ABSTRACT
Microorganisms confined to annual sea ice in the Southern Ocean are exposed to highly variable oxygen and carbonate chemistry dynamics because of the seasonal increase in biomass and limited exchange with the underlying water column. For sea-ice algae, physiological stress is likely to be exacerbated when the ice melts; however, variation in carbonate speciation has rarely been monitored during this important state-transition. Using pulse amplitude modulated fluorometry (Imaging-PAM, Walz), we documented in situ changes in the maximum quantum yield of photosystem II (Fv/Fm) of sea-ice algae melting out into seawater with initial pH values ranging from 7.66 to 6.39. Although the process of ice-melt elevated seawater pH by 0.2–0.55 units, we observed a decrease in Fv/Fm between 0.02 and 0.06 for each unit drop in pH during real-time fluorescence imaging. These results are considered preliminary but provide context for including carbonate chemistry monitoring in the design of future sea ice state-transition experiments. Imaging-PAM is a reliable technology for determining Fv/Fm, but is of limited use for obtaining additional photosynthetic parameters when imaging melting ice.
Introduction
High-latitude waters of the Southern Ocean will be among the first places on Earth to experience the effects of anthropogenic-induced climate change, including ocean warming and acidification (Fabry et al. Citation2008; Doney Citation2010). In the McMurdo Sound region of Antarctica, the mean seawater pH is currently 8.05, with a seasonal range of 0.42 units. By 2100 pH is predicted to drop below 7.9 and exhibit greater annual variability (Kapsenberg et al. Citation2015). The associated change in seawater carbonate speciation (increased CO2, HCO3−, H+; decreased CO32−) and its effect on polar ocean primary production are not fully resolved. Shelf waters surrounding Antarctica are effective sinks for anthropogenic carbon emissions, and increased oceanic CO2 availability could facilitate faster rates of photosynthesis and growth in marine photoautotrophs (Riebesell et al. Citation1993). However, dissolved inorganic carbon is rarely in short supply in planktonic ecosystems and many taxa, including diatoms, possess carbon-concentrating-mechanisms that are highly efficient at elevating CO2 at the site of RuBisCO (Reinfelder Citation2011; Kranz et al. Citation2015). To date, incubation experiments with Southern Ocean phytoplankton have demonstrated a variable response to elevated CO2 (Müller et al. Citation2015). Some phytoplankton down-regulate carbon-concentrating mechanisms, a response that potentially liberates energy that can be reallocated to other functions (Raven et al. Citation2011), but this does not always equate to increased growth rates and primary production (Torstensson et al. Citation2012; Trimborn et al. Citation2013; McMinn et al. Citation2014; Young et al. Citation2015).
Figure 1. Location and schematic representation of sampling site at Cape Evans, McMurdo Sound, Antarctica. The image on the right shows a typical ice sample melting into manipulated seawater. The ice has been imaged using minimal fluorescence, Fo, with an I-PAM. The circles represent regions of relatively high algal biomass.
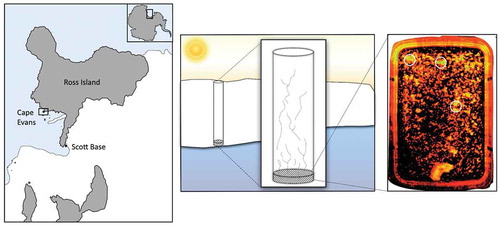
Figure 2. Linear third-order polynomial model of maximum quantum yield (Fv/Fm) for each pH treatment and the control contrasting the difference between start and end-point measurements. The error variance is represented by the dark grey band.
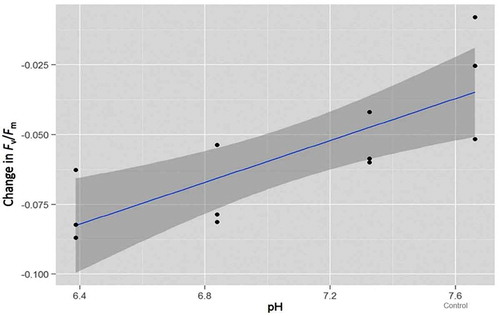
For microbes confined to annual sea ice in the Southern Ocean the situation is significantly different. Because of the seasonal increase in biomass and limited exchange with the underlying water column, there are a number of dramatic chemical changes that take place within the ice matrix, specifically variable CO2, O2 and pH dynamics (Thomas & Dieckmann Citation2002; Thomas & Papadimitriou Citation2003). Efficient physiological acclimation that sustains photosynthesis within the ice is therefore critically important (Arrigo & Thomas Citation2004; McMinn et al. Citation2005), but the influence of pH on algal physiology is not clearly understood. Experimental work with cells growing near the upper surface of the ice demonstrates that increased CO2 enhances growth by approximately 20%, provided that cells are incubated at a constant pH of ca. 8.0 (McMinn et al. Citation2014). If microbes are exposed to decreasing pH (< ca. 7.2), then the increase in H+ ions has been shown to reduce growth rates by almost 50% (McMinn et al. Citation2014).
