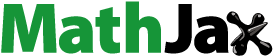
ABSTRACT
Sea surface currents probably are the most relevant essential ocean variable affecting diverse societal challenges concerning the marine environmental (as, for example, safe and efficient navigation, marine pollution and ecological connectivity). This work introduces a climatological Atlas (monthly resolution) of currents in the Mediterranean and Canary–Iberian–Biscay basins, based on today's state of the art reanalyses of the ocean circulation. The focus is on surface and subsurface reanalyses (here understood as and
m, respectively) provided by the Copernicus Marine Environment Monitoring Service (CMEMS). The climatological values are computed from the median of the empirical probability density functions and the Atlas also includes the variance matrix and a bimodality index to have quantitative information on their variability. For both domains, the subsurface climatological fields are reasonably consistent with circulation schemes proposed in the previous literature but clearly improving the time and space resolution of the emerging patterns. For the Canary–Iberian–Biscay domain, the monthly climatological surface currents capture accurately the characteristic seasonal signal and its transition between a favourable and non-favourable upwelling regime. In the Mediterranean basin, differences between the near-surface and the 15 m velocity fields suggest a non-negligible role of winds over the variability of the uppermost ocean layer, specially in the Eastern Mediterranean basin. This is, up to our knowledge, the first time that such near-surface climatological patterns are computed. It has been found that, in general, the resulting patterns agree with surface drifter trajectories. In several regions, interannual variability foster bimodal and multimodal probability distributions. The Atlas has been conceived with the purpose of providing a first quantitative assessment on the surface circulation, thus being a complementary tool of real-time ocean forecasting systems. The Atlas is distributed following the FAIR principles and is accompanied with a digital version, with enhanced visualization capabilities for both research and assessment.
1. Introduction
In today's globalised economy most commodities and manufactured goods are transported through maritime shipping routes. The efficiency and safety of modern navigation systems is linked to main weather patterns and ocean currents. Moreover, the variability of these patterns is also needed to assess the management of the marine space (pollution events, safety at sea, leisure activities, ecosystem and marine biodiversity changes, fisheries, etc.). Consequently, ocean currents are included in both the list of essential ocean variables (EOV), defined by the Global Ocean Observing System (GCOS Citation2011) and the list of the essential climate variables (ECV, Bojinski et al. Citation2014).
The concept of climatology refers to features that emerge when data are averaged over a long period of time. Despite the simplicity of the previous statement, the shape of the permanent features (and its interpretation) varies depending on the particular methodology used to calculate them. At a given time, the oceanographic circulation patterns are the consequence of a large number of processes interacting non-linearly over a huge spatio-temporal range. Therefore, the climatology will also depend on the particular time window and average window length as well as on the presence of unresolved modes of variability or trends. Hence, the question about the representative of such averaged pictures needs to be examined and contrasted with independent data. Long-term averages of observed values have been, and are, the traditional way of rendering our knowledge about what we call climatological fields. These fields provide a general description of the spatial structure of the system providing patterns having recurrences times lower than the resolved climatological period. Thus a climatological year may roughly capture up to seasonal variability but not those patterns with interannual and longer variability.
In the case of velocity fields, climatologies based on measurements remain a challenging topic of the Earth observation system (Isern-Fontanet et al. Citation2017). To elaborate appropriate climatological maps of ocean currents, observational data sets should be spatially dense and embrace a period of time long enough to calculate reliable statistics. Although the first currentmeters date back from more than a century (Woltmann Citation1790; Eckman Citation1932), direct measures of velocity fields, using these devices are relatively sparse (Holloway et al. Citation2011). Long-time direct measurements of velocities from currentmeters are only available for a few sites in the world. Thus, much of the available velocity time series are relatively short in time and/or restricted to a few depth levels. Most of the maps and schemes of the sea circulation up to recent times have been built from hydrographic observations and satellite altimetry through the geostrophic relationship. Geostrophic balance provides a first dynamical contribution to the oceans currents which is easily computed from sampling the vertical thermohaline properties or from sea level gradient in the case of altimetry. A second approach to directly get currents is from the observation of drifters trajectories (see the reviews of Gould Citation2001; Lumpkin and Pazos Citation2007; Maximenko et al. Citation2013). Maps are then built from pseudo-eulerian approaches where drifter velocities are binned into a regular geographical grid (e.g. Niiler et al. Citation2003; Poulain et al. Citation2012). The information from drifters released by the Global Drifter Program (GDP, Niiler Citation2001) is by far the most reliable source of information to get a climatology of near-surface currents (Lumpkin and Johnson Citation2013; Laurindo et al. Citation2017).
A third approach exists based on the reanalysis of the ocean state by mixing existing data with numerical models, these being a kind of four-dimensional dynamic interpolator of data through data assimilation techniques. A review and discussion in terms of advances and challenges of ocean reanalysis can be found in Storto et al. (Citation2019). The major advantage is that we have access in an almost continuous way to the whole domain, including coastal boundaries and for the whole water column. The drawbacks are related with the properties of the models in terms of resolution, parametrisations, sensibility and processes resolved in order to provide realistic fields. Besides its limitations these are the most suitable and probably complete sources to assess climatologies and low frequency pictures of the ocean state.
The Canary–Iberian–Biscay and the Mediterranean basins are two marine systems connected through the Gibraltar Strait (). These areas encompass the major traffic of European marine trade and support a high anthropic pressure over the marine environment. Despite the recent opening of the Arctic Sea to maritime trade, the Mediterranean-Gibraltar-Biscay path still provides the shortest route between Asia and Europe. The straits of Gibraltar, Bosphorus and Suez Channel are three of the eight primary key points in the global trade of goods and commodities (Notteboom et al. Citation2021). Thus, through the Mediterranean basin, which only represents the 0.8% of the world's sea surface, it crosses approximately the 16% of the global marine trade and 33% of the world oil transport. In addition, Mediterranean countries are the preferred destination for 30% of all international tourism (World Tourism Organization Citation2015). This accumulation of population, particularly intense during the summer season, is a challenging situation for managing the maritime space from many aspects, ranging from marine safety issues to the pressure upon ecosystems, eutrophication, fisheries exploitation, pollutants and marine litter (Zambianchi et al. Citation2017).
Figure 1. Schematic localisation of the main features (currents, fronts and gyres) described in the text. The acronyms are listed in . Numbers indicate geographic features. In the Canary–Iberian–Biscay Atlantic basin (grey background): 1 Bay of Biscay, 2 Cape Finisterre, 3 Duero river's mouth, and 4 Cadiz Gulf. In the Mediterranean Basin (white background): 5 Strait of Gibraltar, 6 Ibiza Channel, 7 Gulf of Lion, 8 Corsica Channel, 9 Sardinian Channel, 10 Talbot Bank, 11 Sicilian Channel, 12 Sicilian Plateau, 13 Tunisian Plateau, 14 Gulf of Sirte, 15 Strait of Otranto, 16 Gulf of Venice, 17 Bosphorous Strait, and 18 Suez Canal. The area covered by the Atlas is delimited by the geographical coordinates (25.0N, 25.0
W) and (52.0
N, 36.5
E).

Table 3. List of acronyms.
The Atlantic domain considered here, is the upper part of the North Eastern boundary of the Atlantic subtropical circulation where three main surface currents systems are often identified: the Canary current, the Azores current and the Portugal current system. An extensive review of the circulation and variability for this region is given in Mason et al. (Citation2005). Basically, it has a marked seasonal variability strongly correlated with the atmospheric forcing. In summer, roughly between April and September, the permanent trade winds blow along the African coast inducing the Canary Upwelling System and are southward along shore winds in the Iberian shelf inducing an upwelling regime in the Portugal and North Western Spanish coasts (Alvarez et al. Citation2008b, Citation2011; Santos et al. Citation2011). During the winter season, the atmospheric circulation close to the Iberian Peninsula weakens and a poleward and along shelf flow develops entering the Gulf of Biscay sometimes denoted as Navidad current (Haynes and Barton Citation1990; Peliz et al. Citation2005). This pattern of variability in the Gulf of Biscay is clearly recovered in climatological analysis from drifters (Charria et al. Citation2013).
The Mediterranean domain is shaped by a complex topography with two main sub-basins which in turn include several sub-basins, islands and straits. The most relevant aspect at a large scale is a positive imbalance of evaporation-precipitation that is partially compensated by the exchanges through the Gibraltar strait. Thus at the surface, Atlantic waters enter through the Gibraltar Strait and recirculate over the whole basin gradually changing its properties. Deep water formation and cascading events produce several modal waters that ultimately escape the basin through the lower section of the Gibraltar Strait (Malanotte-Rizzoli et al. Citation2014) made a quite complete summary and review of view of the state of the art of knowledge about the main oceanographic characteristics of the Mediterranean basin.
The closer synthesis to our modern view of the basin circulation emerged at the end of the 90's coinciding with the development of many cruises in the area and the consolidation of the satellite Earth observation programs (e.g. Robinson et al. Citation1992; Robinson and Golnaraghi Citation1993; Millot Citation1999; Hamad et al. Citation2005; Millot and Taupier-Letage Citation2005). Much more recently, the contribution of the ARGO and SVP programs in the Mediterranean, the improvements in data assimilation techniques and the feasibility of eddy resolving numerical models, have allowed to obtain a more quantitative views of the characteristics and variability of the Mediterranean circulation (e.g. Molcard et al. Citation2002; Poulain and Zambianchi Citation2007; Poulain et al. Citation2012, Citation2013; Ciappa Citation2014; Pinardi et al. Citation2015; Estournel et al. Citation2021). Several studies have to build quantitative schemes of the surface circulation but restricted to the geostrophic component (Poulain et al. Citation2012, Citation2013) or derived from SVP drifters' trajectories (Gerin et al. Citation2009) which strictly speaking does not correspond to the surface circulation.
