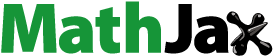
Abstract
Lactate, a main product of rumen microbial fermentation from dietary starch, has been recognised as an important mediator of rumen microbiota regulation in rumen acidosis. However, the mechanism of lactate transport and metabolism in the rumen remains unclear. This study aimed to evaluate the significance of the absorption, transport, and metabolism of lactic acid in the pH and LPS-induced subacute ruminal acidosis (SARA) model of rumen epithelial cells (RECs). In the current study, RECs were obtained from two adult Saanen goats (44.5 ± 4.6 kg BW) and using Lipopolysaccharide (LPS) and low pH to co-stimulate RECs to construct a microenvironment under SARA conditions, to further analyse the lactate transport and metabolism by RECs. The results showed that the LPS and low pH could significantly reduce cell viability by 29.36% (p < 0.05), induce inflammation in RECs, destroy tight junctions between RECs, and increase cell permeability. In addition, stimulation leads to changes in cellular metabolic activity and inhibits the expression of lactate transporters (p < 0.05), promoting an increased risk of lactate accumulation in the intracellular and intestinal lumen. This study hopefully provides a better understanding of lactate metabolic regulation and new perspectives for the nutritional prevention and treatment of SARA.
Lactic acid is an important fermentation product in the rumen of ruminants, and its transport and metabolic regulation mechanisms are still unclear.
To understand transport uptake in the rumen epithelium, a SARA model was therefore used.
Low pH and high LPS trigger epithelial inflammation, increasing the risk of lactic acid entering the bloodstream.
HIGHLIGHTS
Introduction
Ruminal acidosis is a common nutritional and metabolic disease of ruminants. It is well known that the imbalance of lactic acid metabolising flora in the rumen leads to a large accumulation of lactic acid in the rumen, causing the pH of the rumen’s internal environment to decrease, thereby destroying the structural integrity of the epithelium and inhibiting the growth of microorganisms, and affect the normal process of host metabolic function (Enemark et al. Citation2002; Xia et al. Citation2019). Lactic acid is an important intermediate in rumen microbial starch catabolism and plays an important role in rumen acidosis (Plaizier et al. Citation2008). Previous studies have pointed out that when the lactate concentration in the rumen is higher than 40 mM, the body will show severe acidosis (Owens et al. Citation1998). However, in modern intensive ruminant production, ruminants fed on high-grain diets were more prone from subacute rumen acidosis (SARA) than from rumen acidosis. It has been reported that the accumulation of lactic acid in the rumen is not evident, suggesting a metabolic mechanism for rapid clearance of lactic acid in the rumen (Counotte et al. Citation1981; Zhang et al. Citation2017). Notably, lactate formed in the rumen can be transported and absorbed through the rumen epithelium (Lettat et al. Citation2010). Therefore, understanding the metabolic process of lactic acid in the rumen is of great significance for regulating the occurrence and development of SARA (Halestrap and Meredith Citation2004). The link between rumen microbiota and metabolic health has been continuously demonstrated. However, the mechanisms of lactate transport and metabolism control in the rumen are poorly understood due to the complexity of metabolism. It should be pointed out that there is no single lactate transporter in the rumen epithelium, ruminal lactate relies on the co-transportation of monocarboxylate transporters (MCTs) with other monocarboxylates (Halestrap Citation2013). Emerging evidence suggests that monocarboxylate transporter 1 (MCT1) in the basolateral membrane is mainly responsible for the transport of lactate to the blood, while monocarboxylate transporter 4 (MCT4) and sodium-coupled monocarboxylate transporter (SMCT1) in the apical membrane are responsible for the transport from the lumen to the cells(Kirat et al. Citation2006; Steele et al. Citation2011). Thus, whether the rumen epithelial ability to absorb and transport lactate is affected by SARA is still a matter of concern. Furthermore, when high-grain diets are fed, the tight junctions of the rumen epithelium are disrupted, leading to impairment of barrier function, resulting in increased cellular permeability (Xie et al. Citation2014). Increased epithelial cell permeability through the deterioration of tight junctions during SARA also increases the transport of lactate from the gut lumen to the blood stream (Liu et al. Citation2013). Therefore, focusing on lactate uptake by the rumen epithelium under SARA may contribute to understanding the pathogenesis of SARA and its link to acidosis. In this experiment, two Saanen dairy goats were selected to prepare primary rumen epithelial cells (RECs), using LPS and pH to co-stimulate RECs to construct a microenvironment under the epithelial SARA conditions, and to determine the difference in lactate under SARA conditions by detecting absorption, transformation, transport, and metabolism. This study will provide a basis for further understanding lactate metabolism and its role in metabolic regulation the development rumen of acidosis.
