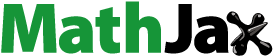
Abstract
This work aimed to investigate the effect of energy nature (lipids vs. carbohydrates) on enteric methane emission (eCH4) and performance in dairy cows fed grass silage-based diets. Eight multiparous Holstein cows were used in a 4 × 4 Latin square design, with 4 experimental periods of 28 days each. Cows were fed with 4 iso-energetic diets based on grass silage and supplemented with different levels of rapeseed oil (RO) (0, 1.5, 3.0, 4.5% of dietary dry matter (DM), Control, RO-low, RO-medium, RO-high diets, respectively) in substitution of starch from concentrate. Dairy performance, total-tract digestibility, and rumen parameters were measured when animals were in individual respiration chambers for quantifying eCH4. Intake of DM decreased with RO-high compared to other diets. Methane emissions were lowered similarly with all diets containing RO compared to Control (on average, -19% in g/d, −13% in g/kg DMI, −21% in g/kg milk). Ruminal propionate proportion was higher, whereas that of butyrate was lower with RO-high compared to the other diets. Milk yield was higher with RO-low and RO-medium, and was lower for RO-high compared to Control. Milk C16:0 concentration was lower, and C18:1c9, C18:1t11, and other rumen biohydrogenation intermediate concentrations were higher with diets containing RO compared to Control. The shift from the C18:1t11 to C18:1t10 biohydrogenation pathway is in agreement with the milk fat depression observed with RO-medium and RO-high. The inclusion of RO at 1.5% was the best compromise between eCH4, feed efficiency, and milk nutritional quality in cows fed grass silage-based diets.
HIGHLIGHTS
Energy substitution of carbohydrate (mainly starch) by rapeseed oil reduced methane emissions in dairy cows fed grass silage-based diets (up to –23% in g/d)
Rapeseed oil content above 3% induced milk fat depression and impaired milk fatty acid profile
The inclusion of 1.5% rapeseed oil was the best compromise to reduce methanogenesis (–15% in g/d) and improve dairy performance and milk nutritional quality
Introduction
Greenhouse gas emissions from agriculture increased by 16% between 2000 and 2017 and enteric methane emissions (eCH4) from cattle account for about 40% of these emissions (FAO, Citation2020). One of the challenges of livestock production systems is to find solutions to reduce eCH4 while maintaining the efficiency of animal production, in order to meet the quantity and quality of animal products for human nutrition needs (Hristov et al. Citation2013).
Among different solutions to mitigate eCH4 in dairy farming systems, nutritional strategies are quite efficient and readily applicable (Beauchemin et al. Citation2020; Arndt et al. Citation2022). Replacing carbohydrates (cellulose, hemicellulose, starch) in the diet with lipids, is an interesting eCH4-mitigating nutritional solution (Martin et al. Citation2010; Beauchemin et al. Citation2020). In addition, lipid supplementation of cow diets can greatly modify the fatty acid (FA) composition of milk, either through the production of polyunsaturated FA (PUFA) intermediates during the ruminal biohydrogenation process, or at the level of de novo synthesis of FA in milk via the decrease in this synthesis due to an inhibitory effect by the dietary PUFA (Chilliard et al. Citation2007; Kliem and Shingfield Citation2016).
Among different lipid sources, rapeseed represents an interesting option, as it reduces eCH4 in dairy cows (Brask et al. Citation2013; Bayat et al. Citation2018) and produces high concentrations of milk FA of nutritional interest (oleic acid, C18:3n-3, CLAc9t11 and monounsaturated FA (MUFA)), and reduces saturated FA (SFA) concentration compared to palm oil (Kliem and Shingfield Citation2016; Givens et al. Citation2009; Bernard et al. Citation2021). The effectiveness in mitigating eCH4 and modulating milk FA profile of rapeseed changes according to the form of presentation (Givens et al. Citation2009). Rapeseed oil (RO) seems to be more effective than whole, crushed or milled rapeseed at a similar lipid dose in modifying milk FA profile (Hoffmann et al. Citation2016; Kliem and Shingfield Citation2016; Givens et al. Citation2009). However, RO depending on the level of supplementation also can induce milk fat depression (MFD), especially when added to starch-rich diets (Hoffmann et al. Citation2016; Bougouin et al. Citation2019). Rapeseed oil can also increase some FA susceptibility to be undesirable for human health, like C18:1t10 and t FA especially with corn silage compared to grass silage-based diets (Givens et al. Citation2009; Bayat et al. Citation2018; Kliem et al. Citation2019). To the best of our knowledge, few studies have tested the effect of RO inclusion in dairy cow diets on both milk FA (i.e. Hoffmann et al. Citation2016; Givens et al. Citation2009) and eCH4 (Brask et al. Citation2013; Bayat et al. Citation2018). However, no research has been conducted on iso-energetic diets to find the best compromise between the positive effects of lipid inclusion as alternative energy source to carbohydrate on digestive processes, including mitigation of eCH4, and dairy performance, including milk FA composition.
We tested the effect of the nature of energy (lipids vs. carbohydrates) on eCH4 emission and performance in dairy cows fed four different iso-energetic grass silage-based diets supplemented with different levels of RO (0, 1.5, 3.0, 4.5% of dietary dry matter (DM), mainly in substitution of starch from concentrate. We aimed to find the best compromise between eCH4, dairy performance (notably milk and milk fat yields), and milk FA composition in dairy cows.