For most of the societal applications mentioned before, the sea near-surface velocity fields are the most relevant. In order to build surface climatological products, only multi-observational products and model reanalysis approaches are suitable to provide this information. Rio et al. (Citation2014) have produced a 27 years long reanalysis at global scale (except the Mediterranean among other regions) combining satellite altimetry and SVP drifters to reconstruct ocean currents at the surface and subsurface (15 m). For the Mediterranean circulation, Pinardi et al. (Citation2015), have presented a 23-years model reanalysis (1985–2007) focused on the subsurface (15 m depth) and intermediate (200–300 m depth) layers.
In the later years, crucial steps have been the implementation of the Medargo program Poulain et al. (Citation2007), which has becoming mature since 2011 experiencing a large and significant increase of the number of profilers deployed; the launching of new ocean observation satellites (Jason-2, Jason-3, Cryosat or Sentinel 3A/3B); and the improvement of circulation models. Thus the aim of this paper is to introduce a high-resolution monthly atlas of the sea surface and subsurface currents in the domain of represented in , based on the latest reanalyses spanning the last three decades. The methodology to derive the climatological atlas is described in the next Section 2, including a description of data sets, the statistical approach and variability indicators. Section 3 is devoted to presenting the results where some qualitative assessment is discussed based on previously published schemes, some independent data, and variability issues. Finally, the general conclusions are given in Section 4.
2. Materials and methods
2.1. Data sets
All the data sets used here are provided by the European Earth observation program available through the Copernicus Marine Service website (CMEMS, https://marine.copernicus.eu/). A summary of the products used to compute the corresponding Atlas is listed in . The disparity of scales and the methods used to build the climatological products of the Atlantic side and the Mediterranean basin makes it difficult to harmonise products in terms of resolution and time coverage. So it has been decided to calculate and distribute them as independent climatological datasets. In the case of the Canary–Iberian–Biscay region, the fields have been computed using the daily dataset consolidated by Globcurrent project (http://globcurrent.org, Rio et al. Citation2014). This product (in the following denoted as GLOBCURRENT) provides total currents at the surface and 15 m. It combines the geostrophic currents derived from altimetry data, the Ekman component derived from the ERA5-ECWMF (European Centre for Medium-Range Weather Forecasts) wind stress products (Hersbach et al. Citation2020) and drifters observations at 15 m extracted from observations of the Surface Velocity Program (SVP Centurioni et al. Citation2017). The daily mean GLOBCURRENT product has been used to generate the Atlas at the original spatial resolution of covering the period 1993–2018, both included.
Table 1. Synoptic information about the products used to compute climatological zonal and meridional velocity.
For the Mediterranean basin, we have prepared two separate climatologies, denoted hereafter as SEALEVEL and MEDSEA to look at the differences between a data based product and a model reanalysis. The SEALEVEL delayed-time product provides Sea Level Anomalies (SLA) and Absolute Dynamic Topography (ADT) computed with respect to a twenty-year mean. The anomalies are then computed by Optimal Interpolation of the along-track data from a wide variety of available altimeter missions (Pujol and Mertz Citation2020; Tabure and Pujol Citation2020). The geostrophic currents are finally obtained from SLA and ADT by assuming a geostrophic balance.
The MEDSEA comes from a model reanalysis (Escudier et al. Citation2020) generated through data assimilation in an hydrodynamic model and forced by ERA5 atmospheric fields (Hersbach et al. Citation2020). The model is the Nucleus for European Modelling of the Ocean (NEMO) version 3.6 (Madec and the NEMO team Citation2016). The assimilated data are in situ temperature and salinity profiles and SLA along track satellite data. The data assimilation scheme applied is the OceanVar scheme developed by Dobricic and Pinardi (Citation2008) and modified by Storto et al. (Citation2016). Additional information about the processing details can be found in the quality information document available in Escudier et al. (Citation2020). Although the MEDSEA product provides up to 141 depth levels, the emphasis here is in the structure of the surface and subsurface circulation. Furthermore, in order to have comparable products for both basin domains, the analysis has been harmonised to the levels provided by SEALEVEL for the Atlantic basin (0 m and 15 m). So the levels of the MEDSEA model reanalysis closest to such depths have been selected, i.e. nominal levels of 1.0182 m (surface) and 16.2706 m (subsurface).
The period covered by the SEALEVEL product, for the Mediterranean domain, coincides with the GLOBCURRENT product for the Atlantic domain whereas, the temporal coverage of MEDSEA is slightly longer (1987–2019). The reanalysis used to built the MEDSEA climatology can be considered an extension of the reanalysis done by Adani et al. (Citation2011), who used previous versions of both the ocean circulation model and the assimilation scheme but limited to the period of 1985–2007. Such product has been used by Pinardi et al. (Citation2015) to make a retrospective analysis of the Mediterranean circulation.
2.2. Climatology
The monthly velocity climatologies have been computed as follows:
(1) | Stratify the time series of daily zonal and meridional velocity values for each grid point by the month of the year. | ||||
(2) | Generate, for each targeted month and grid point, the histograms for the zonal (u) and the meridional (v) components. | ||||
(3) | At each point, calculate the mean, the median, the mode, and the second moment. | ||||
(4) | Use third and fourth moments (skewness and excess of kurtosis) to test the normality hypothesis. These moments, together with the mode(s), are used to detect multimodal distributions. | ||||
(5) | Finally, assume that the median of the corresponding histogram represents the climatological value of that point. |
For the SEALEVEL and GLOBCURRENT products, the points at which the histograms are collected are those defined by the original resolution of the products (1/8 and 1/4
, respectively). However, to provide the same resolution for both Mediterranean climatologies, the data corresponding to the MEDSEA product (originally provided at 1/24
) have been grouped to conform histograms on the same 1/8
grid used by the SEALEVEL product.
Based on the expected accuracy of the products, the histograms for u and v have been created by accumulating their values in bins of 0.02 ms for each grid point i and natural month
. Only velocity values within the interval (−10:10) ms
are considered, resulting in distributions
discretised in 1000 intervals or classes. For any given geographical grid point i and targeted month j, the statistical absolute frequency (histogram) for the class k, (
), is obtained by a simple sum of the number of their u (or v) values in the selected k range regardless of the year. Therefore, we can define
and
as the total number of u (or v) values and the total values of u (or v) for a given grid point i and month j by, respectively, summing over all classes (
) both the statistical absolute frequencies
and the classes values
,
(1)
(1)
2.2.1. The climatological representative: The median
The mean of the distribution for each grid point i and targeted month j is computed using the definitions given by Equation (Equation1
(1)
(1) ) as
(2)
(2) and the median (second quartile
) is obtained through interpolation of
(3)
(3) where p is the percentile (
),
is the lower boundary of the class containing the nth quartile (the kth class),
is its width, N is the number of classes (bins) and
is the statistical absolute frequency corresponding to the lth class. In our study
and
for all classes and ij values. The class k containing the nth quartile is the first one that accomplishes
(4)
(4) The second moment (the variance) is expressed here by means of its square root, i.e. the standard deviation σ, which is computed for each grid point i and month j as
(5)
(5) where the sum extends over all classes, and where
and
are the relative frequency and the mean value of u (or v) corresponding to the class k defined as,
(6)
(6) Finally, using the definitions of (Equation5
(5)
(5) ) and (Equation6
(6)
(6) ), the third and fourth standardised moments (skewness γ and absolute kurtosis κ, respectively) are given by:
(7)
(7) For variables following Gaussian probability distributions the choice of the statistic characterising the central value of the population is irrelevant because the mode, the mean, and the median values coincide. However, ocean velocity time series often deviate from normal statistics (Provenzale Citation1999; Pasquero et al. Citation2001; Isern-Fontanet et al. Citation2006) and skewed distributions with long tails are common. For positively skewed distributions (having a large tail for high distribution classes) the mode appears at lower velocity values than the median, and the median velocity is smaller than the mean one. For negative skewed distributions, the order reverses. As, by definition, the median divides the velocity distribution into two identical areas, we can consider, independently of the shape of the distribution, the median velocity as the best representative value of the central location of the velocity distribution.
A particular aspect to take into account is the presence of outliers, understood as those rare events belonging to the distribution tails. Although the median is less affected by outliers than the mean, it is convenient to apply an outlier detection to avoid false multimodal positives. Thus, an outlier removal process has been performed for each u and v distribution . In this work, a velocity value (u or v) is classified as an outlier if it accomplishes Tukey's rule (Tukey Citation1977):
(8)
(8) In this equation,
and
are the lower and the upper quartile of each velocity component distribution
and its interquartile range
is denoted as IQR. The quartile computation is performed by means of Equation (Equation3
(3)
(3) ).
Skewness and kurtosis provide a first estimate of how much a given distribution deviates from the normal one. Kurtosis is sensitive to departures from normality on the tails, whereas skewness provides information about how much the overall shape of a distribution deviates from the normal one. It reflects the asymmetry of the distribution function. For large enough sample sizes (typically larger than 300), it is acceptable to consider the distribution does not deviate much from a normal univariate distribution if the skewness lies in the range [−2:2] and the absolute kurtosis is lower than 7 (West et al. Citation1995; Kim Citation2013). The data sets used to perform this study cover 27 years for GLOBCURRENT and SEALEVEL products and 33 years for MEDSEA product. Moreover, the MEDSEA climatology has been computed at a resolution 3 times coarser than the original product and the number of available values to compute the climatology at each point and for each month is 9 times larger. This number is slightly reduced due to the outliers detection performed on all products, but for each distribution, it mainly moves in the range 650–800 for GLOBCURRENT and SEALEVEL products, and 7500–9000 for MEDSEA product, long exceeding the mentioned limit of 300 measures and the normality test can be applied without the need to resort to z-test, K–S tests or Q–Q plots. Only small deviations from normality are observed for the three data sets described in .