A general understanding of how sea ice impacts the carbon cycle and ocean–atmosphere CO2 exchange in polar regions is now emerging (see Rysgaard et al. Citation2011; Fransson et al. Citation2015), but the associated changes in carbonate chemistry due to melting ice remain poorly characterized (Bates et al. Citation2014). The bottom-ice algae that dominate the biological assemblages in Antarctic fast-ice undergo considerable physiological stress during the melt process. This ecological state-transition has been well studied with respect to UV-B and PAR (e.g., Palmisano & Sullivan Citation1983; Mock & Kroon Citation2002; McMinn et al. Citation2010; Ryan et al. Citation2012), temperature (e.g., Arrigo & Thomas Citation2004; Mock & Thomas Citation2005; Ralph et al. Citation2005) and salinity (e.g., Arrigo & Sullivan Citation1992; Ralph et al. Citation2007; Ryan et al. Citation2011; Ugalde et al. Citation2014), but not to associated changes in pH. The evolution of distinct pH environments within sea ice has only recently been described by utilizing an experimental outdoor facility (Hare et al. Citation2013). Analysis of vertical pH profiles revealed a consistent C-shaped pattern with highest pH values (>9) in both top and bottom sections of the ice and lowest pH (ca. 7) in interior ice sections, albeit in the absence of biological activity. In spring and summer, microbial photosynthetic activity depletes dissolved CO2 and pH rises. However, it is not known how variation in pH influences algal photophysiology with respect to freeze–thaw dynamics.
A particularly useful tool for correlating algal photophysiology with changing environmental conditions has been pulse amplitude modulated fluorometry. This technique is based on measuring variable chlorophyll a fluorescence, which provides a unique indicator of intracellular activity in photoautotrophs (Schreiber Citation2004; Ralph & Gademan Citation2005; Baker Citation2008). McMinn et al. (Citation2003) pioneered the use of pulse amplitude modulated fluorometry in Antarctica, and various configurations of the technology have now been employed for the in vitro analysis of ice shavings, extracted brines and melted ice cores (e.g., Ryan et al. Citation2004; Ralph et al. Citation2007; Kennedy et al. Citation2012; Martin et al. Citation2012). I-PAM (Walz, Germany) provides unprecedented two-dimensional in situ imagery which allows physiological stress to be measured with significantly less disruption of the physical habitat (Ryan et al. Citation2011; Hawes et al. Citation2012; Lund-Hansen et al. Citation2014). This technology has only been employed once in Antarctica and with mixed success (Ryan et al. Citation2011), but the capacity to generate in situ data warrants further investigation. In this preliminary study, our objectives were to expose sea-ice algae to changes in pH to determine whether short-term (8-h) exposure to reduced pH influences algal photophysiology (Experiment 1) and to quantify changes in pH that occur during the melt process (Experiment 2). We also determine whether imaging fluorescence is a viable technology to resolve changes in algal photophysiology during state-transition experiments.
Materials and methods
Study area
Bottom-ice algae were collected from 1.8 m thick annual fast-ice at Cape Evans, McMurdo Sound, Antarctica (77°38ʹS, 166°24ʹE), between 27 November and 1 December 2013 (). Limited incubation space in the field laboratory required that the experiment be carried out over four days; one day per pH treatment and one day for the control. The order of the treatments and control was selected at random to minimize any effect of in situ change in the microbial community. Two ice cores were required each day and these were obtained by drilling to within 300 mm of the ice/water interface using a powered ice auger (Jiffy), and then using a Mark V corer (Kovacs) to extract the last section of ice from the bottom of each hole. Once clear of the ice, each core was immediately transferred to a black polythene bag to protect the algae from light shock and transported a short distance to the field laboratory inside a cool box.