Materials and methods
The experimental protocol was approved by the Animal Care and Use Committee of Yangzhou University. All animal experiments adhered to the animal experiment policy of Yangzhou University, China. (225009)
Animals and tissue collection
Tissues for this experiment were obtained from two adult non lactation Saanen goats (44.5 ± 4.6 kg of initial body weight), housed in slatted wood floor pens (1.5 × 2.5 m) with an automatic drinking bowl and feed chute and fed twice at 08:00 and 16:00 (600 g per feeding). The diet was formulated according to the recommendation of the National Research Council (NRC Citation2007) to meet the energy requirements of dairy goat. The chemical and ingredient compositions are shown in Table . Goats were granted free access to water during the experiment. After anaesthesia, the goats were bled to death at the jugular vein. The rumen was taken out and the rumen tissue was repeatedly washed with cold saline containing 5% penicillin-streptomycin mixture (Sodium penicillin G: 100 U/mL, streptomycin sulphate: 0.1 mg/mL) (Solebao P1400, Beijing, China). The serosa was bluntly stripped and placed in 5% penicillin- Streptomycin mixture and 0.25 mg/mL gentamicin in DMEM/F12 medium (Invitrogen, USA) and then quickly transferred to the laboratory (Kent-Dennis and Penner Citation2021).
Table 1. Ingredients and chemical composition of the experimental diet for goats.
Ruminal epithelial cell isolation and cultivation
The rumen epithelial cell layer and the muscle layer of the serosa layer of the rumen tissue were further separated, digested with trypsin-EDTA (0.25%) (Gibco, USA) at 37 °C for 5 min, repeated 7 times, and discarded after the first 3 digestions. The supernatant was removed, and the collected supernatant was filtered through a sterilised 200-mesh nylon mesh. After centrifugation at 106 × g for 5 min, the cell-containing pellet was washed three times with phosphate buffer saline (PBS), and resuspended in PBS. In complete medium containing DMEM/F12, 5% FBS (Invitrogen, USA), 2% penicillin-streptomycin mixture, 1 × ITS (Insulin, Transferrin, Selenium) (Gibco, USA) and 15 ng/mL Epidermal growth factor (EGF) (PeproTech, USA). After cell counting, the cells were seeded into 25 cm2 cell culture flasks (Corning, USA) at a density of 1 × 106 cells/mL. Subsequently, the cells were kept at 37 °C in a constant temperature and humidity containing 5% carbon dioxide incubator (Memelt INC108, Germany) for primary culture, the medium was changed every 2 days. When the cell confluence rate reached 85–95%, the primary cells were subcultured. The cell morphology was checked by an inverted phase contrast microscope (CKX41, Olympus, Japan) to observe.
Experimental design
In this experiment, the rumen epithelial cells were subjected to 10% HCl (vol/vol) Sinopharm Group; China) and LPS (E. Coli O55: B5, Sigma-Aldrich, USA) co-treated medium (pH 5.5, 10 μg/mL LPS) and were continuously cultured for 3 h to build a cell model of SARA. As a control, the rumen epithelial cells were cultured in in control DMEM/F12 medium (pH 7.2, no LPS) for the same period of time. To further investigate the effect of SARA on lactate transport in RECs, we washed the treated RECs three times with PBS (Solarbio, Beijing, China), and added complete media of 1 mM sodium L-lactate labelled with 3-13C (Sigma-Aldrich, USA). After culturing for 24 h, the cells and their culture medium were collected for subsequent determination of relevant indicators.
Cell immunofluorescence identification
The subcultured cells were digested from the culture flask using trypsin-EDTA (0.25%) (Gibco, USA) and the cell density was adjusted to seed a 6-well plate (Corning, USA) at a density of 1 × 106, and cultured in incubators for 24 h. Each treatment was repeated three times and the experiment was repeated three times. Then 1 mL of self-made cell fixative (acetone: methanol 1:1, Sinopharm Group, China) was added to each well and incubate at 4 °C for 30 min. Afterward, the plate washed three times with PBS and 1 mL of 3% FBS-containing PBS solution was added to each well, and incubate at room temperature for 30 min. The solution of plate was discard and 500 μL of CK18 antibody (1:100 dilution, ab668, Abcam, USA) was added to incubate overnight at 4 °C. After washing three times with PBS, 500 μL of FITC-goat anti-mouse IgG (1:100 dilution, Boster, Wuhan, China) was added and incubated at room temperature in the dark for 1 h. After discarding the FITC-labelled fluorescent secondary antibody, the plate washed three times with PBS and 500 μL of DAPI (Abcam, USA) was added to stain the nucleus for 7 min. Finally, the staining solution was discarded and washed three times with PBS, and observed under an inverted fluorescent microscope (Olympus IX73, Olympus, Japan). The excitation wavelength of DAPI is 358 nm and the emission wavelength is 461 nm, while the excitation wavelength of FITC is 495 nm and the emission wavelength is 519 nm. The same method of sampling was used to localise and express the tight junction proteins Claudin-1 and ZO-1 in rumen epithelial cells.