2.2.2. Multimodal distributions
For multimodal distributions, the climatological concept is not generally clearly defined. Therefore, when calculating climatologies, the detection of the multimodal character of a given distribution is of importance. Skewness and kurtosis can help to reveal if a distribution is not unimodal. For example, the Sarle's coefficient (β) defined as
(9)
(9) provides a condition to characterise bimodality (Knapp Citation2007): values of β larger than 5/9 (the value for a uniform distribution) may indicate bimodal or multimodal marginal distributions.
The relative position and amplitude of the peaks (the modes) can be characterised by means of the bimodal amplitude (), and bimodal ratio (
) defined as
(10)
(10) respectively (Zhang et al. Citation2003). The subscript v indicates the class with the minimum amplitude between peaks, subscript m indicates the class corresponding to the smaller peak, and subscript M refers to the class of the larger one. The bimodal separation (
) (Zhang et al. Citation2003) has been simplified in our study as the separation between the classes corresponding to the peaks referring to the distribution width
(11)
(11) where
and
are, respectively, the first and last classes accomplishing
(12)
(12) Therefore
, corresponding the value 1 to U-shaped distributions like the arcsine distribution and 0 to unimodal distributions.
In order to improve the estimation of these quantities, its necessary to reduce the high-frequency noise present in empirical histograms. This reduction can be achieved by simply applying a centred rectangular moving window to the original distribution. This moving average filter allows to reduce random white noise while keeping the sharpest step response (Smith Citation1997). To preserve the position of the peaks, it is necessary to apply an odd rectangular window width (w = 2n + 1; ). Additionally, the width of the moving window has to be small compared with the width of the original time series. In many cases, it is useful to apply the smoothing window multiple times. These multiple-passes increase the high-frequency noise reduction and it is especially effective in highlighting the peaks of a distribution. The more passes, the fewer peaks remain. The multiple-times moving rectangular window filtering is equivalent to a pseudo-Gaussian smoothing window of width
where p is the number of passes of the moving window. The optimal value for the window width depends on the variability of the original distribution (i.e. the number of non-empty classes in which the data has been distributed). For GLOBCURRENT and SEALEVEL products, the use of three passes and a window having a wide of 3 or 5 points gives good smoothing results. The MEDSEA product provides distributions with a wider velocity range, and some of them can be filtered using up a 7-point window width without degrading them excessively. shows the ratio of the coefficients for the pseudo-Gaussian filter in all used cases. Note that the application of an excessively wide pseudo-Gaussian filter could underestimate the bimodal separation value. The smoothed histogram is used to locate the class at which the maximum value is attained as well to compute equations (Equation10
(10)
(10) ) and (Equation11
(11)
(11) ). It should be noted that a climatological representant is still reliable for bimodal distributions having small values of bimodal amplitude, bimodal ratio, or bimodal separation.
Table 2. Equivalence between applying three times (p = 3) a square window and the corresponding pseudo-Gaussian smoothing filtering coefficients ratio.
Inspection of all the distributions satisfying the bimodality condition () shows that those having a bimodal amplitude below 0.15 are statistically indistinguishable from those having long tails. Also, bimodal distributions having the secondary peak much lower than the main one or those with the two peaks too closer can be considered as unimodal in terms of the characteristic value computation. Therefore, we only consider as truly bimodal distributions those accomplishing
(13)
(13) where
,
, and
are given by expressions (Equation10
(10)
(10) ) and (Equation11
(11)
(11) ).
2.3. Variability
The representativeness of a climatological value depends on the variability around that value. It is, therefore, useful to provide an estimate of the temporal dispersion of the data. The standard deviation provides a measure of the spread of the data. However, for bivariate data such as horizontal ocean velocities two more intuitive variability measures can be used: variance ellipses and current roses ().
Figure 2. Left: Covariance ellipse computed for April surface velocity data coming from GLOBCURRENT product. Right: Current rose for the same location and month.

The spread of bivariate data may be characterised by their covariance matrix (see Stewart et al. Citation2015 and references therein, and Deakin Citation2005). In particular, for 2D velocity fields with components u and v, the covariance matrix can be expressed as
(14)
(14) where the overlined quantities refer to mean values.
Covariance matrices are symmetric, so they are always diagonalisable. Therefore, it is possible to find the direction (in terms of u and v) of maximum variance of the data. This one coincides with the direction of the eigenvector having the largest eigenvalue and its magnitude is the eigenvalue value. The second eigenvector defines the direction of the second largest spread of the data about its mean. For symmetric matrices, this direction is always orthogonal to the first one. The spread of data magnitude, i.e. the variance, along this direction is given, as in the previous case, by its eigenvalue.
For covariance matrices, the eigenvalues are easily found analytically by Preisendorfer and Mobley (Citation1988):
and the rotation angle of the eigenvector associated to
with respect to the u axis is given by:
(15)
(15) The covariance matrix of the velocities rotated by such angle θ is given by a diagonal matrix with elements
and
. Therefore, in this rotated frame and for normal distributions, the ellipse of semi-axis
and
encircles approximately the 68.27% of the data, ellipse having semi-axis
and
encircle about a 95.45% of the data,
and
ellipse encircles the 99.73% of the data and so on. The spread of the data can be visualised in a u−v frame as rotated ellipses centred at the mean values. Each of these ellipses defining different confidence levels. Usually, they are plotted as centred in the origin and including a vector pointing to the mean value (see left-hand side of ). The median is assumed as the climatological value, so a vector pointing to the median value is also included.
A more intuitive information about the main direction of the current and the scattering to both sides for a given month and location is provided by the current roses. Current roses, preferred for nautical purposes, have been constructed following Wyrtki (Citation1960) recommendations. The current roses are divided into 16 directions to provide a complete information of the scattering of the currents. The variability of the ocean currents at each point and month is indicated by the percentage of observed directions (see right-hand side of and ).
Figure 7. Alboran current direction distribution from 1987 to 2019 for July from MEDSEA product at 2.81E, 37.44
N. Left: surface in December. Center: surface in July. Right: subsurface in July.

In the Mediterranean sea, the variance ellipses and the current roses have been computed grouping the data available for each natural month at each 1/8 SEALEVEL cell. The same grid has been used for MEDSEA. The latter is provided in a grid three times denser than the former (1/24
). Therefore, the set used to create each variance ellipse for MEDSEA contains the data of each 3×3 original cell. On the contrary in the Canary–Iberian–Biscay Atlantic region the original 1/4
grid has been used to compute the variance ellipses in water covering the continental shelf. Nevertheless, due to the less spatial variability offshore, the used grid there was 1
.
Finally, the Atlas includes the climatological current value, the skewness, the kurtosis, and the bimodality flag condition. To follow the guidelines of the FAIR principles (Wilkinson et al. Citation2016), the Atlas has been deployed in the certified repository DIGITAL.CSIC (Martínez et al. Citation2021). Readers are invited to access the site https://cosmo.icm.csic.es/currents, with interactive visualization capabilities to consult all the monthly climatological velocity fields and including graphical information of point by point variance ellipses and currents roses.
3. Results
In this section we will review the main circulation patterns appearing in the Atlas and how they compare with the ones reported in the literature, focusing on the added value of the monthly climatologies. We have computed the climatological currents for the products listed in , collecting all the acronyms definitions for most of the characteristic current patterns in .
The figures below display velocity fields via their Lagrangian representation: Lines represent the trajectories that floating particles would follow during a given period of time. Darker regions indicate intense currents, i.e. squeezed streamlines.
3.1. Canary–Iberian–Biscay domain
In , we represent some selected monthly fields of GLOBCURRENT climatology for the Iberian-Biscay region, reflecting the characteristic dynamics associated to this part of the North Atlantic Gyre (NAG Mason et al. Citation2005; Charria et al. Citation2013). It is strongly constrained by westerly winds at the north and by the trade winds in the south (Alvarez et al. Citation2008, Citation2011). In spring, and especially in summer, the returning branch of NAG induces the southward North Atlantic Drift (NAD). NAD is observed in April, being clearly established during July–August ( centre) and is absent after October.
Figure 3. Surface flow in December (left) and July (center). The July subsurface situation (15 m) is shown on the right. Thick lines indicate the schematic main directions of the flow. Thin lines are the Lagrangian representation of current at the atlas resolution.

The undulating Western Iberia Winter Front (WIWiF, see the left panel of ), represents the transition to the southern area of the Iberian Basin. Located around 41, slightly north of the location indicated by Peliz et al. (Citation2005) and Mason et al. (Citation2005), it bifurcates in two branches (northward and southward) along the Iberian Coast. The north branch follows the Galician coast towards the Gulf of Biscay, where it contributes to the Rennell Current (RC) that goes further to the north close to the French coast. The southward branch is distorted by the presence of several gyres.
In the left panel of , the relatively narrow undulating zonal band along the 35–36N corresponds to the Azores Current (AZC). As it approaches the Gulf of Cadiz, it starts to recirculate southward through the Canary archipelago and along the Western African coast. The undulating shape of these southward currents towards could be explained because of the presence of several bottom seamounts near and north of Madeira island. The AZC is detected on the surface during the October–February period. However, at 15 m depth, the period extends, and the AZC does not completely disappear until June, reappearing in September (see right for July subsurface currents pattern). The Canary Current (CNC), located at the south of the AZC, is permanently present on the surface, reinforced by the trade winds and having an SW-WSW direction. Although the recirculation of the AZC only feeds the CNC in winter, the CNC also intensifies in summer, driven by the north-present circulation patterns throughout the Iberian basin and by the action of the Canarian Upwelling System.