Materials and methods
The experimental procedures were approved by the Auvergne Rhône-Alpes Ethics Committee for Experiments on Animals C2EA-02 (France; DGRI agreement APAFIS#821-2015060811534198), and followed the European Union Directive 2010/63/EU guidelines (https://eur-lex.europa.eu/LexUriServ/LexUriServ.do?uri=OJ:L:2010:276:0033:0079:en:PDF). Experiments were performed at the INRAE experimental facilities (Herbipôle, INRAE, 63122 Saint-Genès-Champanelle, France, DOI: 10.15454/1.5572318050509348E12) from February to June 2016.
Animal, experimental design, and diets
Eight multiparous lactating Holstein cows after lactation peak (mean ± SD, BW of 726 ± 65 kg, DIM of 62 ± 2.5 days, parity of 2.5 ± 0.9, milk yield of 39.3 ± 2.5 kg/d, milk fat content of 36.9 ± 6.6 g/kg and milk protein content of 27.8 ± 1.6 g/kg at the start of the experiment) were blocked in pair and assigned to 1 of the 4 dietary treatments in a replicated 4 × 4 Latin square design with 4 experimental periods of 28 days.
Each experimental period consisted of an adaptation period to the diet from days 1 to 6. During the first 20 days, cows were housed together in a free-stall barn equipped with individual feed bunks and automatic gates (model Dairy Gates 3, Sodalec) and fed ad libitum. From days 21 to 26, cows were restricted fed (95% of individual voluntary feed intakes determined during days 7 to 20) to ensure total consumption of the diets and moved into individual open-circuit respiration chambers (RC) for measurement of dairy performance, gas emissions and total-tract digestibility. From days 27 to 28, the cows returned to the free-stall barn and were fed ad libitum the same diet as from days 7 to 21.
Cows were fed with four diets formulated to result in an iso-energetic intake, based on grass silage supplemented with different levels of RO (0, 1.5, 3.0, or 4.5% diet DM) in substitution of starch from concentrates. The main ingredients of the diets were forages (grass silage and hay), starchy pelleted concentrates (InVivo NSA, Chierry, France), and RO. The grass silage and hay (first cut) were harvested from a broadly diversified permanent grassland representative of the mountain area of central France. Rapeseed oil was manually mixed with pelleted concentrates immediately before feeding and top-dressed over the forages. The detailed composition of the diets offered to the animals during the measurement period is given in Table . As a decrease in intake was expected with increasing doses of RO in the diets, diet composition was formulated considering a linear decrease of 0.4 kg DM intake per 1% of diet DM-supplemented RO. Diets were formulated to meet individual requirements for the maintenance and lactation of dairy cows (INRA, Citation2018).
Table 1. Ingredients and chemical composition of the four experimental grass silage-based diets fed to dairy cows.
Cows were fed twice daily (08:00 h and 16:00 h) and received 2/3 of the daily offered diets in the morning, and the remaining 1/3 in the afternoon after milking. Cows had free access to water throughout the experiment. Animals were weighed at the beginning and end of each experimental period.
Measurements, sampling and chemical analyses
Feed intake and composition
Feed offers and refusals were weighed and recorded daily to estimate the DM intake (DMI). The DM content of the feed was determined (103 °C for 24 h) on samples (100 g) taken every day. In addition, 2 samples of each diet ingredient (100 g each) were taken on days 22 to 24 to be pooled. The first pooled sample was dried (60 °C for 48h) and the second one was freeze-dried; both samples were ground (1-mm screen, ZM 200 Retsch Mill) before being analysed for the determination of chemical composition.
Organic matter was determined by ashing at 550 °C for 6 h (method 942.05; AOAC International, Citation2005). Total nitrogen (N) was analysed according to the Dumas method (method 968.06; AOAC International, Citation2005) using a N-Dumas analyser (Dumas Method, Rapid N Cube, Elementar Analysensystem GmbH, Hanau, Germany); CP was calculated as N x 6.25. Fibre (NDF and ADF) content was determined by sequential procedures (Van Soest et al. Citation1991) using an Ankom system (Ankom Technology Corp., Fairport, NY, USA). Starch content was analysed by enzymatic method (Faisant et al. Citation1995) using a commercial kit for measuring the amount of starch broken down enzymatically (Boehringer Mannheim/R-Biopharm, Germany). The gross energy (GE) was analysed by isoperibolic calorimetry (model C200, IKA, Staufen, Germany). Ether extract content was determined after acid hydrolysis (method 954.02; AOAC International, Citation2005). The FA methyl esters of the freeze-dried feed samples were prepared using a direct transesterification procedure (Sukhija and Palmquist, Citation1988; adapted by Loor et al. (Citation2005) using 23:0 (Sigma, Saint-Quentin-Fallavier, France) as internal standard.
Milk yield and composition
Cows were milked twice daily (07:30 h and 15:30 h) and individual milk yield was recorded at each milking. Milk composition (fat, protein, lactose) was determined by infra-red spectroscopy using MilkoScan 4000 (Foss Electric A/S, Hillerod, Denmark; Lial, Aurillac, France) on milk samples (30 mL, 4 °C with 2-2-nitropropane-1,3-diol) collected over 6 successive milking on days 22 to 24. Individual fat and protein corrected milk (FPCM) yield was calculated according to Gerber et al. (Citation2011) as follows:
The milk FA composition was determined on individual milk samples (3 mL, −20 °C without preservative) taken after each milking on day 24. All samples were freeze-dried and then composited per day based on individual am and pm milk yields before analyses. The milk FA composition was determined by flame ion detection gas chromatography and the composition of FA methyl esters of CLA isomers in milk fat was analysed by HPLC as reported by Bougouin et al. (Citation2018).