Experimental set-up
Each day, the two extracted ice cores were cut, under minimal light, into three pieces 40 mm × 40 mm × 20 mm thick to include bottom-ice algae. The six blocks of ice were then dark-adapted for 30 min in a cool box maintained at about 0°C before being placed into individual airtight plastic containers with clear plastic covers containing 112 ml of seawater (filtered to 0.7 µm, −1.8°C). The seawater had previously been collected from a local dive hole. Seawater pH was reduced by adding HCl which changed the total alkalinity (AT) while keeping the dissolved inorganic carbon (CT) concentration relatively constant. Untreated seawater was used as a control treatment (pH 7.66, pCO2 1651 μatm). The three pH treatments ranged from 7.32 to 6.39 (free scale). During the daily incubation, the six plastic containers containing ice core samples were placed in a temperature controlled water bath at 0.5°C ± 0.2°C for 480 min. An artificial light source comprising a 3 × 4 array of LED lamps suspended 800 mm above the water bath was used to provide an incubation irradiance of 40 μmol photons m−2 s−1. Three of the plastic containers were periodically opened in order to assess algal photophysiology (Experiment 1). Constant agitation of the seawater inside the containers (generated by circulation of the liquid in the water bath) ensured that the ice was bathed in seawater and prevented stratification as the ice melted. The other three containers, also exposed to light, remained closed for the duration of the experiment to examine the change in carbonate chemistry due to the melt process and biological activity (Experiment 2).
Experiment 1: photophysiology
Variable chlorophyll fluorescence was measured using the Maxi version of the I-PAM instrument (Walz). The I-PAM Maxi can resolve the distribution of chlorophyll a and activity of photosystem II in two dimensions over an area of 130 mm × 100 mm and the user has the option of employing pulse-modulated excitation for the generation of rapid light curves, actinic light and saturation pulses. The blue excitation light supplied by the I-PAM is centred on 450 nm, which is a wavelength that is characteristic of the blue-green spectra at the bottom of the ice (Lund-Hansen et al. Citation2014). The saturation pulse method was used to determine minimum (Fo) and maximum (Fm) fluorescent yields (Fv/Fm) of the dark-adapted ice samples. The I-PAM operational software includes an area-of-interest function that was used to select three specific locations on each block of ice to obtain Fv/Fm measurements. Selecting locations on each block of ice was a semi-random process; data were only acquired from areas-of-interest where Fo was >0.15. Fo is a measure of minimum fluorescence intensity of algae in the dark-adapted state where intensity is proportional to biomass. Fluorescent measurements of the blocks of ice were made by first removing the lid and then carefully aligning each plastic container on the stage of the I-PAM by using the live near-infrared imaging feature. After obtaining fluorescence measurements the lid was placed back on the container and immediately returned to the water bath. This process was performed in the dark in a field laboratory maintained at ca. 4°C and took no more than 30 s per sample. An initial assessment of Fv/Fm was made before the ice was added to the containers and then at 30-min intervals up to 120 min. Thereafter, fluorescence imaging was carried out at 240, 360 and 480 min. Samples were dark-adapted in the water bath for 10 min prior to fluorescence analysis (10–15 min is typical for dark-adapting sea ice algae (Ralph et al. Citation2007; McMinn et al. Citation2010)) by turning off the overhead light array. The generation of rapid light curves was attempted, but like Ryan et al. (Citation2011), we could not achieve the required saturation to derive the necessary parameters.
Experiment 2: carbonate chemistry
Samples for CT and AT were taken from replicates of the melted ice at the end of each experimental run. CT samples were filtered through 0.2 μm sterile filters into 12.5 ml Exetainers (Labco), poisoned with HgCl2 and sealed air tight. AT samples were filtered through GF/F filters, poisoned with HgCl2 and stored in 250 ml polythene flasks. The programme CO2SYS version 1.05 (Lewis & Wallace Citation1998) was used to calculate the carbonate system using the dissociation constants for carbonic acid (Roy et al. Citation1993). CT and AT samples were analysed as the mean of triplicate measurements with the infrared detection method using a dissolved inorganic carbon analyser (Apollo SciTech, model AS-C3) and the potentiometric titration method for AT. Data were corrected to Certified Reference Materials (Scripps Institution of Oceanography, USA). Consecutive measurements of the Dickson standard resulted in an average precision of >98% for both CT and AT.