As a summary, and at the surface, four main zonal sectors can be clearly defined in winter. These sectors are delimited, from north to south, by the currents generated by westerlies (around 50N), the WIWiF (around 41
N), AZC (around 35
N) and CNC (roughly delimited by the Moroccan coast and the Canary Islands).
In the north, and according to Santos et al. (Citation2011) and references therein, the upwelling region along the Galician Coast and the western coast of the Iberian Peninsula is considered to be the northern edge of the Canary Upwelling System. The works of Alvarez-Salgado (Citation2007), Alvarez et al. (Citation2008, Citation2008b) and Herrera et al. (Citation2008) describe the western Iberian Peninsula upwelling occurring during the spring-summer season. Our Atlas reproduces this feature: it clearly starts in April and it encompasses a region between the mouth of the Duero river and the Galician coast. It progressively extends to the south while increasing the intensity in May and June. The upwelling is still intense in the July–August period and decreases in September ceasing in October. The surface currents during the upwelling regime on the coast of the Iberian Peninsula in August is visible in (a). The geographical extension and the time-line roughly coincide with such obtained from the monthly Ekman pumping velocity by Alvarez et al. (Citation2008). Although the surface signature is clearly indicated by the divergence of surface fields, the offshore cross-shelf circulation is better appreciated at the subsurface field.
Figure 4. Correspondence between the GLOBCURRENT climatology (thin black lines) and surface drifters trajectories (in red and yellow). Thick black lines with arrows indicate the direction of the flow streamlines. (a) June–August drifters trajectories over-impressed on the August climatological surface current. Trajectories start in June in the north. Yellow dots indicate the position of the drifter at the beginning of each month over the period under consideration and December climatological surface current. The trajectories of seven drifters deployed in November 2006 (red) and seven drifters deployed in December 2002 (orange). Yellow dots indicate the initial deployment positions of drifters.

In the most meridional sector, northeast and north winds intensify the CNC at the surface during spring and summer (see ). They also induce in August and September, at the southwest of the Madeira and Canary Islands, the formation of eddies and vortex dipoles, more intense in the subsurface layer. In July, the CNC, close to the African coast, reaches values up to 0.25 ms at the surface, whereas the Canary Current offshore speeds are around 0.15 ms
. The speed reduces by half at subsurface level. Part of this strength increase in July can be due to the Canary upwelling reinforcement that takes place in summer coinciding with the Trade winds maximum (Mason et al. Citation2011).
Figure 5. Mean monthly winds in July (upper figure) and December (lower figure) according to ERA5 from 1987 to 2019 at 1000 mb level.

Besides the qualitative agreement with the mentioned published schemes and results, the currents of the Atlas are also consistent with independent data drifters trajectories. In , we overlap the climatological northeast flow of the Iberian Peninsula with surface drifters for two different situations. In the upper side of (a), two surface drifters released near the shelf (Sotillo et al. Citation2008) clearly match the characteristic flow towards the south in summer which is the period under the upwelling regime. In the second example ((b)) climatological currents of December are compared with two sets of drifters experiments deployed in the Cape of Finisterre (in December 2002 and in November 2006, García-Ladona et al. Citation2005, Citation2015). The trajectories also have a high correspondence with the corresponding monthly Atlas currents and consistent with past observations (Haynes and Barton Citation1991). However, as drifters approach the east of the Bay of Biscay, their motion became quite turbulent, separating from the smoothed fields that we observe in the Atlas. This could be due to the high variability of the direction of the currents in this zone, but also to the large tidal correction necessary to obtain the velocity maps in this region.
3.2. Mediterranean
The creation of a monthly climatology differentiating between surface and subsurface allows us to distinguish the characteristic seasonal circulation features, evidencing potential differences between summer and winter features and, more importantly, influence of the wind-induced forcing. In , we have represented some characteristic examples of the Mediterranean circulation obtained from the MEDSEA climatology. We can observe the marked differences in summer between the surface and subsurface fields and between a typical winter and summer situation. These differences are prominent in summer when certain Mediterranean areas are hit by winds. Regarding this seasonal variability, we have to remark that the MEDSEA fields exhibit significant monthly variability compared with the SEALEVEL climatology which remains quite stationary (not shown). On the other hand, the winter subsurface patterns are quite similar to the schematic mean subsurface patterns obtained by Pinardi et al. (Citation2015, see their Figure 12).
Figure 6. Climatologic currents for MEDSEA product. (a) Surface currents in December. (b) Surface currents in July and (c) Subsurface currents in July.

The surface-subsurface difference in the MEDSEA climatology is more marked during the summer months (compare (b,c)). These differences should be attributable to the surface response to the wind included in the model reanalysis. The monthly mean of the ERA5 reanalysis product () computed for the period 1987–2019, shows the presence of strong northwesterly winds in the Gulf of Lion, the Ionian Sea, and in the Levantine Sea. Intense northerly winds blow the Aegean Sea in summer (see ) whereas the presence of easterly winds on the Algerian Coast in summer could explain the seasonal deviation to the north of the Algerian Current (AC) (see (b,c), and the current roses from ). This behaviour, much more intense in the surface layer than in the subsurface, was originally described by Millot (Citation1985) and found also by Pinardi et al. (Citation2015) in the subsurface layer. The ERA5 reanalysis product reinforces the hypothesis that the Algerian Coast is, in summer, an area of upwelling as was pointed out by Bakun and Agostini (Citation2001).
Similarly, the summer NW winds affect the uppermost layer of the Northern Current (NC) as it passes in front of the Gulf of Lion (compare (a,b)). This response has not been reported in the literature, presumably because the surface patterns are usually established from SVP drifters' observations (Poulain et al. Citation2013; Malanotte-Rizzoli et al. Citation2014). These drifters outline the 15 m currents (subsurface) and are less affected by wind. To this end, the reader can compare the wind maps from , (a,b), and the central and the right parts of .
The MEDSEA climatological fields of summer, (b), seems to be induced by the dominant wind field (top of the ) forcing a southward surface current field in the Eastern Mediterranean. The non-negligible role of winds (Gerin et al. Citation2009) in shaping the climatologic circulation in this area seems to be supported by surface drifters trajectories according to . Following Poulain and Zambianchi (Citation2007), the data provided by CMOD-100 drifters are included in the figure because we assume that these drifters lost rapidly their drogue and, as a result, they move with the surface currents.
Figure 8. Surface drifters trajectories of July over-impressed to the monthly climatological surface current provided by MEDSEA. The trajectories have been extracted from the COSMO database (https://cosmo.icm.csic.es/drifters/) and post-processed to include only points corresponding to July independently of the year. The beginning of the July trajectory is indicated by a yellow dot.

It remains to be investigated to what extent the role of the wind might be overestimated by the model reanalysis, exaggerating the near-surface vertical shear we observe in our climatology. Nevertheless, the summer wind effect on the surface currents is also reported by the US Atlas of Pilot Charts (NGA Citation2002).
3.2.1. Western Mediterranean
The Alboran sub-basin is dominated by the Western Anticyclone Gyre (WAG, see Renault et al. Citation2012; Sánchez-Garrido et al. Citation2013 for details), which is present all the year in both products (MEDSEA surface and subsurface, and SEALEVEL). However, the product derived from altimetry is more dominated by gyres, resulting in a weaker Atlantic Jet (AJ). The Eastern Anticyclonic Gyre (EAG) and Almeria-Oran Front (AOF) appear usually in July and are completely established in August, remain until October, weaken in November, and vanish in December. This behaviour coincides with the description by Renault et al. (Citation2012) as well as the appearance of the Eastern Cyclonic Gyre (ECG) at the East of the AOF in the same period. This gyre is more clearly defined in the MEDSEA product, coinciding with a higher transport associated to the highest intensity of the AJ.
In winter (see (a)), the Alboran Current (AC) continues as the Algerian Current up to the Sardinia Channel where it is reinforced by the Western Sardinia Current (WSC) (Olita et al. Citation2013). The AC splits into three branches: One follows the north coast of Sicily and turns northward towards the Tyrrhenian Sea, the other eastward through the Sicily Channel, and the last one moves towards the Tunisian Plateau slope. The Tyrrhenian branch runs along the north coast of Sicily and moves northward along the west coast of Italy up to arrive at Corsica where forms the cyclonic Northern Tyrrhenian Gyre (NTG) and partially flows through the Corsica Channel arriving to the Liguro–Provencal sub-basin forming what is known as the Northern Current (NC). The NC travels south following the French and Spanish coasts up to the Balearic Sea where turns east forming a meandering free jet, the Western Mid-Mediterranean Current (WMMC), described by Pinardi et al. (Citation2015). The latitudinal position of this jet depends on the month but is located between 39N and 41
N and turns south in Sardinia west coast forming the WSC.
The situation is different in summer (see July surface and subsurface currents in (b,c)). As has been mentioned, the AC deviates north, especially in this season, and a northward current crosses the Ibiza Channel (similar to Tziperman and Malanotte-Rizzol Citation1991; Pinardi et al. Citation2015) and turns east to feed the WMMC. The AC divides in the Sardinian Channel, where the Tyrrhenian branch moves towards the Sardinian east coast travelling northward up to the NTG where its cyclonic rotation prevents their passage further north. Therefore, this current comes back to the south following the west coast of Italy up to the northeast Sicilian coast where part of the current is poured into the Sicily channel.
3.2.2. Eastern Mediterranean
At the Sicily Channel, the summer and winter circulation patterns differ. During winter, the Algerian Current is divided into two currents due to the bathymetry. The climatological fields show that both currents separate at the Talbot Bank, a sedimentary bank located at a depth less than 40 m (Civile et al. Citation2016). One of the resulting currents moves following the Tunisian Plateau slope. The other, weaker, advances along the Sicilian Plateau slope passing between Malta and Sicily. Both SEALEVEL and MEDSEA climatologies show an acceleration of the flow at this place. In the case of MEDSEA surface and subsurface products, the Tunisian branch travels to the south turning up to the north in front of the Tripoli coast joining the Sicilian branch at the east of the Malta Island. On the contrary, the branches appearing in SEALEVEL join at the west of Malta generating the Atlantic-Ionian Stream (AIS). This is a more complex behaviour than such described for surface waters by the literature (Astraldi et al. Citation1999; Poulain et al. Citation2013; Malanotte-Rizzoli et al. Citation2014).