Methane and carbon dioxide emissions
Daily eCH4 and CO2 emissions were measured continuously using 4 RC (1 cow per chamber) from days 21 (07:30 h) to 26 (07:30 h) of each experimental period. The system design and associated analytical equipment of the RC are detailed in Guyader et al. (Citation2015). Briefly, the RC (3.7 m long, 2.2 m wide and 2.4 m high, giving a volume of 19.6 m3) was operated at a slight negative pressure, with an average airflow of 687 m3/h (SD = 9.6). Airflow entered the RC through an aperture at the bottom of the rear door (0.44 m2) and exited through an exhaust duct on the top of the chamber (25-cm diameter). Airflow in the exhaust duct of each chamber was continuously measured (CP300 pressure transmitter; KIMO, MontponMénestérol, France) and recorded with 1 average data point every 5 min (KT210AO datalogger; KIMO). Concentrations of gases (CH4 and CO2) in the ambient air of the barn and in the 4 RC were alternatively analysed at a 0.1Hz sample frequency for 5 min every 25 min using an infra-red detector (Ultramat 6; Siemens, Karlsruhe, Germany) and recorded (Nanodac Invensys recorder/controller; Eurotherm Automation SAS, Dardilly, France). The detectors were manually calibrated the day before each measurement period using pure N2 and a standardised mixture of CH4 (650 mg/kg) and CO2 (700 mg/kg) in N2.
Management procedures of the RC before and during experiment are detailed in Bougouin et al. (Citation2018). Briefly, the RC was flushed with ambient air for 3 days before each measurement period. Doors to access the RC were opened only for feeding (front door), milking, and removing recovery boxes for faeces and urine collection (rear door); they were never opened at the same time. Gas emissions were calculated as the difference in gas concentration between RC and ambient air of the barn, multiplied by the airflow corrected for environmental parameters (temperature, relative humidity, and pressure) according to Pinares-Patiño et al. (Citation2012); they were not corrected by the CO2 recovery test of RC performed before starting the experiment.
Total-tract digestibility
Total faeces and urine were daily and individually collected during five consecutive days (days 22 to 26) of each experimental period when cows were in RC. After weighing and mixing of faeces and urine, two individual fresh daily aliquots (0.5% of daily excretion, each) of urine and faeces mixture were used for DM determination (103 °C for 24 h) and pooled weekly before drying (60 °C, 48 h) and grounding (1-mm screen, ZM 200 Retsch Mill) for chemical analyses (OM, NDF, ADF, starch), according to the procedures described for feeds.
Rumen parameters
Rumen fluid samples (500 mL) were collected after morning milking and before feeding on day 26 of each experimental by stomach tubing (Shen et al. Citation2012). Then, fluid samples were strained through a polyester monofilament fabric (250-µm pore size) and the filtrate was subsampled for VFA, ammonia (NH3) and protozoa analyses (Bougouin et al. Citation2018). The volatile fatty acids (VFA) and NH3 concentrations were analysed on a gas chromatograph equipped with a flame ionisation detector (Morgavi et al. Citation2008). Total protozoa counts were done by microscopy (Williams and Coleman Citation1992) and data were log10-transformed for statistical analysis.
Statistical analysis
All data collected daily during the period in the RC (intake, milk yield and composition, quantity of gas emitted and faeces and urine excreted) were weekly averaged for statistical analyses. The cow was used as a statistical unit. Data were analysed using mixed effect models with the lme4 package (version 1.1–23) in R statistical software (version 1.3.959, R Foundation for Statistical Computing, Vienna, Austria). Models included the fixed effects of period (n = 4), diet (n = 4), and the random effect of individual animals (n = 8). Least-squares means were reported with the pooled standard error of the mean derived from the model. Differences among means were adjusted for multiple comparisons using Tukey-Kramer’s method and were declared significant at p < 0.05. Results with p < 0.10 were considered as tendency. The normalised Z score was used for the spider-plots (Spiegel, Citation1975). The Z scores were calculated for each variable included in the spider plots as follow: Z = (x–μ)/σ, where x is the average of each diet; μ is the overall average and σ is the overall standard deviation.
As a results, the standardised Z scores have an average = 0 and a standard deviation = 1. As Z scores are standardised variables, their representation on a graph allows to compare variables expressed in different units of measurement, with different ranges. The extent of the difference for such variables among diets becomes comparable.
Results
Intake and dairy performance
In line with the experimental design, intakes of GE and CP were similar among diets. Intakes of starch and lipids, respectively, decreased and increased gradually from Control to RO-high (p < 0.001; Table ).
Table 2. Nutrient intakes and milk performances in dairy cows fed iso-energetic diets based on grass silage and characterised by different contents of rapeseed oil and starch.
Intakes of DM and OM were lower for RO-high compared to Control, with a decrease of −2.2 kg/d for DM and −3.0 kg/d for OM, (p < 0.05), respectively. Fibre intake did not differ between diets. The DM intake, lower than expected for RO-high, reduced the RO proportion of the diet from 4.5 to 4.0% when related to DM intake.
Milk yield was higher for RO-low and RO-medium compared to RO-high (+4.0 and +4.6 kg/day, respectively; p < 0.05), but none of the diets differed from Control (Table ). Feed efficiency was numerically higher (p < 0.10) for RO-low compared to Control and RO-high (+0.10 and +0.11 kg milk/kg DMI, respectively).