Ice algal species composition
A 10 ml sample of three randomly selected core sections was fixed in 0.5% gluteraldehyde. A 0.5 ml aliquot from each sample was prepared using a special settling chamber and cells were examined using a Zeiss compound microscope with standard optics. Microalgae were counted from 10 randomly selected fields of view.
Data analysis
To examine potential changes in maximum quantum yield (Fv/Fm) relative to pH and incubation period, a linear third-order polynomial model was fitted to the data using the statistical package RStudio (version 0.98.939; RStudio Team).
Results and discussion
This is the first study to employ chlorophyll imaging fluorometry to examine the effect of decreased pH on algal photophysiology. Experiments were conducted in a remote Antarctic field camp with limited facilities to replicate a precisely controlled laboratory environment. A broad initial pH regime was chosen (6.39–7.66) to allow for shifts in carbonate chemistry induced by outgassing and experimental handling procedures, specifically the removal of plastic lids to perform I-PAM measurements, which exposed ice samples to the atmosphere (3.5 min during the 8-h incubation, Experiment 1).
The sea ice at Cape Evans in McMurdo Sound was 1.8 m thick in November–December 2013, with no snow cover. The bottom-ice community was dominated by the pennate diatom Nitzschia stellata, which accounted for >90% of the observed cells. Other taxa present within the ice included Fragilariopsis spp., Gyrosigma sp., Navicula directa and N. glaciei.
Photophysiology
The ratio Fv/Fm derived using the saturation pulse method is a sensitive indicator of photosynthetic potential in photoautotrophs. Prior to incubation, Fv/Fm of the sea-ice algae ranged from 0.37 to 0.52, which is indicative of a relatively healthy community that was well-acclimated to the light climate within the ice matrix (). At the incubation temperature of 0.5°C, it took approximately two hours for the ice to completely melt, during which time the ice was imaged four times. The post-melt Fv/Fm values obtained at 240, 360 and 480 min were derived from algae that were suspended in seawater (). The maximum quantum yield of photosystem II declined in all treatments and the control during the period of ice-melt (). This response is indicative of the physiological stress associated with both the transition from semi-solid ice to liquid water and exposure to light. A similar response was documented by Ryan et al. (Citation2011) when sea-ice algae were first examined using imaging fluorescence. These authors also identified salinity to be a significant co-stressor, particularly at concentrations <24. To prevent saline shock in the current study, we used smaller blocks of ice. As a result, the salinity only decreased from 34 to ca. 28 by the end of the experiment () and any effect on Fv/Fm is considered to be minimal. To determine whether pH also influenced the photosynthetic yield measurements, we fitted a linear third-order polynomial model to the fluorescence data. This model accounted for the variation in initial Fv/Fm that was evident in the pH 7.66 treatment (). By retaining only the first order term in the model, the analysis revealed that for each unit drop in pH, the change in yield of photosystem II decreased over time by between 0.02 and 0.06 (t (10) = 4.06, r2 = 0.623, p < 0.01; ).
Table 1. Response of sea-ice algae exposed to variable pH during ice-melt. Maximum quantum yield (Fv/Fm) was determined using imaging fluorescence (I-PAM). Each value is the mean with standard error in parentheses, n = 3.
Table 2. Carbonate chemistry of CO2 manipulation experiment utilizing bottom-ice algae at Cape Evan, 2013. The end of experiment data (8-h incubation) illustrates the changes in carbonate chemistry associated with ice-melt. The unit for CT, AT and CO2 is μmol kg−1. The unit for pCO2 is μatm.