During summer, the Tunisian surface branch wears off in the MEDSEA product, partially due to the easterly winds that weaken the Algerian Current (). The subsurface currents also show a very weak Tunisian branch. Although to a lesser extent, weakening of this current is also evident in SEALEVEL being the Sicilian branch the main contributor to the AIS in this season.
The Western Adriatic Current (WAC), coming from the north of the Adriatic Sea, travels southward in front of the Italian Coast towards the eastern coast of Sicily. This current, together with AIS conforms, in winter, the Mid-Ionian Jet (MIJ), that moves eastward (upper ). The location of the MIJ resulting from MEDSEA and SEALEVEL is about around 36.5N, i.e. displaced southward from the position estimated by Poulain et al. (Citation2013). In summer, due to the action of northeasterly wind, the MIJ disappears at the surface from MEDSEA, but not in the subsurface and neither from SEALEVEL product.
The fraction of the AIS not contributing to the MIJ, continues into south limit of the Ionian Sea, turning south in the Libyan coast and eastward in the Gulf of Sirte up to Tripoli, where it travels northward and joins to the main AIS, this pattern coincides with the schema shown by Poulain et al. (Citation2013).
The entrance of surface water to the Adriatic Sea from the Ionian Sea takes place in the eastern side of the Strait of Otranto and it follows the east coast towards the north. The Adriatic Sea is dominated by the nearly permanent Southern Adriatic Gyre (SAG) and the WAC (Astraldi et al. Citation1999; Poulain et al. Citation2013). The location of the SAG, present all year long in both products, maps the deepest bathymetric part of this sub-basin. Both products show the Middle Adriatic Gyre (MAG) (Artegiani et al. Citation1997) located just at the north of the SAG during all year (). Only small changes in the shape of the MAG are observed along the year when, according to SEALEVEL and MEDSEA products, three or even four gyres are aligned in the Adriatic Sea. The northernmost one located at the Gulf of Venice.
The superficial WAC originates in the northern part of the Adriatic Sea and it is reinforced coinciding with the maximum Po river discharge, typically in December, as has been pointed by Cushman-Roisin et al. (Citation2001). This current travels to the south and, according to MEDSEA product, is slightly stronger in winter.
As has already been mentioned, the summer irruption of the northeasterlies could be the reason for the differences between the surface and the subsurface currents (see east Mediterranean in ). Nevertheless, in winter the differences between both layers are small. The subsurface patterns provided by MEDSEA are very similar all year around, although some changes in intensity appear.
The Eastern sub-basin is dominated by the Libya-Egyptian Current (LEC). This coastal current feeds the Cilician Current (CC) in the north and the Asia Minor Current (AMC) when arriving at the west towards the Crete Island (Menna et al. Citation2012). Both Mediterranean products indicate that the Mid-Mediterranean Jet (MMJ) is reinforced, in winter, from the AMC retroflection. The AMC turns south in arriving to the Rhodes Island and to the east when it overpasses the south of the Crete Island forced by the Mid-Mediterranean Jet coming from the Ionian Sea. The retroflection gives rise to the Rhodes Gyre (RG). According to the MEDSEA subsurface layer, the RG moves to the west in spring, it gets weak at the beginning of the summer and finally, it is reinforced at the end of the summer and in the fall.
The Ierapetra Eddy (IE) is present in the MEDSEA product from October to January, in both the surface and subsurface layers. However, it is already visible since August in the SEALEVEL one. It is worth noting the high temporal variability reported by Mkhinini et al. (Citation2014) and Ioannou et al. (Citation2017) in the stages of this eddy: formation, maturity, intensification, mergings, and dissipation. This large variability is also present in the products used to generate the Atlas, affecting the reliability of the climatologic value in this zone.
The Mersa-Matruh Eddy (MME) is well defined during all year but, for MEDSEA, this is only true in the subsurface, possibly due to the high impact that winds have on Eastern Sea surface circulation in summer in this product. The same for the Cyprus Eddy (CE), although its centre is displaced towards the west in March and returns to the east in October–November.
The Aegean Sea shows a complex circulation pattern, resulting from the presence of a number of islands and islets present at the region. The general circulation shown by MEDSEA product consists of a northward current in the east of the sub-basin that arrives up to the Thracian Sea and returning to the south at the western part of the region. This pattern agrees with Olson et al. (Citation2007) except for the surface layer in summer. The SEALEVEL product does not describe accurately enough the currents of this sub-basin, probably due to the lower accuracy of sea level estimates due to the intricate orography.
3.3. Multimodality and variability
Detection of regions having a high variability is key to establishing the reliability of the climatologic value. To this end the Atlas provides the standard deviation of the zonal and meridional components of the velocity and a bimodality flag. This bimodality flag takes the values 0 (no detected modality), 1 (if Sarle's coefficient is larger than 5/9) or 2 (if conditions (Equation13(13)
(13) ) are accomplished).
As is expected, regions containing vortex dipoles and transient eddies have a large variability. Such regions are, in the Atlantic basin, the southwest of the Madeira and Canary Islands in summer. In the Mediterranean, the larger variability is obtained in the Alboran Sea all year, in the zone of the Ierapetra Eddy during autumn, and to a minor extent in the Algerian Current.
Regarding multimodality, in the Atlantic basin only a few isolated points of the AZC and close to the Strait of Gibraltar show bimodal distributions. In the Mediterranean basin, the SEALEVEL product shows a noteworthy behaviour but not the MEDSEA product. This is due to the wider velocity range of the latter and its smoother temporal changes.
The SEALEVEL bimodality is restricted to some months and very localised areas. is a synopsis of its temporal and spatial extent: The Ionian Sea, close to the Greek coasts; the Alboran Sea, close to the Almería-Oran front; and the Ierapetra Eddy region are the most important. An inspection of the temporal evolution of the currents in such places indicate that the bimodality for a given month is due to the interannual variability and not to the month variability.
Figure 9. Points affected by bimodality for SEALEVEL product. Purple circles indicate points where bimodality affects to the zonal component (u) whereas the green ones indicate that bimodality affects to the meridional component (v). Semitransparent circles indicate that bimodality has been detected only in one climatological month.

In the Ionian Sea, close to the Greek Islands, the bimodal situation is detected in January and August–October for the meridional velocity. As it is shown in (top), the cause is the different current regimes in some years (1993–1996 and 2006–2007) for which the current travels to the south. The rest of the period the meridional speed is much lower and does not have a clear behaviour towards north or south. This behaviour is also detected for the MEDSEA product in both, the surface and the subsurface layers (center and bottom of ). As has been reported by Gačić et al. (Citation2010), Poulain et al. (Citation2012), Pinardi et al. (Citation2015), and more recently by Menna et al. (Citation2019), the period prior to 1997 is dominated by an anticyclonic circulation. This behaviour is shown also by both products used in the Atlas and also in the short period 2006–2007. This second anticyclonic period has been also reported by Menna et al. (Citation2019) although in their case the period extends from 2006 up to 2010. During these anticyclonic periods, the circulation in the Ionian Sea is dominated by MIJ, which is located far north and beyond the latitude indicated by the climatological value. According to the same authors, for the rest of the years, a cyclonic regime takes place in the intermediate layer due to the occurrence of the Eastern Mediterranean Event (EMT, Roether et al. Citation1996). Therefore, an coinciding with Poulain et al. (Citation2012), it is not observed in the surface and subsurface layers.
Figure 10. Meridional component for each September for the whole period used to compute the Atlas (left) and the corresponding histogram (right) at 19.6875E, 38.1875
N (close to Kefalonia, Ionian Islands). Top row: SEALEVEL product. Middle and bottom rows: MEDSEA product at 1 m and 16 m depth, respectively. Dashed vertical lines indicate the transition from anticyclonic regime (negative values of meridional velocity)

The bimodal situation in the Alboran Sea extends from June to September for zonal velocity component and along June and July for the meridional one. This change in the regime of circulation among the years is mainly linked with the formation of the AOF and periods in which the EAG is displaced to the east. The mean circulation pattern for the considered products in June of the years 2007 and 2009, for which the AOF is not formed, are in accordance with those described by Renault et al. (Citation2012). This situation is also observed in 2011 and in the 2014–2017 period for SEALEVEL and MEDSEA although the latter does not show an important bimodality.
Regarding to variability and multimodality, the most complex situation takes place in the Ierapetra Eddy zone. The currents located at the southeast of the Crete Island are affected by multimodality during the August – January period, mainly for the zonal velocity, being more important in October when the meridional velocity is also affected. In this area (see ), it is not unusual to find distributions for zonal velocity that have three and even four modes. Such distributions are an indicator of the high interannual variability of the evolution of the Ierapetra Eddy (formation, intensification, and dissipation). This large variability coincides by the reported by Mkhinini et al. (Citation2014) and Ioannou et al. (Citation2017).
4. Conclusions
Persistent general circulation schemes and atlases continue to be a useful source of information that provides a first assessment on how the ocean circulation is organised. These schemes are the synthesis of our evolving knowledge about ocean dynamics that improves according with the availability of new observational capabilities. The combination of state of the art realistic ocean circulation models, the Earth satellite observation program and the maturity of ARGO and SVP programs have facilitated the development of reanalyses spanning three decades. These products may now serve not only to reassess the classical circulation schemes but also to provide quantitative information about the circulation patterns with unprecedented detail and may even be used for operational purposes.