Milk fat content differed among diets (p < 0.001): it was the lowest in RO-medium (27.1 g/kg milk), followed by the RO-high (30.6 g/kg milk), but the milk fat content of RO-low (33.1 g/kg milk) did not differ from that of the Control (36.1 g/kg milk). The RO-high diet had the highest milk protein content, compared to the other diets (on average, +2.5 g/kg milk; p < 0.01). Consequently, FPCM was higher for RO-low than for RO-high (+3.9 kg/d), but none of these diets differed from Control. Milk fat yield was higher for Control and RO-low compared to RO-medium and RO-high (p < 0.001), while milk protein yield was not affected by the diet.
Methane and carbon dioxide emissions
Enteric CH4 emissions expressed in g/d, per unit of intake (g/kg DMI; % GEI), per unit of milk produced (g/kg milk) or per unit of nutrient digested (g/kg of digested DM, or g/kg of digested NDF) were lower for the RO-low, RO-medium and RO-high compared to the Control (p < 0.05; Table ).
Table 3. Methane and carbon dioxide emissions in dairy cows fed iso-energetic diets based on grass silage and characterised by different contents of rapeseed oil and starch.
The eCH4-mitigating effect was mainly observed with the RO-low compared to Control (i.e. −15%, −12%, and −21%, as expressed as g/d, g/kg DMI, g/kg milk, respectively; p < 0.05). The previously reported eCH4 production, yield and intensity were numerically lower with RO-medium and RO-high compared to RO-low.
Carbon dioxide emissions were lower for the RO-low, RO-medium and RO-high compared to Control (on average, −1298 g/d; p < 0.05; Table ). The CO2 emissions did not differ among diets including RO.
Total-tract digestibility and rumen fermentation
The total-tract digestibility of DM and OM was similar among the diets (Table ). Total-tract digestibility of fibre was higher for RO-high than Control (+4.9 and +5.3%, for NDF and ADF, respectively; p < 0.05).
Table 4. Total tract digestibility of nutrients and rumen parameters before feeding in dairy cows fed iso-energetic diets based on grass silage and characterised by different contents of rapeseed oil and starch.
The rumen concentrations of total VFA and NH3 were similar among diets, whereas VFA composition was modified by the diet (Table ). The rumen propionate proportion was higher in RO-high compared to all other diets (from 16.4 to 19.2%; p < 0.01), whereas the rumen butyrate proportion was lower (from 12.3 to 10.4%; p < 0.05), as well as the acetate/propionate and (acetate + butyrate)/propionate ratios (p < 0.01). The total protozoa concentration in the rumen was unaffected by the diet (p > 0.05).
Milk fatty acid composition
When dairy cows were fed with RO-low compared to Control, they produced milk with lower concentration of SFA, odd-chain FA (OCFA), de novo synthesised FA, and C16:0 (-9.32, −0.26, −4.37, −7.89 g/100 g FA, respectively; p < 0.001; Table ), whereas higher concentration of C18:0, C18:1c9, and MUFA were observed (+2.97, +5.58 and +6.17, g/100 g FA, respectively; p < 0.001; Table ). However, only the SFA, OCFA, and de novo synthesised FA, concentrations were lower, comparing RO-low to RO-medium or RO-high. Milk C16:0, C18:0, C18:1c9 and MUFA concentrations did not differ among diets. The BCFA, C18:2n-6, C18:3n-3, total n-3, and C18:1t10 concentrations did not differ between Control and RO-low, but BCFA, C18:2n-6, C18:3n-3, and total n-3 were lower, whereas the C18:1t10 was higher in RO-medium milk compared to RO-low milk (-0.23, −0.21, −0.15, −0.15, and +1.92 g/100 g FA, respectively; p < 0.05). The C18:2n-6 and C18:3n-3 concentrations were lower in RO-high compared to RO-medium milk. Milk from RO-low and RO-medium showed higher concentrations of C18:1t11, and CLAc9t11 (+1.46, and +0.47 g/100 g FA, respectively), compared to Control, but milk concentration from RO-high was lower compared to RO-low and RO-medium. Milk PUFA concentration differed from Control only for RO-low (+0.61 g/100 g FA), whereas tFA differed from Control only with RO-medium and RO-high (+5.72, and +3.97 g/100 g FA, respectively; p < 0.01). The full milk fatty acid profile in dairy cows fed the four different diets is given in Supplementary Table 1.
Table 5. Milk fatty acid profile in dairy cows fed iso-energetic diets based on grass silage and characterised by different contents of rapeseed oil and starch.
Compromise between enteric methane emissions, dairy performance, and milk fatty acid composition
Figure compares the four different grass silage-based and iso-energetic diets characterised by summarising the main pattern of each diet on digestive processes including eCH4 (g/d, g/kg DMI, g/kg milk), and milk production and composition. The difference between Control and diets containing RO is obvious for eCH4, the Control diet being the only diet far from the optimum eCH4 (Figure ). Only RO-low was close to Control in terms of milk fat content and the closest to the optimum concerning feed efficiency (Figure ). Concerning milk FA profile, Control showed the farthest SFA, C18:1c9 and CLAc9t11 concentrations from the optimum. With RO-low, all the FA become closer to the optimum (Figure ). RO-medium and RO-high diets showed the values the closest to the optimum for C18:1c9 and CLAc9t11 (only RO-medium), but n-6, n-3, BCFA and tFA got away from the optimum when compared to RO-low (Figure ).
Figure 1. Spider plots of the normalised (Z score) variables1 describing (a) dairy performance and digestion parameters including eCH4 and (b) milk fatty acid (FA) composition according to the nature of energy (rapeseed oil vs. starch) in the four iso-energetic and grass silage-based diets of dairy cows (Control, RO-low, RO-medium, RO-high). The optimum shape is represented by the red line: positive variables for the dairy performance or healthy milk FA for human nutrition were expected to have the maximum Z score, whereas the minimum Z score was desired for eCH4 or non-healthy milk FA (SFA); Z score scale from -1.5 to 1.0 with Z = 0 for the overall average of the four diets.