Cell viability assay
The cells were placed in a 96-well plate (Corning, USA), seeded at a density of 15,000 cells per well, and the culture volume was 100 μL, The CELL viability was determined by CELL TITER-GLO (CTG) luminescence method after 24 h of cell adhesion growth. The specific operation is as follows: after thawing the CTG-Glo2.0 Assay (Promega, USA), equilibrate at room temperature for 30 min, take out the 96-well plate from the incubator, equilibrate at room temperature for 10 min, add to each well of the 96-well plate. After mixing, 100 uL of CellTiter-Glo reagent was gently shaken on a destaining shaker for 2 min, and then allowed to stand for 10 min. Finally, the luminescence intensity was measured on a SpectraMax® M5 (Molecular Devices, USA) multifunctional microplate reader. In the experiment, 3 replicates were set for each treatment, and the entire experiment was replicated 3 times.
Determination of lactate and lactate dehydrogenase
The treated cell culture supernatant was collected, digested with trypsin, and the cell pellet was collected by centrifugation, resuspended in PBS and used a VCX-130 sonicator (Sonics, UK) with pulses on for 30 s and pulses off for 30 s. the parameters of the power 100 W, after 10 min of continuous disruption, collect the cell disruption liquid. The protein concentration was determined by BCA protein detection kit (Beyotime, China), lactate concentrations of cell culture supernatant were measured using a lactate Assay Kit (Jiancheng Bioengineering Institute, Nanjing, China) according to the manufacturer’s instructions protocol. LDH (Lactate dehydrogenase) release of cell culture supernatant was detected using a LDH kit (Jiancheng Bioengineering Institute, Nanjing, China).
Determination of transmembrane resistance and membrane permeability
The integrity of the monolayer was measured by measuring transmembrane electrical resistance (TEER) and paracellular permeability (Watson et al. Citation2013). RECs were seeded on top of Transwell cell culture inserts loaded with polycarbonate membranes (Growth area 1.12 cm2, diameter 12 mm, pore diameter 0.4 μm, thickness 10 μm, Corning, USA). At the same time, 1.5 mL of medium was added to the lower layer. After the cells adhered, the cell resistance metre (Millicell ERS-2, USA) was used to measure the transmembrane potential (TEER) every 24 h until the TEER resistance value stabilised, and the resistance difference between the upper layer (RTOTAL) and the bottom layer (RBLANK) of Transwell cell culture inserts was recorded and expressed in units Ωcm2. The resistance value of the cells in the test was calculated according to the following formula (Wengst and Reichl Citation2010):
Cell permeability was measured using FITC-dextran (FD-40) (Kim et al. Citation2012). The culture medium on the upper side of the treated Transwell chamber was removed, 0.5 mL of 0.5 mg/mL FD-40 (Chondrex, USA) was added, and 1.5 mL of cell culture medium was added to the outer side of the lower chamber. Cells were then cultured for 24 h. 100 μL of medium was removed from the lower chamber, placed in a 96-well plate, and the fluorescence intensity was detected on a SpectraMax® M5 multifunctional microplate reader with an excitation wavelength of 490 nm and an emission wavelength of 520 nm (Kent-Dennis et al. Citation2019).
RNA extraction and real-time quantitative PCR
At the end of the incubation, and the medium was discarded after the treatment using a phenol-chloroform extraction (Invitrogen, USA) for phase separation, followed by double precipitations with ice-cold isopropa-nol (Thomas et al. Citation1961). Purity, concentration, and quality of RNA were determined using NanoDrop 1000 (NanoDrop Technologies, Wilmington, DE, USA). RT-qPCR was performed using SuperReal PreMix Colour (SYBR Green) (FP171207, Tiangen Biotech, Beijing, China) on an ABI 7500 real-Time PCR system (Applied Biosystems, USA) following the manufacturer’s instructions. The internal reference gene was β-actin. PCR amplification conditions were as follows: pe-denaturation at 95 °C for 15 min followed by 40 cycles of 95 °C for 10 sec and 60 °C for 32 sec. Primers used are listed in Table . PCR primers were designed using Primer-Blast and relative quantification analysis was performed by the 2-ΔΔCT method.