In this paper, we have presented high-resolution climatological Atlases of surface and subsurface currents for the Canary–Iberian–Biscay and the Mediterranean basins from recent reanalyses of ocean the ocean circulation. The focus has been on the fields at the surface m and subsurface
m. Up to our knowledge, this work is the first time that climatological values of total velocity fields at the surface, which is the relevant field for many environmental applications, are provided. In previous quantitative schemes either the analysis was done from mean geostrophic components (e.g. Poulain et al. Citation2012, Citation2013) or restricted to the 15 m analysis (Pinardi et al. Citation2015). Those directly established, based upon pseudo-eulerian computations of drifters trajectories or combined with geostrophic altimetry, have been built based on the information of SVP drifters (Charria et al. Citation2013; Rio et al. Citation2014; Menna et al. Citation2019). The subsurface fields here provided allow us to check the consistency of this atlas by comparing it to previously published schemes, particularly those built based on the information of SVP drifters.
Generally speaking, the main subsurface patterns are consistent and agree with such schemes (Poulain et al. Citation2012; Charria et al. Citation2013; Menna et al. Citation2019). For the Atlantic area, the computed Atlas is based on the 27 years GLOBCURRENT reconstruction from observational systems (SVP drifters and altimetry). The product captures and recovers the characteristic switching between a seasonal upwelling and a coastal poleward flow regime. In winter, the general surface circulation is zonally divided. From north to south we have a northern flow forced by westerlies around 50N, the ondulating Western Iberia Winter Front roughly located at 41
N, the Azores Current at 35
N and the SW-WSW Canary Current. The monthly, high-resolution climatology is able to capture the temporal an spatial details of this seasonal cycle. When compared with surface drifter trajectories, the agreement appears to be quite good and representative in terms of both the spatial and temporal structure. The differences appear close to the corner of the Bay of Biscay where drifters exhibit small-scale variability missing in the climatology probably attributable to resolution limitations of the original product itself or to the large tidal correction that is necessary rather than to the climatology procedure.
In the Mediterranean, the SEALEVEL product and the MEDSEA subsurface layer currents are in a reasonable agreement with features described by the most recent schemes Poulain et al. (Citation2012, Citation2013). The main differences with the most recent ones (Poulain et al. Citation2013; Pinardi et al. Citation2015) are displacements of some gyres and currents. In particular, the Mid-Ionian Jet is found at 36.5N, this is to the south of the position indicated by Poulain et al. (Citation2013). Nevertheless, as it has been pointed out, this is a climatologic position, and during the anticyclonic regime, its location is much further north.
Remarkably different patterns appear regarding the MEDSEA surface currents in summer. They are easily recognisable in three zones: the Gulf of Lion, the Algerian Current, and the Eastern Mediterranean (Ionian, Aegean, and Levantine seas) which seem to be correlated with summer dominant winds. It must be noted that, in summer, the signature of winds upon the surface currents in the Eastern basin agrees with observed surface drifters trajectories. Unfortunately, there is a shortage of surface buoys in summer in the Alboran Sea and the Gulf of Lion that prevents elucidating if the wind effects on the surface current reported by the atlas at these places are overestimated or not by the model reanalysis.
Finally, well beyond the improvement of our understanding of circulation patterns provided by the new climatological reanalyses, especially from the point of view of their spatial resolution, there remains the question of whether they can be used for operational purposes. The assessment of the GLOBCURRENT product made by Hart-Davis et al. (Citation2018), using SVP trajectories, exhibited a general agreement, although some biases, regionally dependent, were observed. They also tried to use the GLOBCURRENT product for a search and rescue case of a capsized catamaran in the Agulhas region. However, they concluded that a Lagrangian tracking tool coupled to the GLOBCURRENT currents was inappropriate to forecast the real trajectory particularly because the target has not the same drift as a SVP-like drifting buoy. Here, in contrast, the examination of a few examples comparing streamlines with surface drifter trajectories allows us to suggest that these climatological Atlases have a potential use as a complementary tool for operational purposes. Obviously, the lack of transient features prevents direct comparison with (or to substitute) the skill of dedicated ocean forecasting systems, which is not our intention. However, it seems to be valid for a first assessment to take decisions in facing marine emergencies. In the case of search and rescue operations, they can be used to define quickly initial searching areas. Systematic and continuous validation of these products is therefore encouraged to assess both: their utility during emergencies, and the genuineness of the observed permanent features.
Acknowledgments
The authors would like to thank the institutional support of the ‘Severo Ochoa Centre of Excellence’ accreditation (CEX2019-000928-S).
Disclosure statement
No potential conflict of interest was reported by the author(s).
Additional information
Funding
Notes on contributors
Justino Martínez
Justino Martínez was born on February 13, 1966, in Girona, Spain. He received the B.Sc. degree in Physics 1991 and the Ph.D. in Physics in 1995 from the Autonomous University of Barcelona, Barcelona, Spain. Currently, he is working as contracted researcher with the Institute of Marine Sciences (ICM-CSIC), Barcelona, in the Physical Oceanography and Technological Department. He has worked on Remote Sensing and is currently focused on extreme events, Lagrangian dynamics, and numerical modeling.
Emilio García-Ladona
Emilio García-Ladona is currently staff researcher at the Marine Sciences Institut (ICM-CSIC) in Barcelona. Graduated in Physics by the Autonomous University of Barcelona (UAB) in 1986, in 1991 he received his Ph.D degree in Physics by the same university and became lecturer between 1990–1995. In 1995 he moved to ICM-CSIC where he has leaded the Physical Oceanography Dept. (2007–2009) and then, ICM Deputy Director (2009–2012). From 2010–2021 he has been a member of the Oceanographic Section from the Spanish Geodesy and Geophysical Commission, a collegiate and consultation body of the Spanish Ministry of Public Works. The researcher interest and trajectory have been mainly focused in the study of ocean currents and processes, lagrangian dyanamics in particular with respect to the Western Mediterranean region. Other research interest goes from data analysis and pattern recognition techniques to ecological modelling.
Joaquim Ballabrera-Poy
Joaquim Ballabrera-Poy was born on March 30, 1966, in Barcelona, Spain. He received the B.Sc. degree from the University of Barcelona (1991), Barcelona, and the Ph.D. degree in Geophysics from the Joseph Fourier University, Grenoble, France (1998). He is currently a senior researcher with the Institute of Marine Sciences (ICM-CSIC), Barcelona, working on numerical modeling, data assimilation, remote sensing and in-situ observation of the marine environment.
Jordi Isern-Fontanet
Jordi Isern-Fontanet was born on 1975, in Banyoles, catalonia. He received the B.Sc. degree from the Universitat de Barcelona (1998) and the Ph.D. degree in Applied Physics from the Universitat Politècnica de Catalunya (2003). He is currently a senior researcher with the Institut de Ciencies del Mar, CSIC, Barcelona. He is currently professor at the Remote Sensing and SIG master by the CREAF and Universitat Autònoma de Barcelona. His current research is focused on upper ocean dynamics and geophysical turbulence.
Sergio González-Motos
Sergio González-Motos was born on May 29, 1993, in Barcelona, Spain. He received the B.Sc. degree in Environmental Sciences from the University of Barcelona (2015) and the M.Sc. degree in Marine Biology: Biodiversity and Conservation from the University of La Laguna (2018). Currently he is working on his Ph.D. degree in the Institute of Marine Sciences (ICM-CSIC), Barcelona, studying marine microbial ecology, from communities to populations.
José Manuel Allegue
José Manuel Allegue was born, Santander (Spain) on january the 7th, 1966. He studied a degree in merchant marine at Cantabria University (Spain) and a Master degree in Civil Protection and Emergency Management from the Polytechnic University of Valencia (Spain). Professionally he developed his career as a merchant seaman and since 1999 in the Spanish Maritime Safety Agency, as Head of the Maritime Rescue Coordination Center in Almería (Spain).
Cristina González-Haro
Cristina González-Haro was born on 1979 in Barcelona, Spain. She has a multi-disciplinary background having studied Electronic and Telecommunications Engineering BsC (2004), having specialized in remote sensing of the ocean during her MSc (2010) and acquiring fundamental understanding of physical oceanography during her PhD (2015). She is currently a PostDoc researcher at the Institut de Ciències del Mar (ICM) in the Barcelona Expert Center (BEC) group. Her research activity is centered in Ocean Remote Sensing, with special emphasis in the exploitation of remote sensing data to study and investigate the dynamics of the ocean’s upper layers.
References
- Adani M, Dobricic S, Pinardi N. 2011. Quality assessment of a 1985–2007 mediterranean sea reanalysis. J Atmos Ocean Technol. 28(4):569–589.
- Alvarez I, Gomez-Gesteira M, deCastro M, Dias J. 2008. Spatiotemporal evolution of upwelling regime along the western coast of the Iberian Peninsula. J Geophys Res Oceans. 113. C07020.
- Alvarez I, Gomez-Gesteira M, deCastro M, Lorenzo M, Crespo A, Dias J. 2011. Comparative analysis of upwelling influence between the western and northern coast of the Iberian Peninsula. Cont Shelf Res. 31(5):388–399. Coastal processes in Northwestern Iberia, Spain.
- Alvarez I, Gomez–Gesteira M, deCastro M, Novoa E. 2008b. Ekman transport along the Galician Coast (NW, Spain) calculated from QuikSCAT winds. J Mar Syst. 72(1):101–115. Oceanography of the Bay of Biscay.
- Alvarez-Salgado XA. 2007. Contribution of upwelling filaments to offshore carbon export in the subtropical Northeast Atlantic ocean. Limnol Oceanogr. 52(3):1287–1292.