1Fatty acids, g/100g FA; Feed efficiency, kg milk/kg DMI; Milk yield, kg/d; Milk fat, g/kg; daily eCH4, g/d; eCH4 yield, g/kg DMI; eCH4 intensity, g/kg milk; NDFD, NDF digestibility, %; BCFA, branched-chain FA.

Discussion
Intake and dairy performance
The inclusion of RO in iso-energetic diets decreased nutrient intakes of dairy cows only for RO-high. This finding is in agreement with the literature data, in which a drop in DMI (about −2.5 kg DMI) was significant only for dairy cow diets supplemented with RO content greater than 3.3% of DM (Brask et al. Citation2013; Bayat et al. Citation2018), whereas no effect on DMI was reported when RO content was about 2.2% of DM (Hoffmann et al. Citation2016). Accordingly, to lower DMI in RO-high, the levels of RO and total lipids were about 4.0% and 7.7% of DMI, respectively. The resulting RO intake was above the recommendation for the total lipids content in cow diets (maximum 5 to 6% of DM; INRA, Citation2018).
Milk and FPCM yields were higher with RO-low and RO-medium than with Control diets, similar to the findings of Givens et al. (Citation2009) and Hoffmann et al. (Citation2016). However, this was not the case for RO-high, probably due to the reduced DMI. An MFD was observed for RO-medium and RO-high diets (differences between the control and the minimum values observed in the two diets: −9.0 g of fat/kg milk; −0.23 kg/d of fat yield, respectively). A decrease in milk fat content of about −2.5 g/kg milk was observed by Brask et al. (Citation2013) and Hoffmann et al. (Citation2016) with different rapeseed supplementation levels in cow diets (3.3% of DMI = 0.52 kg/d and 2.2% DMI = 0.51 kg/d of fat ingested from RO, respectively). These authors explained that this MFD is due to an inhibitory effect of the intermediates of t10 biohydrogenation pathways (notably C18:1t10 and CLAt10c12) on the de novo synthesis of FA in the mammary gland (Ferlay et al. Citation2017). A dilution due to lower milk yield could explain the higher milk protein content in RO-high diet, but this increase partially accounted for the compensation in FPCM, as FCM was similar to RO-medium, but still lower than RO-low.
Digestive processes including enteric methane emissions
Changing the nature of energy by including RO in dairy cow diets instead of starch reduced daily eCH4 compared to Control (–15%, −20% and −23% in g/d for RO-low, RO-medium and RO-high, respectively). This eCH4-mitigating effect was maintained when eCH4 was expressed per unit of intake or milk produced. In agreement with our results, Brask et al. (Citation2013) observed a decrease in eCH4 (–19% g/d, −11% g/kg DMI, −15% % GEI, −15% g/kg milk) with a RO supplementation of 3.3% DMI. A similar decrease in daily eCH4 (-23% g/d) was reported by Bayat et al. (Citation2018) with 5.0% RO supplementation, but this decrease was mostly ascribed to a decrease in DMI and milk yield, as eCH4 yield and intensity were unaffected by dietary treatment.
Several hypotheses have been put forward to explain the reduction in eCH4 with lipid supplements: lipids are not digested in the rumen and can lower the quantity of ruminal fermented OM, and consequently the quantity of H2 produced as a substrate for methanogenesis (Martin et al. Citation2010; Beauchemin et al. Citation2020); lipids can decrease the fibre digestibility, resulting in a higher ruminal fill that can decrease the DMI (Beauchemin and McGinn Citation2006; Brask et al. Citation2013). These hypotheses are not consistent with our results as the total VFA concentration, related to the quantity of OM fermented in the rumen, was similar among diets. In addition, total-tract fibre digestibility increased with RO-supplemented diets which might be related to the replacement of dehydrated alfalfa NDF from concentrates with more digestible NDF from grass and with the increase in forage/concentrate ratio with RO-supplemented diets (INRA Citation2018). Improvement of fibre digestibility when replacing carbohydrate energy by RO may be also related to lower ruminal digestive interactions between starch and fibre fractions and consequently, a lower depressive effect on total-tract fibre digestibility when starch content in cow diets is decreased (Martin and Michalet-Doreau Citation1996). In parallel to the decrease in eCH4, lipid supplementation can also reduce the protozoa population with a consequent orientation in ruminal fermentation to propionate at the expense of butyrate as reported by Guyader et al. (Citation2014) in their meta-analysis approach. This was observed in our trial only for RO-high. A stronger depressive effect on eCH4 (daily, yield and intensity) was observed with RO-low when compared to the Control diet but without changes in protozoa concentration and VFA proportion in the rumen. This suggests that the action of RO in reducing eCH4 emissions was related to a direct inhibition of methanogens by dietary unsaturated FA (Martin et al. Citation2010; Popova et al. Citation2011). Another explanation of eCH4 (daily, yield and intensity) reduction could be the H2 uptake by the ruminal biohydrogenation of rapeseed unsaturated FA, but its influence on methanogenesis is negligible (i.e. 1–2%) based on stoichiometric (Czerkawski, Citation1986) and modelling (Mills et al. Citation2001; Giger-Reverdin et al. Citation2003) approaches.