Table 2. Primer sequences of rumen microbiota for RT-RT-qPCR.
13C NMR spectrometry measurement
After the cells were stimulated by pH and LPS, the medium containing 1 mM 3-13C-labelled sodium L-lactate was replaced and cultured for 24 h. The cell culture broth was collected and separated by centrifugation at 10,000 × g for 10 min, and the supernatant was filtered by 0.22 μm membrane for determination of lactate conversion. 3 mL of prepared supernatant was acidified (pH = 2.0) by using (6.38 mol/L) potassium phosphate buffer (Aschenbach and Gäbel Citation2000). 800 μL of the above solution was transferred to a tube containing 200 μL of D2O. 13C-NMR spectra were recorded at 125 MHz using a Bruker AvanceII 500-MHz superconducting spectrometer (BrukerBiospin, Bern, Switzerland) equipped with a dual 13C/1H probe, and 83.6 µmol/L methanol-containing D2O solution was used as an external standard for quantification. Chemical shift of organic acids was measured based on past reports, and MestRe-C software (version 4.9.9.9, Mestrelab Research, Santiago de Compostela, Spain) was used for peak detection and peak area integration.
Statistical analysis
Data were subjected to independent-samples t-test using SPSS 22.0 (IBM, USA). Origin software (version 2020b, OriginLab Corp) was used to make the graphics. Spectra were processed using the MestRe-C 4.9.9.9 software (Universidad de Santiago de Compostela, Spain). Data were expressed as mean ± standard error of the mean (SEM). p-Values less than 0.05 indicated significant differences.
Results
Cell viability and inflammatory gene expression
The immunofluorescence results of primary cultured goat rumen epithelial cell-specific keratin CK18 (Cytokeratin 18) are shown in Figure . In order to construct the SARA model of cells, we co-stimulated the cells with LPS and pH for 3 h, the expression of inflammatory gene mRNA of major rumen epithelium cells was determined via qPCR. As shown in Figure . Specifically, LPS and pH stimulation significantly increased the expression of TLR-4 (Toll -Like Receptor 4), IL-1 (Interleukin-1), IL-6 (Interleukin-6) and TNF-ɑ (Tumour Necrosis Factor Alpha) in the rumen epithelial cells. This indicated that the SARA cell model we constructed successfully induced the expression of intracellular inflammatory response. In addition, the number of cells was significantly decreased after LPS and pH treatment (Figure ). The cell inhibition rate was 29.36%.
Figure 1. Identification and morphology of primary rumen epithelium cells (100 μm:100 ×). Note: (A) The nuclei were counterstained using DAPI. (B) Cytoplasm were counterstained using FITC-labelled CK18. (C) Merge of DAPI and CK18. (D) The morphology of primary rumen epithelium cell at 8 days of cultured. n = 3 RECs for each treatment.

Figure 2. Effects of SARA and pH on cell viability and inflammatory gene expression in rumen epithelial cells. Data were expressed as mean ± SEM (n = 3). Note: (A) Effects of LPS and pH in viability of rumen epithelial cells. CON, DMEM/F12 medium cultured for 3 h; LPS, DMEM/F12 supplemented with 10 μg/mL LPS and adjusted pH to 5.6 cultured for 3 h. (B) Effect of LPS and pH in inflammatory genes expression of rumen epithelium cells. Significant differences are denoted by *(p < 0.05), and extremely significant differences are indicated by **(p < 0.01).

The change of tight junction proteins
The effects of LPS and pH stimulation on tight junction proteins gene was shown in Figure . The results showed that the LPS and pH treatment significantly reduced the mRNA expression of tight junction-related proteins Claudin-1 and ZO-1 (p < 0.05), while there was no significant difference in Claudin-4 and Occludin between the two groups. From the results of cell immunofluorescence, Claudin-1 and ZO-1 were positively expressed on the membranes of rumen epithelial cells that were not treated with LPS and pH, but were weakly expressed after LPS and pH treatment. This suggests that our treatment may have disrupted the integrity of the rumen epithelium (Figure ).
Figure 3. Effect of SARA and pH in expression of tight junction related genes on rumen epithelium cells Data were expressed as mean ± SEM (n = 3). Note: Significant differences are denoted by *(p < 0.05).

Figure 4. Effect of LPS and pH in immunofluorescence of tight junction on rumen epithelium cells (100 μm 100 ×). Note: The green fluorescence was FITC labelled protein antibody, the blue fluorescence was DAPI labelled nucleus, and the white arrow was the expression site of the protein. n = 3 RECs for each treatment.