- Artegiani A, Paschini E, Russo A, Bregant D, Raicich F, Pinardi N. 1997. The adriatic sea general circulation. Part II: baroclinic circulation structure. J Phys Oceanogr. 27(8):1515–1532. https://journals.ametsoc.org/view/journals/phoc/27/8/1520-0485_1997_027_1515_tasgcp_2.0.co_2.xml.
- Astraldi M, Balopoulos S, Candela J, Font J, Gačić M, Gasparini G, Manca B, Theocharis A, Tintoré J. 1999. The role of straits and channels in understanding the characteristics of Mediterranean circulation. Prog Oceanogr. 44(1):65–108.
- Bakun A, Agostini VN. 2001. Seasonal patterns of wind-induced upwelling/downwelling in the mediterranean sea. Sci Mar. 65(3):243–257.
- Bojinski S, Verstraete M, Peterson T, Richter C, Simmons A, Zemp M. 2014. The concept of essential climate variables in support of climate research, applications, and policy. Bull Am Meteorol Soc. 95(9):1431–1443.
- Centurioni L, Horányi A, Cardinali C, Charpentier E, Lumpkin R. 2017. A global ocean observing system for measuring sea level atmospheric pressure: effects and impacts on numerical weather prediction. Bull Am Meteorol Soc. 98(2):231–238.
- Charria G, Lazure P, Le Cann B, Serpette A, Reverdin G, Louazel S, Batifoulier F, Dumas F, Pichon A, Morel Y. 2013. Surface layer circulation derived from Lagrangian drifters in the Bay of Biscay. J Mar Syst. 109-110:S60–S76.
- Ciappa A. 2014. The controversial path of Atlantic water in the eastern mediterranean. Prog Oceanogr. 123:74–83.
- Civile D, Lodolo E, Caffau M, Baradello L, Ben-Avraham Z. 2016. Anatomy of a submerged archipelago in the Sicilian Channel (central Mediterranean Sea). Geol Mag. 153(1):160–178.
- Cushman-Roisin B, Gačić M, Poulain P, Artegiani A. 2001. Toward the future. In: Poulain P, Cushman-Roisin B, Gačić M, Artegiani A, editors. Oceanography of the adriatic sea. Dordrecht: Springer.
- Deakin R. 2005. Notes on least squares. RMIT University. Technical report.
- Dobricic S, Pinardi N. 2008. An oceanographic three-dimensional variational data assimilation scheme. Ocean Model. 22(3):89–105.
- Eckman V. 1932. On an improved type of current meter. J Cons Int Explo Mer. 7:3–10.
- Escudier R, Clementi E, Omar M, Cipollone A, Pistoia J, Aydogdu A, Drudi M, Grandi A, Lyubartsev V, Lecci R, et al. 2020. Mediterranean sea physical reanalysis (CMEMS MED-currents) (Ver. 1) [dataset]. Copernicus Monitoring Environment Marine Service (CMEMS). http://doi.org/10.25423/CMCC/MEDSEA_MULTIYEAR_PHY_006_004_E3R1.
- Estournel C, Marsaleix P, Ulses C. 2021. A new assessment of the circulation of atlantic and intermediate waters in the eastern mediterranean. Prog Oceanogr. 198:102673.
- Gačić M, Borzelli GLE, Civitarese G, Cardin V, Yari S. 2010. Can internal processes sustain reversals of the ocean upper circulation? The ionian sea example. Geophys Res Lett. 37(9).L09608.
- García-Ladona E, Font J, del Río E, Julià A, Salat J, Chic O, Orfila A, Álvarez A, Basterretxea G, Vizoso G, et al. 2005. The use of surface drifting floats in the monitoring of oil spills. The Prestige case. In: Proceedings of the 19th Biennial International Oil Spill Conference (IOSC). Miami; Vol. CD-ROM:14718A.
- García-Ladona E, Salvador J, Fernández P, Pelegrí J, Elósegui P, Sánchez O, Jiménez Madrid J, Pérez F, Ballabrera J, Isern-Fontanet J, et al. 2015. 30 years of research and development of Lagrangian buoys at the Institute of Marine Sciences. Sci Mar. 80(S1):141–158.
- GCOS. 2011. Systematic observation requirements for satellite-based products for climate supplemental details to the satellite-based component of the implementation plan for the global observing system for climate in support of the UNFCCC: 2011 update. https://library.wmo.int/doc_num.php?explnum_id=3710.
- Gerin R, Poulain P, Taupier-Letage I, Millot C, Ben Ismail S, Sammari C. 2009. Surface circulation in the Eastern Mediterranean using drifters (2005–2007). Ocean Sci. 5:559–574.
- Gould W. 2001. Direct measurements of subsurface ocean currents: a success history. In: Deacon TRCSM, editor. Understanding the oceans: a century of ocean exploration. London: CRC Press, Taylor Francis; p. 173–192.
- Hamad N, Millot C, Taupier-Letage I. 2005. A new hypothesis about the surface circulation in the eastern basin of the mediterranean sea. Prog Oceanogr. 66(2):287–298. Mediterranean physical oceanography and biogeochemical cycles: mediterranean general circulation and climate variability.
- Hart-Davis M, Backeberg B, Halo I, van Sebille E, Johannessen J. 2018. Assessing the accuracy of satellite derived ocean currents by comparing observed and virtual buoys in the greater agulhas region. Remote Sens Environ. 216:735–746.
- Haynes R, Barton ED. 1990. A poleward flow along the atlantic coast of the Iberian Peninsula. J Geophys Res Oceans. 95(C7):11425–11441.
- Haynes R, Barton ED. 1991. Lagrangian observations in the Iberian coastal transition zone. J Geophys Res Oceans. 96(C8):14731–14741.
- Herrera J, Rosón G, Varela R, Piedracoba S. 2008. Variability of the western Galician upwelling system (NW Spain) during an intensively sampled annual cycle. An EOF analysis approach. J Mar Syst. 72(1):200–217. Oceanography of the Bay of Biscay.
- Hersbach H, Bell B, Berrisford P, Hirahara S, Horányi A, Muñoz-Sabater J, Nicolas J, Peubey C, Radu R, Schepers D, et al. 2020. The ERA5 global reanalysis. Quart J R Meteorol Soc. 146(730):1999–2049.
- Holloway G, Nguyen A, Wang Z. 2011. Oceans and ocean models as seen by current meters. J Geophys Res Oceans. 116, C00D08.
- Ioannou A, Stegner A, Le Vu B, Taupier-Letage I, Speich S. 2017. Dynamical evolution of intense ierapetra eddies on a 22 year long period. J Geophys Res Oceans. 122(11):9276–9298.
- Isern-Fontanet J, Ballabrera-Poy J, Turiel A, García-Ladona E. 2017. Remote sensing of ocean surface currents: a review of what is being observed and what is being assimilated. Nonlinear Process Geophys. 24(4):613–643.
- Isern-Fontanet J, García-Ladona E, Font J, García-Olivares A. 2006. Non-Gaussian velocity probability density functions: an altimetric perspective of the Mediterranean sea. J Phys Oceanogr. 36(11):2153–2164.
- Kim H-Y. 2013. Statistical notes for clinical researchers: assessing normal distribution (2) using skewness and kurtosis. Restor Dent Endod. 38(1):52–54.
- Knapp T. 2007. Bimodality revisited. J Mod Appl Stat Methods. 6(1):8–20.
- Laurindo L, Mariano A, Lumpkin R. 2017. An improved near-surface velocity climatology for the global ocean from drifter observations. Deep Sea Res I: Oceanogr Res Pap. 124:73–92.
- Lumpkin R, Johnson G. 2013. Global ocean surface velocities from drifters: mean, variance, el nino southern oscillation response, and seasonal cycle. J Geophys Res Oceans. 118(6):2992–3006.
- Lumpkin R, Pazos M. 2007. Measuring surface currents with surface velocity program drifters: the instrument, its data and some recent results. In: Griffa TOA, Kirwan AD jr., Rossby T, editors. Lagrangian analysis and prediction of coastal and ocean dynamics, LAPCOD. New York: Cambridge University Press; p. 39–67.
- Madec G and the NEMO team. 2016. NEMO ocean engine: version 3.6 stable. Note du Pole de modelisation, Institut Pierre-Simon Laplace N 27. Technical report. https://www.nemo-ocean.eu/wpcontent/uploads/NEMO_book.pdf.
- Malanotte-Rizzoli P, Artale V, Borzelli-Eusebi GL, Brenner S, Crise A, Gačić M, Kress N, Marullo S, Ribera d'Alcalà M, Sofianos S, et al. 2014. Physical forcing and physical/biochemical variability of the Mediterranean sea: a review of unresolved issues and directions for future research. Ocean Sci. 10(3):281–322.
- Martínez J, García-Ladona E, Ballabrera-Poy J. 2021. Atlas of Mediterranean and Canary/Iberian/Biscay Atlantic currents (Ver. 1.0) [dataset]. https://doi.org/10.20350/digitalCSIC/13834.
- Mason E, Colas F, Molemaker J, Shchepetkin A, Troupin C, McWilliams J, Sangrà P. 2011. Seasonal variability of the Canary current: a numerical study. J Geophys Res Oceans. 116. C06001.
- Mason E, Coombs S, Oliveira P. 2005. An overview of the literature concerning the oceanography of the eastern North Atlantic region.. Relat Cient Téc IPIMAR Série digital. 33:1–58.
- Maximenko N, Lumpkin R, Centurioni L. 2013. Ocean surface circulation. In: Siedler JGG, Griffies SM, Church JA, editors. Ocean circulation and climate. Academic Press. Chapter 12. p. 283–304. (International geophysics; vol. 103).
- Menna M, Poulain P-M, Zodiatis G, Gertman I. 2012. On the surface circulation of the Levantine sub-basin derived from Lagrangian drifters and satellite altimetry data. Deep Sea Res I: Oceanogr Res Pap. 65:46–58.