Experimental manipulation of pH has two main effects on marine photoautotrophs, specifically acidification (increased H+) and carbonation (elevated CO2). Relative to ambient oceanic conditions, the former generally has a negative effect on algal physiology, the latter a positive effect. This results in an optimum curve with respect to the physiological response in marine microbes (see Bach et al. Citation2015). McMinn et al. (Citation2014) were the first to examine how algae released from the ice matrix respond to manipulated carbonate chemistry, and while a decline in Fv/Fm was observed in brine algae exposed to a pH regime that varied from 8.66 to 7.19, the trend was not significant. More recently, Coad et al. (Citation2016) melted algae from the snow/ice interface region of annual pack-ice into a pH regime that varied from 7.82 to 7.0, but only modest impacts on photophysiology were documented after seven days. Because of this non-response in photosynthetic parameters, McMinn et al. (Citation2017) have questioned the use of pulse amplitude modulated fluorometry to measure stress response in pH/CO2 experiments. In this study, we adopted a more acidic regime and did observe a significant response to reduced pH. However, the relationship between H+ concentration and Fv/Fm has yet to be substantiated. Previous studies that have included growth rates (e.g., McMinn et al. Citation2014; McMinn et al. Citation2017) have shown that a decline in growth is attributed to high H+ concentration rather than high CO2. This suggests that H+ concentration can influence intracellular pH and/or changes in membrane potential, energy partitioning and enzyme activity (Beardall & Raven Citation2004; Suffrian et al. Citation2011), and this has a subsequent effect on photosynthetic performance. Although the decline in photosynthetic potential observed during ice-melt was most pronounced in cells exposed to the lowest pH (6.39), Fv/Fm was consistently higher compared to the less acidic treatments and the control (). This highlights the fact that a significant increase in H+ ions has a minimal short-term effect on Fv/Fm, as assessed using an I-PAM. The relative change in Fv/Fm described by the model must be considered a subtle trend, but it is potentially indicative of a more significant stress response had the experiment been conducted over a longer time period. Importantly, we did not observe a threshold at which point algal photophysiology is compromised to the extent observed when other stressors such as light (Ralph et al. Citation2007; Ryan et al. Citation2009; Ryan et al. Citation2011), temperature (Ralph et al. Citation2005; Rajanahally et al. Citation2014) or salinity (Ryan et al. Citation2004; Ralph et al. Citation2007; Ryan et al. Citation2011) are manipulated.
Carbonate chemistry
The aim of Experiment 2 was to provide a rudimentary insight into the changes in pH that occur as the sea ice melts. The post-incubation carbon chemistry data () was obtained from plastic containers that were not opened during the incubation period and for that reason the data are not directly comparable with the photophysiology experiment. By the end of the incubation period, ice-melt and the biological activity of liberated microbes had increased the seawater pH in all treatments and the control, from 0.2 to 0.55 units. This provides a very basic illustration of the dynamic that occurs at the receding ice edge which has recently been described at an ecologically relevant scale in the Arctic Ocean as part of the NASA ICESCAPE project (Bates et al. Citation2014). The carbonate chemistry of below-ice interface melt waters at 19 ice stations was variable and difficult to predict, but generally exhibited higher pH and lower pCO2 compared to the co-located mixed layer beneath. Although we collected ice cores from a discrete area of sea ice, there was significant variation in post-melt measurements of CT and AT, presumably due to variability in biomass and the ratio of ice to brine volume in each block of ice. However, algal cellular physiology is not directly affected by variation in CT and AT; of significance is the change in the total concentration of ions (CO2, HCO3, CO3 and H+) that are made available.
In situ fluorescence imaging
The practice of actively releasing cells from the ice matrix is likely to modify or damage cellular physiology and ice-associated microbes should ideally be studied in situ. While this has been successfully achieved using oxygen electrodes (e.g., Kühl et al. Citation2001; Trenerry et al. Citation2002; McMinn et al. Citation2012) and habitat modification experiments (Martin et al. Citation2011), options for physiology experiments are limited. In situ fluorescence imaging is of significant interest because algal photophysiology can be assessed at a spatially relevant scale, specifically the assessment of cells inside the microscopic brine channels that characterise the ice matrix. Although our measurements are only indicative of ablation at the receding ice edge in the austral summer, real-time measurements are considered important because this state-transition, and exposure to physico-chemical stress, occurs relatively quickly. Like Ryan et al. (Citation2011), we could not use the I-PAM to generate rapid light curves and obtain the additional photosynthetic parameters rETRmax, alpha, and Ek. The maximum rate of electron flow from photosystem II to photosystem I (ETRmax) is of particular interest because it describes photosynthetic capacity. The initial ice dimensions used by Ryan et al. (Citation2011) were 70 mm × 60 mm × 15 mm and we reduced this to 40 mm × 40 mm × 20 mm (i.e., a 50% reduction in volume). However, the relative abundance of algae within the ice was still too high. The rapid light curves do not reach an asymptote because multiple layers of densely packed cells confound the required response to the increasing irradiance emitted by the fluorometer. To an extent, this problem has now been addressed by Hawes et al. (Citation2012), who successfully generated rapid light curves RLCs during fluorescence imaging of newly forming Arctic sea ice with a relatively low biomass. These authors used the I-PAM Mini (Walz), which has a reduced imaging area of 30 × 23 mm, and integrated the fluorescence data obtained from a series of ice core sections that were 2 mm thick. Working with such thin sections is not a practical solution in state-transition experiments because of rapid ice-melt. For this reason we suggest the I-PAM Maxi is only relevant for collecting in situ measurements of maximum quantum yield (Fv/Fm).
