and methylene blue adsorption. ACS Appl Mater Interfaces. 2014;6(16):14653–14659. doi: 10.1021/am503997e.
- Sun Z, Srinivasakannan C, Liang J, et al. Preparation of hierarchical magnesium silicate with excellent adsorption capacity. Ceram Int. 2019;45(4):4590–4595. doi: 10.1016/j.ceramint.2018.11.146.
- Wang Y, López-Valdivieso A, Zhang T, et al. Preparation of microscale zero-valent iron-fly ash-bentonite composite and evaluation of its adsorption performance of crystal violet and methylene blue dyes. Environ Sci Pollut Res Int. 2017;24(24):20050–20062. doi: 10.1007/s11356-017-9426-2.
- De Castro MLFA, Abad MLB, Sumalinog DAG, et al. Adsorption of methylene blue dye and Cu(II) ions on EDTA-modified bentonite: isotherm, kinetic and thermodynamic studies. Sustainable Environ Res. 2018;28(5):197–205. doi: 10.1016/j.serj.2018.04.001.