- Menna M, Suarez NR, Civitarese G, Gačić M, Rubino A, Poulain P-M. 2019. Decadal variations of circulation in the central mediterranean and its interactions with mesoscale gyres. Deep Sea Res II: Top Stud Oceanogr. 164:14–24. Revisiting the Eastern Mediterranean: recent knowledge on the physical, biogeochemical and ecosystemic states and trends (Volume I).
- Millot C. 1985. Some features of the Algerian current. J Geophys Res. 90(C4):7169–7176.
- Millot C. 1999. Circulation in the western mediterranean sea. J Mar Syst. 20(1):423–442.
- Millot C, Taupier-Letage I. 2005. Circulation in the mediterranean sea. In: Saliot A, editor. The Mediterranean sea. Berlin, Heidelberg: Springer. Chapter 12. p. 29–66. (Handbook of environmental chemistry; vol. 5K).
- Mkhinini N, Coimbra ALS, Stegner A, Arsouze T, Taupier-Letage I, Béranger K. 2014. Long-lived mesoscale eddies in the eastern mediterranean sea: analysis of 20 years of aviso geostrophic velocities. J Geophys Res Oceans. 119(12):8603–8626.
- Molcard A, Pinardi N, Iskandarani M, Haidvogel D. 2002. Wind driven general circulation of the mediterranean sea simulated with a spectral element ocean model. Dyn Atmos Oceans. 35(2):97–130.
- NGA. 2002. Atlas of pilot charts North Atlantic ocean (Including Gulf of Mexico). In: US atlas of pilot charts. Number 106. National Geospatial-Intelligence Agency. [accessed 2022 Mar 21].
- Niiler P. 2001. In: Siedler Gerold, Church John, Gould John, editors. Ocean Circulation and Climate. Observing and modelling the Global Ocean. Vol. 77. p. 193–204.
- Niiler P, Maximenko N, Panteleev G, Yamagata T, Olson D. 2003. Near–surface dynamical structure of the kuroshio extension. J Geophys Res: Oceans. 108(C6).3193.
- Notteboom T, Pallis A, Rodrigues J-P. 2021. Global maritime routes and chokepoints.
- Olita A, Ribotti A, Fazioli L, Perilli A, Sorgente R. 2013. Surface circulation and upwelling in the sardinia sea: a numerical study. Cont Shelf Res. 71:95–108. https://www.sciencedirect.com/science/article/pii/S0278434313003427.
- Olson D, Kourafalou V, Johns W, Samuels G, Veneziani M. 2007. Aegean surface circulation from a satellite-tracked drifter array. J Phys Oceanogr. 37(7):1898–1917.
- Pasquero C, Provenzale A, Babiano A. 2001. Parameterization of dispersion in two-dimensional turbulence. J Fluid Mech. 439:279–303.
- Peliz A, Dubert J, Santos A, Oliveira P, Le Cann B. 2005. Winter upper ocean circulation in the Western Iberian basin–fronts, eddies and poleward flows: an overview. Deep Sea Res I: Oceanogr Res Pap. 52(4):621–646.
- Pinardi N, Zavatarelli M, Adani M, Coppini G, Fratianni C, Oddo P, Simoncelli S, Tonani M, Lyubartsev V, Dobricic S, et al. 2015. Mediterranean sea large-scale low-frequency ocean variability and water mass formation rates from 1987 to 2007: a retrospective analysis. Prog Oceanogr. 132:318–332. Oceanography of the Arctic and North Atlantic basins.
- Poulain P-M, Barbanti R, Font J, Cruzado A, Millot C, Gertman I, Griffa A, Molcard A, Rupolo V, Le Bras S, et al. 2007. Medargo: a drifting profiler program in the mediterranean sea. Ocean Sci. 3(3):379–395.
- Poulain P-M, Bussani A, Gerin R, Jungwirth R, Mauri E, Menna M, Notarstefano G. 2013. Mediterranean surface currents measured with drifters: from basin to subinertial scales. Oceanography. 26:38–47.
- Poulain P-M, Menna M, Mauri E. 2012. Surface geostrophic circulation of the mediterranean sea derived from drifter and satellite altimeter data. J Phys Oceanogr. 42(6):973–990.
- Poulain P-M, Zambianchi E. 2007. Surface circulation in the central mediterranean sea as deduced from Lagrangian drifters in the 1990s. Cont Shelf Res. 27(7):981–1001.
- Preisendorfer R, Mobley C. 1988. Principal component analysis in meteorology and oceanography. Elsevier; Distributors for the U.S. and Canada, Elsevier Science Pub. Co.
- Provenzale A. 1999. Transport bu coherent barotropic vortices. Annu Rev Fluid Mech. 31(1):55–93.
- Pujol M-I, Mertz F. 2020. Mediterranean sea gridded L4 sea surface heights and derived variables reprocessed [dataset]. https://resources.marine.copernicus.eu/?option=com_csw&view=details&product_id=SEALEVEL_MED_PHY_L4_REP_OBSERVATIONS_008_051.
- Renault L, Oguz T, Pascual A, Vizoso G, Tintoré J. 2012. Surface circulation in the Alborán Sea (western Mediterranean) inferred from remotely sensed data. J Geophys Res. 117.C08009.
- Rio M-H, Mulet S, Picot N. 2014. Beyond GOCE for the ocean circulation estimate: synergetic use of altimetry, gravimetry, and in situ data provides new insight into geostrophic and Ekman currents. Geophys Res Lett. 41(24):8918–8925.
- Robinson AR, Golnaraghi M. 1993. Circulation and dynamics of the eastern mediterranean sea; quasi-synoptic data-driven simulations. Deep Sea Res II: Top Stud Oceanogr. 40(6):1207–1246.
- Robinson AR, Malanotte-Rizzoli P, Hecht A, Michelato A, Roether W, Theocharis A, Ünlüata Ü, Pinardi N, Artegiani A, Bergamasco A, et al. 1992. General circulation of the eastern mediterranean. Earth-Sci Rev. 32(4):285–309.
- Roether W, Manca BB, Klein B, Bregant D, Georgopoulos D, Beitzel V, Kovačević V, Luchetta A. 1996. Recent changes in eastern mediterranean deep waters. Science. 271(5247):333–335.
- Sánchez-Garrido J, García Lafuente J, Álvarez Fanjul E, Sotillo MG, de los Santos F. 2013. What does cause the collapse of the Western Alboran Gyre? Results of an operational ocean model. Prog Oceanogr. 116:142–153.
- Santos F, Gómez-Gesteira M, deCastro M, Álvarez I. 2011. Upwelling along the western coast of the Iberian Peninsula: dependence of trends on fitting strategy. Clim Res. 48:213–218.
- Smith S. 1997. The scientist and engineer's guide to digital signal processing. San Diego: California Technical Pub.
- Sotillo M, Álvarez E, Castanedo S, Abascal A, Menéndez J, Olivella R, García-Ladona E, Ruiz-Villareal M, Conde J, Gómez M, et al. 2008. Towards an operational system for oil spill forecast in the Spanishwaters: initial developments and implementation test.. Mar Pollut Bull. 4(56):686–703.
- Stewart K, Spence P, Waterman S, Sommer JL, Molines J, Lilly J, England M. 2015. Anisotropy of eddy variability in the global ocean. Ocean Model. 95:53–65.
- Storto A, Alvera-Azcárate A, Balmaseda MA, Barth A, Chevallier M, Counillon F, Domingues CM, Drevillon M, Drillet Y, Forget G, et al. 2019. Ocean reanalyses: recent advances and unsolved challenges. Front Mar Sci. 6.418.
- Storto A, Masina S, Navarra A. 2016. Evaluation of the CMCC eddy-permitting global ocean physical reanalysis system (C-GLORS, 1982–2012) and its assimilation components. Quart J R Meteorol Soc. 142(695):738–758.
- Tabure G, Pujol M-I. 2020. Quality information document for sea level TAC-DUACS products. http://marine.copernicus.eu/documents/QUID/CMEMS-SL-QUID-008-032-062.pdf.
- Tukey J. 1977. Exploratory data analysis. Reading, Massachusetts: Addison-Wesley.
- Tziperman E, Malanotte-Rizzol P. 1991. The climatological seasonal circulation of the mediterranean sea. J Mar Res. 49(3):411–434.
- West SG, Finch JF, Curran PJ. 1995. Structural equation models with nonnormal variables: problems and remedies. In: Hoyle RH, editor. Structural equation modeling: concepts, issues, and applications. New York: Sage Publications, Inc. Chapter 4. p. 67–75.
- Wilkinson M, Dumontier M, Aalbersberg I, Appleton G, Axton M, Baak A, Blomberg N, Boiten J-W, da Silva Santos L, Bourne P, et al. 2016. The FAIR guiding principles for scientific data management and stewardship. Sci Data. 3(1):160018.
- Woltmann R. 1790. Theorie und Gebrauch des hydrometrischen Flügels oder eine zuverlässige Methode die Geschwindigkeit der Winde und strömenden Gewässer zu beobachten. B.G. Hoffmann. https://books.google.fr/books?id=z2o_AAAAcAAJ&dq=reinhard+woltmann+1790&hl=fr&source=gbs_navlinks_s.
- World Tourism Organization. 2015. Mediterranean tourism trends. In: UNWTO World Tourism Barometer. 2015 ed. p. 1–12.
- Wyrtki K. 1960. On the presentation of ocean surface currents. Int Hydrogr Rev. 37(1):153–174.
- Zambianchi E, Trani M, Falco P. 2017. Lagrangian transport of marine litter in the Mediterranean sea. Front Environ Sci. 5(5):15 pp.
- Zhang C, Mapes B, Soden B. 2003. Bimodality in tropical water vapour. Quart J R Meteorol Soc. 129(594):2847–2866.