Conclusion
This is the first study to document statistically significant photophysiological stress in sea-ice algae due to decreased pH. Future experiments will require greater experimental precision, but the data provide context for assessing the relative importance of carbonate chemistry to sea-ice ecophysiology. Importantly, the physiological stress associated with rapid exposure to high light and low salinity during ice-melt is significant and the compound stress associated with the projected decline in pH in Antarctic coastal waters has the potential to shift environmental conditions beyond physiologically tolerable levels (McMinn et al. Citation2014). A mechanistic understanding of how ice-associated microbes adapt to freeze–thaw dynamics requires a clearer insight into how pH influences algal physiology. For this reason, we suggest that carbonate chemistry monitoring should be included in the design of future multi-stressor state-transition experiments.
Acknowledgements
We thank Antarctica New Zealand for logistic support and the staff at Scott Base for their help in preparation and deployment to the field.
Disclosure statement
No potential conflict of interest was reported by the authors.
Additional information
Funding
References
- Arrigo K.R. & Sullivan C.W. 1992. The influence of salinity and temperature covariation on the photophysiological characteristics of Antarctic sea ice microalgae. Journal of Phycology 28, 746–8.
- Arrigo K.R. & Thomas D.N. 2004. Large scale importance of sea ice biology in the Southern Ocean. Antarctic Science 16, 471–486.
- Bach L.T., Riebesell U., Gutowska M.A., Federwisch L. & Schulz K.G. 2015. A unifying concept of coccolithophore sensitivity to changing carbonate chemistry embedded in an ecological framework. Progress in Oceanography 135, 125–138.
- Baker N.R. 2008. Chlorophyll fluorescence: a probe of photosynthesis in vivo. Annual Review of Plant Biology 59, 89–113.
- Bates N.R., Garley R., Frey K.E., Shake K.L. & Mathis J.T. 2014. Sea–ice melt CO2-carbonate chemistry in the western Arctic Ocean: meltwater contributions to air–sea CO2 gas exchange, mixed-layer properties and rates of net community production under sea ice. Biogeosciences 11, 6769–6789.
- Beardall J. & Raven J.A. 2004. The potential effects of global climate change on microalgal photosynthesis, growth and ecology. Phycologia 43, 26–40.
- Coad T., Nomura D., McMinn A. & Martin A. 2016. Effect of elevated CO2 concentration on the photophysiology and growth of microalgae in Antarctic pack ice communities. Deep-Sea Research Part II Topical Studies in Oceanography 131, 160–169.
- Doney S.C. 2010. The growing human footprint on coastal and open-ocean biogeochemistry. Science 328, 1512–1516.
- Fabry V.J., Seibel B.A., Feely R.A. & Orr J.C. 2008. Impacts of ocean acidification on marine fauna and ecosystem processes. Journal of Marine Science 65, 414–432.
- Fransson A., Chierici M., Nomura D., Granskog M.A., Kristiansen S., Martma T. & Nehrke G. 2015. Effect of glacial drainage water on the CO2 system and ocean acidification state in an Arctic tidewater-glacier fjord during two contrasting years. Journal of Geophysical Research—Oceans 120, 2413–2429.
- Hare A.A., Wang F., Barber D., Geilfus N.-X., Galley R.J. & Rysgaard S. 2013. pH evolution in sea ice grown at an outdoor experimental facility. Marine Chemistry 154, 46–54.
- Hawes I., Lund-Hansen L.C., Sorrell B.K., Nielson M.H., Borzák R. & Buss I. 2012. Photobiology of sea ice algae during initial spring growth in Kangerlussuaq, west Greenland: insights from imaging variable chlorophyll fluorescence of ice cores. Photosynthesis Research 112, 103–115.
- Kapsenberg L., Kelley A.L., Shaw E.C., Martz T.R. & Hofmann G.E. 2015. Near-shore Antarctic pH variability has implications for the design of ocean acidification experiments. Scientific Reports 5, article no. 10497, doi: 10.1038/srep10497.
- Kennedy F., McMinn A. & Martin A. 2012. Effect of temperature and sea ice on the photosynthetic efficiency and morphotype of Phaeocystis antarctica. Journal of Experimental Marine Biology and Ecology 429, 7–14.