Milk fatty acid composition
Changing the nature of energy (lipids vs. carbohydrates) in iso-energetic diets by including RO affected most of the milk FA. Lower concentration of milk SFA, de novo synthesised FA, and C16:0 in RO diets compared to Control is in agreement with Hoffmann et al. (Citation2016), which found similar results with crushed rapeseed or rapeseed oil fed to dairy cows at 2.2% DMI. These changes are consistent with the fact that dietary inclusion of FA with 18 carbons decreases milk short- and medium-chain SFA through the inhibition of de novo synthesis in the mammary gland (Chilliard et al. Citation2007; Shingfield et al. Citation2010). In parallel, the higher milk concentrations of C18:1c9, C18:1t11, and other intermediate products of dietary PUFA ruminal biohydrogenation in the diets containing RO compared to Control, is in agreement with the higher intake of unsaturated FA with diets including rapeseed (Shingfield et al. Citation2010; Ferlay et al. Citation2017). Indeed, when the intake of unsaturated FA from rapeseed is increased, the milk concentration of most unsaturated long-chain FA, notably those of biohydrogenation intermediate products, increases whatever the form of rapeseed supplementation in dairy cow diets (Givens et al. Citation2009; Bougouin et al. Citation2019). This was observed in our trial with RO-low and RO-medium diets. The reduction of the concentration of FA from de novo synthesis origin is in agreement with the MFD existing for these diets and confirmed by the decreased milk fat content and the shift from C18:1t11 to C18:1t10 observed in milk with RO-medium and RO-high diets (Bauman and Griinari Citation2003). The FA with a trans double bound on carbon 10 has an antilipogenic effect, that decreases de novo FA synthesis in the mammary gland (Bauman and Griinari Citation2003).
High levels of RO inclusion in iso-energetic diets decreased milk concentrations of C18:2n-6 and C18:3n-3, despite a higher intake of these FA and a higher forage to concentrate ratio. This was in agreement with Ferlay et al. (Citation2013), who tested the effect of increasing doses of linseed (rich in C18:3n-3 and C18:2n-6) on milk FA composition. These authors showed a negative exponential relationship between C18:2n-6 or C18:3n-3 intake and their transfer efficiency from the feed to milk, suggesting that the ruminal biohydrogenation rate increased with the availability of the substrate (Ferlay et al. Citation2013). However, a saturation effect by increased dietary 18-carbon FA towards the mammary gland may have limited the uptake of plasma FA (Ferlay et al. Citation2017). Both of these hypotheses can also be supported by the lack of differences in milk concentration of C18:1c9 (and consequently of MUFA) for RO-medium and RO-high compared to RO-low diets.
Compromise between enteric methane emissions, dairy performance, and milk fatty acid composition
Figure clearly shows the advantages of changing the nature of energy (lipids vs. carbohydrates) by introducing different levels of RO in substitution of starch in dairy cow diets in terms of decreasing eCH4 increasing milk yield and feed efficiency, and improving milk FA profile (higher concentrations of MUFA and C18:1c9, and lower concentration of SFA) beneficial for human health.
With the highest levels of RO inclusion, we observed negative effects on dairy performance (decreased milk yield and feed efficiency with RO-high), including MFD and changes in milk FA profile unfavourable for human health (decreased n-3 and n-6 FA concentration, and increased trans FA concentration, in particular that of C18:1t10) with both RO-medium and RO-high. Consequently, in our experimental conditions, the change of the nature of energy (lipids vs. carbohydrates) by including low RO (1.5% DM) in iso-energetic grass silage-based diets resulted in the best compromise for matching a significant reduction in eCH4 (–15% in g/d, −12% in g/kg DMI, −21% in g/kg milk) in dairy cows, increasing dairy performance, and significantly improving milk FA composition for human health.
Conclusion
This study highlighted that including different levels of RO (1.5 to 4.5% of DM) in substitution of starch in grass silage-based diets reduced eCH4 from dairy cows (-19% g/d, −13% g/kg DMI, −21% g/kg milk, on average). Furthermore, RO inclusion increased milk concentration of unsaturated FA (including oleic acid), and reduced the concentration of SFA. However, MFD occurred for the higher levels (> 3% of dietary DM) of RO inclusion in the diets. Thus, a low level of RO (1.5% of dietary DM) in iso-energetic grass-silage based diets resulted in the best compromise to decrease eCH4 and maintain or improve dairy performance and milk nutritional quality for human health.
Acknowledgments
This study was part of the Joint Programming Initiative on Agriculture, Food Security and Climate Change (FACCE-JPI)’s “GLOBAL NETWORK” project. The authors gratefully acknowledge the staff of the Unité Expérimentale Herbipôle for his diligent care of the experimental animals, and of the UMR 1213 Herbivores for his assistance with sample collection and laboratory analysis.
Disclosure statement
No potential conflict of interest was reported by the author(s).
Data availability statement
The data that support the findings of this study are available from the corresponding author, CM, upon reasonable request.
Additional information
Funding
References
- Association of Official Agricultural Chemists International [AOAC]. 2005. Official Methods of Analysis. 18th ed. AOAC Int., Arlington, VA.
- Arndt C, Hristov AN, Price WJ, McClelland SC, Pelaez AM, Cueva SF, Oh J, Dijkstra J, Bannink A, Bayat AR, et al. 2022. Full adoption of the most effective strategies to mitigate methane emissions by ruminants can help meet the 1.5°Ctarget by 2030 but not 2050. Proc Natl Acad Sci U S A. 119(20):e2111294119. doi: 10.1073/pnas.2111294119.
- Bauman DE, Griinari JM. 2003. Nutritional regulation of milk fat synthesis. Annu Rev Nutr. 23(1):203–227. doi: 10.1146/annurev.nutr.23.011702.073408.