Cell membrane integrity
The integrity of the cell membrane is measured by transmembrane resistance and cell permeability. As shown in Figure , the transcellular membrane resistance of the SARA group was not significantly different from that of the CON group (p > 0.05); but the cell permeability results showed in Figure that the cell permeability was significantly increased after LPS and pH treatment (p < 0.05).
Figure 5. Effects of SARA and pH on integrity of rumen epithelial cell membrane. Data were expressed as mean ± SEM (n = 3). Note: (A) Effect of LPS and pH in trans-epithelial electrical resistance on rumen epithelium cells. (B) Effect of LPS and pH in membrane permeability on rumen epithelium cells. Significant differences are denoted by *(p < 0.05).

Absorption of lactic acid by rumen epithelial cells
In order to observe the metabolic status of the cells, the lactic acid in the culture medium was measured under the same number of cells, and the results are shown in Figure . Compared with the CON group, the LPS and pH treatment significantly decreased the concentration of lactic acid in the culture medium (p < 0.05), which indicated that the treatment caused changes in cellular metabolism. Due to lactic acid exist in the form of a product in cells, the absorption of lactic acid in epithelial cells was detected, and the results are shown in Table . The results showed that epithelial cells could metabolise part of exogenously added lactic acid, and the lactic acid consumption of cells was significantly reduced after LPS and pH treatment (p < 0.01). The results showed that epithelial cells could metabolise part of exogenously added lactic acid, and the lactic acid consumption of cells was significantly reduced after LPS and pH treatment (p < 0.01). However, the cell viability after treatment was significantly decreased (p < 0.05), and the cell inhibition rate was 19.23%. The calculation showed that when the number of cells was the same, there was no significant difference in the consumption of exogenous lactate between treatments. The concentration of lactic acid and the activity of LDH in the cells and in the medium after 24 h of continuous culture with lactic acid are shown in Table . There was no significant change of LDH in cell culture medium. However, LPS and pH treatment significantly increased LDH activity in rumen epithelial cells (p < 0.05). while there was no significant difference between lactate concentrations in rumen epithelial cells, but there was an increasing trend (p < 0.01). In addition, the lactate concentration in the cell culture medium after LPS and pH treatment was significantly lower than that in the CON group (p < 0.05). The effects of lactate addition on lactate transport and metabolism-related gene expression under the stimulation of LPS and pH were shown in Figure . The results showed that the gene expressions of MCT1 and MCT4 in the LPS group were significantly lower than that in the CON group (p < 0.05), while the LDH-A gene expression was significantly higher than that in the CON group (p < 0.05). There was no significant difference in the gene expression of SMCT1 between the two groups.
Figure 6. Effect of SARA and pH in lactate transport on rumen epithelium cells supplemented with lactate. Data were expressed as mean ± SEM (n = 3). Note: (A) Effect of LPS and pH in lactate concentration on cell culture. (B) Effect of LPS and pH in lactate concentration and Lactate dehydrogenase activity on rumen epithelium cells supplemented with lactate. Significant differences are denoted by *(p < 0.05), and extremely significant differences are indicated by **(p < 0.01).

Table 3. Effect of SARA and pH in cell number and lactate concentration and Lactate dehydrogenase activity on rumen epithelium cells supplemented with lactate.
Discussion
The rumen epithelium, an important component of the ruminants energy metabolism, is also an important biological barrier for ruminants to resist foreign invasion, the normal functioning of the rumen epithelium is crucial to host health. A large number of studies have shown that the occurrence of SARA is closely linked the release of LPS in the rumen and the increase of the inflammatory response (Kleen et al. Citation2003; Khafipour et al. Citation2009; Kent-Dennis et al. Citation2020). A recent study showed that RECs exposed to LPS stimulation induced changes in inflammatory genes, which were affected by LPS dosis, stimulation time and intensity (Plaizier et al. Citation2012). In addition, Nuclear Transcription Factor-kappa B (NF-κB) has been extensively studied as a LPS-dominated inflammatory response signaling pathway (Zhao et al. Citation2018). To further understand the effect of SARA on RECs, we combined with previous reports and treated cells with mixed stimulation (LPS concentration 10 μg/mL, pH 5.5) for 3 h (Fabre et al. Citation2007). In our study, we found that the co-stimulation not only reduced the activity of rumen epithelial cells, but also activated the TLR4 mediated NF-κB signalling pathway and induced the expression of pro-inflammatory factors TNF-ɑ, IL-1 and IL-6 (Liu et al. Citation2013). This suggested that our stimulation model successfully enhanced inflammatory responses in epithelial cells and can be used to simulate the challenges experienced by the rumen epithelium during SARA. Due to the complex internal environment in the rumen, the absorption of nutrients from the rumen epithelium (e.g. short-chain fatty acids (SCFAs)) is mainly achieved through transcellular pathways. However, the presence of an acidic environment and high concentrations of LPS makes the rumen epithelial mucosa more permeable and susceptible to damage. Therefore, the extravasation of lactate in the intestinal lumen through the epithelial barrier into the blood is also worthy of attention. Tight junctions (Claudin-1, Claudin-4, and Occludin) and their related proteins (ZO-1), as important components of the cytoskeleton, play an important role in paracellular pathways. Consistent with previous reports that SARA causes a decrease in the expression of tight junction proteins, pH and LPS stimulation also decreased the expression of Claudin-1 and ZO-1 in this experiment, suggesting that the integrity of the epithelium may be damaged (Sun et al. Citation2017). Measurement of transcellular membrane resistance and labelling flux as a common measure of epithelial integrity and permeability (Rémond et al. Citation1995). In this experiment, the intercellular flux of FITC-labelled dextran was significantly increased after pH and LPS co- stimulation, indicating an increase in cell permeability. TEER is closely related to the tight junctions between epithelial cells. We did not observe significant changes in TEER in this experiment. This may be related to the adaptive repair of tight junctions after cell stimulation is weakened, which leads to the increase of TEER.