- Kranz S.A., Young J.N., Hopkinson B., Goldman J.A.L., Tortell P.D. & Morel F.M.M. 2015. Low temperature reduces the energetic requirement for the CO2 concentrating mechanism in diatoms. The New Phytologist 205, 192–201.
- Kühl M., Glud R.N., Borum J., Roberts R. & Rysgaard S. 2001. Photosynthetic performance of surface-associated algae below sea ice as measured with a pulse-amplitude-modulated (PAM) fluorometer and O2 microsensors. Marine Ecology Progress Series 223, 1–14.
- Lewis E. & Wallace D.W.R. 1998. Program developed for CO2 system calculations. ORNL/CDIAC-105. Oak Ridge, TN: Carbon Dioxide Information Analysis Center, Oak Ridge National Laboratory, US Department of Energy.
- Lund-Hansen L.C., Hawes I., Sorrell B.K. & Nielsen M.H. 2014. Removal of snow cover inhibits spring growth of Arctic ice algae through physiological and behavioural effects. Polar Biology 37, 471–481.
- Martin A., Anderson M.J., Thorn C., Davy S.K. & Ryan K.G. 2011. Response of sea-ice microbial communities to environmental disturbance: an in situ transplant experiment in the Antarctic. Marine Ecology Progress Series 424, 25–37.
- Martin A., McMinn A., Heath M., Hegseth E. & Ryan K.G. 2012. The physiological response to increased temperature in over-wintering sea ice algae and phytoplankton in McMurdo Sound, Antarctica and Tromsø Sound, Norway. Journal of Experimental Marine Biology and Ecology 428, 57–66.
- McMinn A., Ashworth C., Bhagooli R., Martin A., Salleh S., Ralph P. & Ryan K.G. 2012. Antarctic coastal microalgal primary production and photosynthesis. Marine Biology 159, 2827–2837.
- McMinn A., Martin A. & Ryan K.G. 2010. Phytoplankton and sea ice algal biomass and physiology during the transition between winter and spring (McMurdo Sound, Antarctica). Polar Biology 33, 1547–1556.
- McMinn A., Müller M.N., Martin A. & Ryan K.G. 2014. The response of Antarctic sea ice algae to changes in pH and CO2. PLoS One 9, e86984, doi: 10.1371/journal.pone.0086984.
- McMinn A., Müller M.N., Martin A., Ugalde S.C., Lee S., Castrisios K. & Ryan K.G. 2017. Effects of CO2 concentration on a late summer surface sea ice community. Marine Biology 164, article no. 87, doi: 10.1007/s00227-017-3102-4.
- McMinn A., Pankowski A. & Delfatti T. 2005. Effect of hyperoxia on the growth and photosynthesis of polar sea ice microalgae. Journal of Phycology 41, 732–741.
- McMinn A., Ryan K.G. & Gademann R. 2003. Diurnal changes in photosynthesis of Antarctic fast ice algal communities determined by pulse amplitude modulation fluorometry. Marine Biology 143, 359–367.
- Mock T. & Kroon B.M. 2002. In situ primary production in young Antarctic sea ice. Hydrobiology 470, 127–132.
- Mock T. & Thomas D.N. 2005. Recent advances in sea-ice microbiology. Environmental Microbiology 7, 605–619.
- Müller M.N., Trull T.W. & Hallegraeff G.M. 2015. Differing response of three Southern Ocean Emiliania huxleyi ecotypes to changing seawater carbonate chemistry. Marine Ecology Progress Series 531, 81–90.
- Palmisano A.C. & Sullivan C.W. 1983. Sea-ice microbial communities (SIMCO). I. Polar Biology 2, 171–177.
- Rajanahally M.A., Sim D., Ryan K.G. & Convey P. 2014. Can bottom ice algae tolerate irradiance and temperature changes? Journal of Experimental Marine Biology and Ecology 461, 516–527.
- Ralph P.J. & Gademan R. 2005. Rapid light curves: a powerful tool to assess photosynthetic activity. Aquatic Botany 82, 222–237.
- Ralph P.J., McMinn A., Ryan K.G. & Ashworth C. 2005. Short-term effect of temperature on the photokinetics of microalgae from the surface layers of Antarctic pace ice. Journal of Phycology 41, 763–769.
- Ralph P.J., Ryan K.G., Martin A. & Fenton G. 2007. Melting out of sea ice causes greater photosynthetic stress in sea ice algae than freezing in. Journal of Phycology 43, 948–956.