- Bayat AR, Tapio I, Vilkki J, Shingfield KJ, Leskinen H. 2018. Plant oil supplements reduce methane emissions and improve milk fatty acid composition in dairy cows fed grass silage-based diets without affecting milk yield. J Dairy Sci. 101(2):1136–1151. doi: 10.3168/jds.2017-13545.
- Beauchemin KA, McGinn SM. 2006. Methane emissions from beef cattle: effects of fumaric acid, essential oil, and canola oil. J Anim Sci. 84(6):1489–1496. doi: 10.2527/2006.8461489x.
- Beauchemin KA, Ungerfeld EM, Eckard RJ, Wang M. 2020. Review: fifty years of research on rumen methanogenesis: lessons learned and future challenges for mitigation. Animal. 14(S1):s2–s16. doi: 10.1017/S1751731119003100.
- Bernard L, Pomiès D, Aronen I, Ferlay A. 2021. Effect of concentrate enriched with palmitic acid versus rapeseed oil on dairy performance, milk fatty acid composition, and mammary lipogenic gene expression in mid-lactation Holstein cows. J Dairy Sci. 104(11):11621–11633. doi: 10.3168/jds.2020-20023.
- Bougouin A, Ferlay A, Doreau M, Martin C. 2018. Effects of carbohydrate type or bicarbonate addition to grass silage-based diets on enteric methane emissions, milk production, and fatty acid composition in dairy cows. J Dairy Sci. 101(7):6085–6097. doi: 10.3168/jds.2017-14041.
- Bougouin A, Martin C, Doreau M, Ferlay A. 2019. Effects of starch-rich or lipid-supplemented diets that induce milk fat depression on rumen biohydrogenation of fatty acids and methanogenesis in lactating dairy cows. Animal. 13(7):1421–1431. doi: 10.1017/S1751731118003154.
- Brask M, Lund P, Weisbjerg MR, Hellwing ALF, Poulsen M, Larsen MK, Hvelplund T. 2013. Methane production and digestion of different physical forms of rapeseed as fat supplements in dairy cows. J Dairy Sci. 96(4):2356–2365. doi: 10.3168/jds.2011-5239.
- Chilliard Y, Glasser F, Ferlay A, Bernard L, Rouel J, Doreau M. 2007. Diet, rumen biohydrogenation and nutritional quality of cow and goat milk fat. Eur J Lipid Sci Technol. 109(8):828–855. doi: 10.1002/ejlt.200700080.
- Czerkawski JW. 1986. An Introduction to Rumen Studies, Pergamon Press, Oxfordshire and New York.
- Food and Agriculture Organization of the United Nations [FAO]. 2020. World Food and Agriculture - Statistical Yearbook 2020. Rome.
- Faisant N, Plachot V, Kozlowski F, Pacouret MP, Colonna P, Champ M. 1995. Resistant starch determination adapted to products containing high level of resistant starch. Sci Alim. 15:83–89.
- Ferlay A, Bernard L, Meynadier A, Malpuech-Brugère C. 2017. Production of trans and conjugated fatty acids in dairy ruminants and their putative effects on human health: a review. Biochimie. 141:107–120. doi: 10.1016/j.biochi.2017.08.006.
- Ferlay A, Doreau M, Martin C, Chilliard Y. 2013. Effects of incremental amounts of extruded linseed on milk fatty acid composition in dairy cows receiving hay or corn silage. J Dairy Sci. 96(10):6577–6595. doi: 10.3168/jds.2013-6562.
- Fougère H, Delavaud C, Bernard L. 2018. Diets supplemented with starch and corn oil, marine algae, or hydrogenated palm oil differentially modulate milk fat secretion and composition in cows and goats: a comparative study. J Dairy Sci. 101(9):8429–8445. doi: 10.3168/jds.2018-14483.
- Gerber P, Vellinga T, Opio C, Steinfeld H. 2011. Productivity gains and greenhouse gas emissions intensity in dairy systems. Livestock Sci. 139: 100–108. doi:10.1016/j.livsci.2011.03.012.
- Giger-Reverdin S, Morand-Fehr P, Tran G. 2003. Literature survey of the influence of dietary fat composition on methane production in dairy cattle. Livest Prod Sci. 82(1):73–79. doi: 10.1016/S0301-6226(03)00002-2.
- Givens DI, Kliem KE, Humphries DJ, Shingfield KJ, Morgan R. 2009. Effect of replacing calcium salts of palm oil distillate with rapeseed oil, milled or whole rapeseeds on milk fatty-acid composition in cows fed maize silage-based diets. Animal. 3(12):1067–1074.
- Guyader J, Eugène M, Nozière P, Morgavi D, Doreau M, Martin C. 2014. Influence of rumen protozoa on methane emission in ruminants: a meta-analysis approach. Animal. 8(11):1816–1825. doi: 10.1017/S1751731114001852.
- Guyader J, Eugène M, Meunier B, Doreau M, Morgavi DP, Silberberg M, Rochette Y, Gérard C, Loncke C, Martin C. 2015. Additive methane-mitigating effect between linseed oil and nitrate fed to cattle. J Anim Sci. 93(7):3564–3577. doi: 10.2527/jas.2014-8196.
- Hoffmann A, Görlich S, Steingass H, Terry H, Schollenberger M, Hartung K, Mosenthin R. 2016. Milk production and milk fatty acids in dairy cows fed crushed rapeseed or rapeseed oil. Livestock Sci. 190:31–34. doi: 10.1016/j.livsci.2016.05.016.
- Hristov AN, Oh J, Lee C, Meinen R, Montes F, Ott T, Oosting S. 2013. Mitigation of greenhouse gas emissions in livestock production – a review of technical options for non-CO2 emissions. FAO Animal Production and Health Paper No. 177. Rome, Italy: Food and Agriculture Organization of the United Nations.
