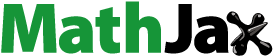
ABSTRACT
This study investigated RCA as a sustainable, cost-effective NA substitute in road construction. Mixtures with 0–100% RCA were tested under freeze-thaw (F-T) cycles for resilience and deformation, compared to non-exposed controls. The F-T cycles, mix composition, and stiffness are interrelated. The resilient modulus of NA-RCA mixtures increased for up to 5 F-T cycles, then slightly dropped. The 50% NA + 50% RCA mixture showed improved stiffness, with average MR increasing from about 321 MPa to 420 MPa. The 100% NA sample’s average MR dropped from about 220 MPa to 196 MPa after 5 F-T cycles. As RCA content increased to 50%, plastic deformation decreased from about 0.085% and then slightly increased. The 50% NA and 50% RCA blend showed the least plastic deformation (0.065%) in all F-T cycles, demonstrating RCA’s effective use in road construction. This composition showed great stiffness and resilience even after multiple freeze-thaw cycles. These results support sustainable road construction without compromising performance. It is essential to note that the study’s scope was limited to laboratory conditions, which may not fully represent real-world conditions. Future research should field test RCA and NA mixtures in various environments to validate the findings and assess long-term performance.
1. Introduction
The high potential for recycling construction waste is increasing its efficient use in various applications within the construction industry; thus, construction waste is gaining increasing importance both economically and environmentally (Bamigboye et al. Citation2021). Recycled concrete aggregates (RCA), derived from construction waste, serve as a sustainable alternative to traditional road building materials in road infrastructure, which is known to consume a significant amount of natural aggregates. In particular, they offer a sustainable solution for managing construction waste generated from large construction projects such as urban renewal projects (Jitsangiam et al. Citation2015; Zaharieva, Buyle-Bodin, and Wirquin Citation2004).
The performance of the base layers, which constitute part of the road infrastructure, is largely determined by the resilience modulus and plastic deformation values, which dictate the load-bearing capacity of the road as well as its resistance to deformation and crack formation (Gokce et al. Citation2011; Gong et al. Citation2015; Li et al. Citation2021). Resilient modulus is a measure of the road material’s ability to return to its original shape after being deformed under a load, and is critical for determining how well the road can absorb and dissipate energy from traffic loads. A higher resilient modulus indicates that the material is better able to resist deformation under load, leading to a smoother, more stable road surface. Plastic deformation, on the other hand, is a permanent change in the shape of the road material due to loading. Excessive plastic deformation can lead to ruts, potholes, and other types of road surface damage, which can decrease the lifespan of the road and increase maintenance costs (Rahla et al.,2021). Therefore, optimising the resilient modulus and minimising plastic deformation are key factors in designing road base layers that are durable and can withstand the constant traffic loads. Freeze-thaw (F-T) cycles, which significantly affect the durability of the layers constituting the road infrastructure in cold climate conditions, can degrade the overall road performance by altering the structure of the material used in the road base layer, thus negatively affecting its resilience modulus and plastic deformation value (Gokce et al. Citation2011; Zhi et al. Citation2023).
Numerous studies have examined the physical, mechanical, and strength properties of road base layers obtained using natural aggregates (NA) and/or RCA (Meesala Citation2019; Wang et al. Citation2022; Wu and Marty Citation2023). For example, Jayakody Arachchige et al. (Citation2012) conducted tests by mixing six different proportions of RCA and compared the results obtained from classification tests, such as particle size distribution, plasticity, compaction, and California Bearing Ratio (CBR), with the results of standard road materials. It was determined that only the base materials mixed in specific ratios were within the limits of the minimum requirements, whereas the others were below these limits. Saberi et al. (Citation2021) implemented a range of conventional tests to assess the mechanical and physical characteristics of RCA, and the outcomes of these examinations revealed that the aggregates presented a superior distribution of particle sizes, durability, and fitting shapes that aligned with the requirements. Additionally, the RCA samples exhibited acceptable CBR values and adequate strength, validating their suitability for road base construction. Aghililotf et al. (Citation2021) assessed the potential of using RCA in road construction layers without confinement. Six NA and three RCA types were examined through various physical, mechanical, and durability tests. The results suggest that all the RCA are suitable for standard road construction applications.