Notably, for ruminants, SCFAs often serve as the main source of energy substrates for RECs (Jayasuriya and Hungate Citation1959). In vitro, the rumen epithelium is powered by glucose in the medium, so lactic acid is eliminated from the cell and accumulates in the cell fluid as the end product of glucose metabolism. The results showed that the concentration of lactic acid in the cell culture solution was significantly decreased after the treatment of pH and LPS, which suggested that the metabolic function of cells or the epithelial transport of lactic acid might be inhibited during this period. Therefore, we continued to incubate RECs with 1 mM 3-13C-labelled sodium L-lactate (Williams and Mackenzie Citation1965). Similar to previous findings, the viability of RECs was adaptively restored with the renewal of the culture system, indicating that the effects of short-term LPS and pH on cells were reversible (Plaizier et al. Citation2012). The pH and LPS stimulation did not cause changes in lactate consumption in this experiment and this may be related to the inefficient consumption of exogenous lactate in RECs (Chatham et al. Citation2001). Therefore, the isotope tracer of 13C in this experiment did not detect substances other than 3-13C-labelled sodium L-lactate, which may be related to the involvement of lactate in the energy metabolism of cells, which means both complex products in the metabolic pool and the concentration threshold of the detection line result in the absence of markers (Legrand et al. Citation1992). The LDH in cell culture is closely related to the degree of cell membrane damage (Jurisic et al. Citation2015). LPS and pH co- stimulation resulted in a decrease in cell viability, but have no effect on the LDH activity in the cell culture medium, which may be related to the intensity of stimulation used in our experiments. In addition, co-stimulation caused an increase in the LDH activity in REC and activated the LDH-A gene expression, which plays a role in the conversion of pyruvate to lactate using NADH as a cofactor (Aschenbach et al. Citation2009). These findings suggested that the LPS and pH stimulation alter the metabolic activity of cells and increased the risk of lactate accumulation in RECs.
The expression of the apical transporter of RECs is the key to the transmembrane transport of lactate. Rumen microbiota-produced lactate is rapidly absorbed across the rumen epithelium cells via the Na+ –coupled or H+ – coupled transporters. Some studies have shown that the expression of MCTs increased with the increase of the level of energy supply, and high concentrations of SCFA and LPS can inhibit the expression of MCT1 and SMCT1 (Halestrap and Meredith Citation2004; Liu Citation2013). In our experiment, we observed a decrease in the expression of MCT1 and MCT4 under mixed stimulation of LPS and pH, this means that lactate transport in the RECs may be inhibited under SARA conditions. Notably, although reduced uptake of lactate by the epithelium may have occurred, the lower rate of absorption made the effect less pronounced than that of SCFAs. In addition, LPS and pH stimulation increased the risk of intracellular lactate accumulation, and the decreased expression of MCT1 during this period exacerbated the possibility of intracellular lactate accumulation.
Conclusion
In conclusion, the SARA model constructed by low pH and high LPS concentration inhibited the transport of lactate on rumen epithelial cells via several pathways. On the one hand, the stimulation of low pH and high LPS concentration changed the fate of cell metabolism and increased the risk of lactate accumulation in the rumen. On the other hand, this SARA model can induce inflammation in disrupt tight junctions, and increase cell permeability which increased the risk of lactic acid infiltrated into the bloodstream via subepithelial. These findings provided information on the utilisation of lactate by rumen epithelial cells in the pH and LPS -induced model of SARA enhancing our understanding of SARA development. However, further studies should focus on whether the production of SCFAs in the rumen could change the transport of lactate in the rumen which is important in comprehensive understanding of lactate metabolism in rumen epithelial cells under SARA condition.