- Institut National de la Recherche Agronomique [INRA]. 2018. Alimentation des ruminants. Versailles, Paris: Quae.
- Kliem KE, Humphries DJ, Kirton P, Givens DI, Reynolds CK. 2019. Differential effects of oilseed supplements on methane production and milk fatty acid concentrations in dairy cows. Animal. 13(2):309–317. doi: 10.1017/S1751731118001398.
- Kliem KE, Humphries DJ, Reynolds CK, Morgan R, Givens DI. 2017. Effect of replacing calcium salts of palm oil distillate with rapeseed oil, milled or whole rapeseeds on milk fatty-acid composition in cows fed maize silage-based diets. Animal. 11(2):354–364. doi: 10.1017/S175173110900442X.
- Kliem KE, Shingfield KJ. 2016. Manipulation of milk fatty acid composition in lactating cows: opportunities and challenges. Eur J Lipid Sci Technol. 118(11):1661–1683. doi: 10.1002/ejlt.201400543.
- Lerch S, Pires JAA, Delavaud C, Shingfield KJ, Pomiès D, Martin B, Chilliard Y, Ferlay A. 2015. Rapeseed or linseed supplements in grass-based diets: effects on milk fatty acid composition of Holstein cows over two consecutive lactations. J Dairy Sci. 98(2):1005–1018. doi: 10.3168/jds.2012-5337.
- Loor JJ, Doreau M, Chardigny JM, Ollier A, Sebedio JL, Chilliard Y. 2005. Effects of ruminal or duodenal supply of fish oil on milk fat secretion and profiles of trans-fatty acids and conjugated linoleic acid isomers in dairy cows fed maize silage. Anim Feed Sci Technol. 119(3-4):227–246. doi: 10.1016/j.anifeedsci.2004.12.016.
- Martin C, Michalet-Doreau B. 1996. Influence of barley and buffer supplements on quantitative aspects of ruminal fiber digestion in cows. Arch Tierernahr. 49(3):203–211. doi: 10.1080/17450399609381881.
- Martin C, Morgavi DP, Doreau M. 2010. Methane mitigation in ruminants: from microbe to the farm scale. Animal. 4(3):351–365. doi: 10.1017/S1751731109990620.
- Mills JA, Dijkstra J, Bannink A, Cammell SB, Kebreab E, France J. 2001. A mechanistic model of whole-tract digestion and methanogenesis in the lactating dairy cow: model development, evaluation, and application. J Anim Sci. 79(6):1584–1597. doi: 10.2527/2001.7961584x.
- Morgavi DP, Jouany JP, Martin C. 2008. Changes in methane emission and rumen fermentation parameters induced by refaunation in sheep. Aust J Exp Agric. 48(2):69–72. doi: 10.1071/EA07236.
- Muñoz C, Sánchez R, Peralta AMT, Espíndola S, Yan T, Morales R, Ungerfeld EM. 2019. Effects of feeding unprocessed oilseeds on methane emission, nitrogen utilization efficiency and milk fatty acid profile of lactating dairy cows. Anim Feed Sci Technol. 249:18–30. doi: 10.1016/j.anifeedsci.2019.01.015.
- Pinares-Patiño CS, Hunt C, Martin R, West J, Lovejoy P, Waghorn G. 2012. New Zealand ruminant methane measurement centre, AgResearch, Palmerston North. In Technical Manual on Respiration Chamber Designs. C. S. Pinares Patino and G. Waghorn, eds. Wellington, New Zealand: Ministry of Agriculture and Forestry p. 9–28.
- Popova M, Morgavi DP, Doreau M, Martin C. 2011. Methane production and ruminal microbial interactions. INRA Prod Anim. 24(5):447–460. doi: 10.20870/productions-animales.2011.24.5.3277.
- Robson DS. 1959. A simple method for constructing orthogonal polynomials when the independent variable is unequally spaced. Biometrics. 15(2):187–191. doi: 10.2307/2527668.
- Shen JS, Chai Z, Song LJ, Liu JX, Wu YM. 2012. Insertion depth of oral stomach tubes may affect the fermentation parameters of ruminal fluid collected in dairy cows. J Dairy Sci. 95(10):5978–5984. doi: 10.3168/jds.2012-5499.
- Shingfield KJ, Bernard L, Leroux C, Chilliard Y. 2010. Role of trans fatty acids in the nutritional regulation of mammary lipogenesis in ruminants. Animal. 4(7):1140–1166. doi: 10.1017/S1751731110000510.
- Spiegel MR. 1975. Probability and Statistics. New York, US: McGraw-Hill Inc. .
- Sukhija P, Palmquist D. 1988. Rapid method for determination of total fatty-acid content and composition of feedstuffs and feces. J Agric Food Chem. 36(6):1202–1206. doi: 10.1021/jf00084a019.
- Vahmani P, Meadus WJ, Duff P, Rolland DC, Dugan MER. 2017. Comparing the lipogenic and cholesterolgenic effects of individual trans-18:1 isomers in liver cells. Eur J Lipid Sci. Technol. 119:410–418.
- Van Soest PJ, Robertson JB, Lewis BA. 1991. Methods for dietary fiber, neutral detergent fiber, and nonstarch polysaccharides in relation to animal nutrition. J Dairy Sci. 74(10):3583–3597. doi: 10.3168/jds.S0022-0302(91)78551-2.
- Williams AG, Coleman GS. 1992. The rumen protozoa. London, UK: Springer-Verlag.