Despite the increasing number of studies that examine the resilience properties of road base layers made with RCA and NA (Meesala Citation2019; Wang et al. Citation2022; Wu and Marty Citation2023), most of them have concentrated on either the effect of the RCA proportion or the effect of F-T cycles on the stiffness characteristics. Research into the performance of the road base layer with different proportions of RCA and varying numbers of F-T cycles is still quite limited. For instance, Toka and Olgun (Citation2022) used NA and RCA from concrete with three different compressive strengths and created mixtures by adding RCAs to NA in proportions of 25%, 50%, and 75%. Notably, these mixtures were not exposed to F-T cycles. The resilient modulus and plastic deformation of these composite materials were evaluated, with 75% RCA mixtures showing the highest resilient modulus values. In a comparable study conducted by Bestgen et al. Twenty different RCA types and four NA materials, as well as chosen mixtures, were evaluated for their resilient moduli and plastic deformations (Bestgen et al. Citation2016). In this study, the resilient modulus values of the 100% RCA sample were assessed by subjecting it to various F-T cycles. Nevertheless, all combinations were tested for plastic deformation after changing the number of F-T cycles. They determined that the samples of 100% RCA and 100% NA had higher resilient modulus values than the mixtures of RCA-NA. Additionally, RCA exhibited better stiffness properties than NA, which further improved when exposed to F-T cycles. In a separate study by Edil et al. (Citation2018), test sections of road base layers were constructed using 100% Reclaimed Asphalt Pavement (RAP), 100% Recycled Concrete Aggregate (RCA), a 50/50 blend of RCA and Natural Aggregate (NA), and a control section of 100% NA. The number of freeze-thaw cycles each test section encountered in the field was determined by examining the base-course layer temperatures. The resilient modulus of the 100% RCA, 100% RAP, and 100% NA samples were tested in the laboratory after up to 20 F-T cycles, but not on the 50/50 RCA-NA blend. The laboratory results revealed that the resilient modulus for RCA increased after 5 freeze-thaw cycles, while the resilient modulus for NA decreased by around 60% after 20 freeze-thaw cycles. Additionally, Haider et al. (Citation2014) conducted laboratory tests on two different RCAs blended with NA in varying proportions to assess the suitability of RCA for road base layers. Their evaluation of the resilient modulus and plastic deformation characteristics under freeze-thaw cycles revealed that RCA has a higher resilient modulus value than NA, with the value increasing under freeze-thaw conditions. Furthermore, mixtures composed of different ratios of RCA and NA generally showed a higher resilient modulus than the individual RCA or NA samples.
In light of the existing literature (Bamigboye et al. Citation2021; Rahla et al.,2021), this study aims to address the gap in understanding the combined effects of different RCA proportions and F-T cycles on the resilient modulus and plastic deformation properties of road base layers. Specifically, this research seeks to assess the impact of various proportions of RCA (0%, 25%, 50%, 75%, and 100%) in combination with NA on the resilient modulus and plastic deformation properties of road base layers. Furthermore, it aims to investigate the effect of F-T cycles (1–20 cycles) on the resilient modulus and plastic deformation of the NA-RCA mixtures. The study also aims to identify the optimal RCA content that enhances the stiffness and resistance to deformation of road base layers, even after multiple F-T cycles. The incorporation of RCA into road base layers offers numerous potential benefits for the construction industry and sustainable road infrastructure, including reducing the consumption of natural aggregates, decreasing landfill waste, and promoting sustainable construction practices. The practical implications of this research include providing recommendations for specific proportions of RCA for road base layers that offer improved resilience and resistance to plastic deformation under varying F-T cycles. These recommendations have direct applications in road construction projects, especially in regions prone to temperature fluctuations, and could lead to reduced road maintenance costs, lower demand for natural aggregates, and minimised environmental impact. By achieving these results, this study provides a comprehensive understanding of the interactions