Author contributions
The contribution of the authors were as follows; conceptualisation of experiment and funding acquisition, HRW, laboratory analysis; BLH, YTF, XJZ writing of original draft; BLH, review and editing; HRW and YTF. All authors have read and approved the final manuscript.
Acknowledgements
The authors would like to acknowledge Prof. Hongrong Wang from Yangzhou University for his help and advice in the design of the study and laboratory analysis of the samples and thank the laboratory staff in the Laboratory of Metabolic Manipulation of Herbivorous Animal Nutrition for their assistance.
Disclosure statement
No potential conflict of interest was reported by the author(s).
Data availability statement
All data generated and/or analysed during this study are available from the corresponding author upon reasonable request.
Additional information
Funding
References
- Aschenbach J, Gäbel G. 2000. Effect and absorption of histamine in sheep rumen: significance of acidotic epithelial damage. J Anim Sci. 78(2):464–470.
- Aschenbach JR, Bilk S, Tadesse G, Stumpff F, Gäbel G. 2009. Bicarbonate-dependent and bicarbonate-independent mechanisms contribute to nondiffusive uptake of acetate in the ruminal epithelium of sheep. Am J Physiol Gastrointest Liver Physiol. 296(5):G1098–G1107.
- Chatham JC, Rosiers CD, Forder JR. 2001. Evidence of separate pathways for lactate uptake and release by the perfused rat heart. Am J Physiol Endocrinol Metab. 281(4):E794–E802.
- Counotte G, Prins R, Janssen R, Debie M. 1981. Role of Megasphaera elsdenii in the fermentation of DL-[2-13C] lactate in the rumen of dairy cattle. Appl Environ Microbiol. 42(4):649–655.
- Enemark JMD, Jorgensen RJ, Enemark PS. Rumen acidosis with special emphasis on diagnostic aspects of subclinical rumen acidosis: a review. Veterinarija ir zootechnika. 2002;20(42):16–29.
- Fabre C, Carvalho G, Tasdemir E, Braun T, Adès L, Grosjean J, Boehrer S, Métivier D, Souquère S, Pierron G, et al. 2007. NF-kappaB inhibition sensitizes to starvation-induced cell death in high-risk myelodysplastic syndrome and acute myeloid leukemia. Oncogene. 26(28):4071–4083.
- Halestrap AP. 2013. Monocarboxylic acid transport. Compr Physiol. 3(4):1611–1643.
- Halestrap AP, Meredith D. 2004. The SLC16 gene family—from monocarboxylate transporters (MCTs) to aromatic amino acid transporters and beyond. Pflugers Arch. 447(5):619–628.
- Jayasuriya G, Hungate RE. 1959. Lactate conversions in the bovine rumen. Arch Biochem Biophys. 82(2):274–287.
- Jurisic V, Radenkovic S, Konjevic G. 2015. The actual role of LDH as tumor marker, biochemical and clinical aspects. Adv Exp Med Biol. 2015;867:115–124.
- Kent-Dennis C, Penner GB. 2021. Effects of a proinflammatory response on metabolic function of cultured, primary ruminal epithelial cells. J Dairy Sci. 104(1):1002–1017.
- Kent-Dennis C, Aschenbach J, Griebel P, Penner G. 2020. Effects of lipopolysaccharide exposure in primary bovine ruminal epithelial cells. J Dairy Sci. 103(10):9587–9603.
- Kent-Dennis C, Pasternak A, Plaizier J, Penner G. 2019. Potential for a localized immune response by the ruminal epithelium in nonpregnant heifers following a short-term subacute ruminal acidosis challenge. J Dairy Sci. 102(8):7556–7569.
- Khafipour E, Krause D, Plaizier J. 2009. A grain-based subacute ruminal acidosis challenge causes translocation of lipopolysaccharide and triggers inflammation. J Dairy Sci. 92(3):1060–1070.
- Kim HJ, Huh D, Hamilton G, Ingber DE. 2012. Human gut-on-a-chip inhabited by microbial flora that experiences intestinal peristalsis-like motions and flow. Lab Chip. 12(12):2165–2174.
- Kirat D, Masuoka J, Hayashi H, Iwano H, Yokota H, Taniyama H, Kato S. 2006. Monocarboxylate transporter 1 (MCT1) plays a direct role in short‐chain fatty acids absorption in caprine rumen. J Physiol. 576(Pt 2):635–647.