- Raven J.A., Giordano M., Beardall J. & Maberly S.C. 2011. Algal and aquatic plant carbon concentrating mechanisms in relation to environmental change. Photosynthesis Research 109, 281–296.
- Reinfelder J.R. 2011. Carbon concentrating mechanisms in eukaryotic marine phytoplankton. Annual Review of Marine Science 3, 291–315.
- Riebesell U., Wolf-Gladrow D.A. & Smetacek V. 1993. Carbon dioxide limitation of marine phytoplankton growth rates. Nature 361, 249–251.
- Roy R.N., Roy L.N., Vogel K.M., Porter-Moore C., Pearson T., Good C., Millero F.J. & Campbell D.M. 1993. The dissociation constants of carbonic acid in seawater at salinities 5 to 45 and temperatures 0 to 45°C. Marine Chemistry 44, 249–267.
- Ryan K.G., Cowie R., Liggins E., McNaughtan D., Martin A. & Davy S.K. 2009. The short-term effect of irradiance on the photosynthetic properties of Antarctic fast-ice microalgal communities. Journal of Phycology 45, 1290–1298.
- Ryan K.G., McMinn A., Hegseth E. & Davy S.K. 2012. The effects of ultraviolet-B radiation on Antarctic sea-ice algae. Journal of Phycology 48, 74–84.
- Ryan K.G., Ralph P.J. & McMinn A. 2004. Photoacclimation of Antarctic bottom ice algal communities to lowered salinities during melting. Polar Biology 27, 679–686.
- Ryan K.G., Tay M.L., Martin A., McMinn A. & Davy S.K. 2011. Chlorophyll fluorescence imaging analysis of the responses of Antarctic bottom-ice algae to light and salinity during melting. Journal of Experimental Marine Biology and Ecology 399, 156–161.
- Rysgaard S., Bendtsen J., Delille B., Dieckmann G.S., Glud R., Kennedy H., Mortensen J., Papadimitriou S., Thomas D.N. & Tison J.-L. 2011. Sea ice contribution to the air-sea CO2 exchange in the Arctic and Southern Oceans. Tellus Series B Chemical and Physical Meteorology 63, 823–830.
- Schreiber U. 2004. Pulse-amplitude-modulated (PAM) fluorometry and saturation pulse method: an overview. In G.C. Papageorgiou & Govindjee (eds.): Chlorophyll a fluorescence: a signature of photosynthesis. Pp. 279–319. Dordrecht: Springer.
- Suffrian K., Schulz K.G., Gutowska M.A., Riebesell U. & Bleich M. 2011. Cellular pH measurements in Emiliania huxleyi reveal pronounced membrane proton permeability. New Phytologist 190, 595–608.
- Thomas D.N. & Dieckmann G.S. 2002. Biogeochemistry of Antarctic sea ice. In R.N. Gibson et al. (eds.): Oceanography and marine biology: an annual review. Vol. 40. Pp. 143–169. Boca Raton, FL: CRC Press.
- Thomas D.N. & Papadimitriou S. 2003. Biogeochemistry of sea ice. In D.N. Thomas & G.S. Dieckmann (eds.): Sea ice – an introduction to its physics, biology and geology. Pp. 267–302. Oxford: Blackwell Publishing.
- Torstensson A., Chierici M. & Wulff A. 2012. The influence of increased temperature and carbon dioxide levels on the benthic/sea ice diatom Navicula directa. Polar Biology 35, 205–214.
- Trenerry L.J., McMinn A. & Ryan K.G. 2002. In situ oxygen microelectrode measurements of bottom-ice algal production in McMurdo Sound, Antarctica. Polar Biology 25, 72–80.
- Trimborn S., Brenneis T., Sweet E. & Rost B. 2013. Sensitivity of Antarctic phytoplankton species to ocean acidification: growth, carbon acquisition, and species interaction. Limnology and Oceanography 58, 997–1007.
- Ugalde S., Martin A., Meiners K.M., McMinn A. & Ryan K.G. 2014. Extracellular organic carbon dynamics during a bottom-ice algal bloom (Antarctica). Aquatic Microbial Ecology 73, 195–210.
- Young J.N., Kranz S.A., Goldman J.A.L., Tortell P.D. & Morel F.M.M. 2015. Antarctic phytoplankton down-regulate their carbon-concentrating mechanisms under high CO2 with no change in growth rates. Marine Ecology Progress Series 532, 13–28.