- Kleen J, Hooijer G, Rehage J, Noordhuizen J. 2003. Subacute ruminal acidosis (SARA): a review. J Vet Med A Physiol Pathol Clin Med. 50(8):406–414.
- Legrand C, Bour J, Jacob C, Capiaumont J, Martial A, Marc A, Wudtke M, Kretzmer G, Demangel C, Duval D. 1992. Lactate dehydrogenase (LDH) activity of the cultured eukaryotic cells as marker of the number of dead cells in the medium. J Biotechnol. 25(3):231–243.
- Lettat A, Noziere P, Silberberg M, Morgavi D, Berger C, Martin C. 2010. Experimental feed induction of ruminal lactic, propionic, or butyric acidosis in sheep. J Anim Sci. 88(9):3041–3046.
- Liu J-H, Xu T-T, Liu Y-J, Zhu W-Y, Mao S-Y. 2013. A high-grain diet causes massive disruption of ruminal epithelial tight junctions in goats. Am J Physiol Regul Integr Comp Physiol. 305(3):R232–R241.
- Liu Z. 2013. Regulation of monocarboxylate transporter 1 (MCT1) by protein kinase A (PKA), The canonical Wnt/beta-catenin, notch signaling pathways, and by A MCT metabolic blocker.
- NRC. 2007. Nutrient requirements of small ruminants: sheep, goats, cervids, and new world camelids. Washington (DC): National Academic Press.
- Owens F, Secrist D, Hill W, Gill D. 1998. Acidosis in cattle: a review. J Anim Sci. 76(1):275–286.
- Plaizier J, Khafipour E, Li S, Gozho G, Krause D. 2012. Subacute ruminal acidosis (SARA), endotoxins and health consequences [J]. Anim Feed Sci Technol. 172(1–2):9–21.
- Plaizier JC, Krause DO, Gozho GN, Mcbride BW. 2008. Subacute ruminal acidosis in dairy cows: the physiological causes, incidence and consequences. Vet J. 176(1):21–31.
- Rémond D, Ortigues I, Jouany JP. 1995. Energy substrates for the rumen epithelium. Proc Nutr Soc. 54(1):95–105.
- Steele MA, Croom J, Kahler M, Alzahal O, Hook SE, Plaizier K, Mcbride BW. 2011. Bovine rumen epithelium undergoes rapid structural adaptations during grain-induced subacute ruminal acidosis. Am J Physiol Regul Integr Comp Physiol. 300(6):R1515–R1523.
- Sun K, Lei Y, Wang R, Wu Z, Wu G. 2017. Cinnamicaldehyde regulates the expression of tight junction proteins and amino acid transporters in intestinal porcine epithelial cells. J Animal Sci Biotechnol. 8(1):1–8.
- Thomas JW, Moore LA, Sykes JF. 1961. Further comparisons of alfalfa hay and alfalfa silage for growing dairy heifers. J Dairy Sci. 44(5):862–873.
- Watson PMD, Paterson JC, Thom G, Ginman U, Lundquist S, Webster CI. 2013. Modelling the endothelial blood-CNS barriers: a method for the production of robust in vitro models of the rat blood-brain barrier and blood-spinal cord barrier. BMC Neurosci. 14(1):1–21.
- Wengst A, Reichl S. 2010. RPMI 2650 epithelial model and three-dimensional reconstructed human nasal mucosa as in vitro models for nasal permeation studies. Eur J Pharm Biopharm. 74(2):290–297.
- Williams VJ, Mackenzie DDS. 1965. The absorption of lactic acid from the reticulo-rumen of the sheep. Aust J Biol Sci. 18(4):917–934.
- Xia G, Zhao F, Wang H. 2019. Advances in lactic acids metabolism in rumen and regulation of ruminal acidosis for ruminants. Chinese J Anim Nutr. 31(4):1511–1517.
- Xie S, Chen M, Yan B, He X, Chen X, Li D. 2014. Identification of a role for the PI3K/AKT/mTOR signaling pathway in innate immune cells. PLOS One. 9(4):e94496.
- Zhang R, Ye H, Liu J, Mao S. 2017. High-grain diets altered rumen fermentation and epithelial bacterial community and resulted in rumen epithelial injuries of goats. Appl Microbiol Biotechnol. 101(18):6981–6992.
- Zhao C, Liu G, Li X, Guan Y, Wang Y, Yuan X, Sun G, Wang Z, Li X. 2018. Inflammatory mechanism of Rumenitis in dairy cows with subacute ruminal acidosis. BMC Vet Res. 14(1):1–8.