between mechanical loading and environmental factors in the performance of base-course materials with varying ratios of NA and RCA. Ultimately, the results of this study are expected to provide valuable insights into the sustainable design and construction of road infrastructure in regions prone to temperature fluctuations, contributing to the advancement of the construction industry and the development of more sustainable road infrastructure. For these purposes, this research was conducted to gain a thorough understanding of the stiffness characteristics of base mixtures that contain various proportions of RCA/NA that have been subjected to different numbers of F-T cycles. To this end, samples were created with varying proportions of RCA obtained from an urban redevelopment project in Istanbul and NA taken from a mine in Istanbul. The base samples were formulated as follows: 100% NA, 75% NA and 25% RCA, 50% NA and 50% RCA, 25% NA and 75% RCA, and 100% RCA. The physical, mechanical, and hydrological characteristics of the samples were evaluated, and they were subjected to 1, 5, 10, and 20 freeze-thaw cycles. After these cycles, the resilient modulus and plastic deformation values were calculated through resilient and plastic deformation tests, and the results were compared to those of samples that had not been exposed to freeze-thaw cycles. By achieving these results, this study provides a comprehensive understanding of the interactions between mechanical loading and environmental factors in the performance of base-course materials with varying ratios of NA and RCA. Ultimately, the results of this study are expected to provide valuable insights into the sustainable design and construction of road infrastructure in regions prone to temperature fluctuations.
2. Materials
The urban redevelopment project in Istanbul generated construction and demolition (C&D) waste which was processed in recycling centres. Within the scope of the experimental research, recycled concrete aggregates (RCA) were obtained from this waste, which had a maximum grain size of 30 mm after being crushed and sorted by type. Natural aggregates (NA) were sourced from a quarry in the Istanbul area of Turkey. The road performance was evaluated under different numbers of freeze-thaw (F-T) cycles by combining RCA and NA in various proportions of 0%, 25%, 50%, 75%, and 100% by weight.
The aggregates that were received were sifted in the lab to obtain different grain sizes. The base samples were created by combining aggregates of different grain sizes. These samples, which had the specified grain-size distributions, fulfilled the criteria outlined in the AASHTO Guide for Design of Pavement Structures (1993). illustrates the grain size distribution of all materials used in this study. The physical properties of the mixtures, as outlined in , were evaluated using various tests, such as sodium or magnesium sulphate use (ASTM C88), Los Angeles (LA) abrasion coefficient (ASTM C131), flakiness index (FI) (BS EN 933–3), 24-hour water absorption (WA24) (ASTM C127), percentage of clay lumps and friable particles in aggregates (ASTM C142), methylene blue index of clay (ASTM C837), and specific gravity (ASTM D854). outlines the mechanical and hydraulic characteristics of these mixtures. These characteristics were obtained from a series of tests conducted in accordance with the ASTM standards: modified Proctor (ASTM D1557), CBR (ASTM D1883), and constant head (ASTM D2434) tests.
Figure 1. Distribution of grain size distributions for all mixtures.

Table 1. Physical properties of the mixtures.
Table 2. Mechanical and hydraulic properties of mixtures.
3. Experimental design and procedures
The effectiveness of road is greatly influenced by how the base-course material reacts to repeated wheel loads. To evaluate the stiffness characteristics of the base-course mixtures, a series of resilient modulus and plastic deformation tests were carried out. To begin with, these tests were conducted on control mixtures that were unfrozen and comprised of different compositions such as 100% Natural Aggregate (NA), 75% NA and 25% Recycled Concrete Aggregates (RCA), 50% NA and 50% RCA, 25% NA and 75% RCA, and 100% RCA. For example, the mixture labelled 25% NA + 75% RCA is a sample composed of 25% NA and 75% RCA, each by dry weight. Afterwards, these mixtures were exposed to freeze-thaw (F-T) cycles to replicate environmental conditions. After 1, 5, 10, and 20 freeze-thaw cycles, the mixtures were tested again for resilient modulus and plastic deformation. This process enabled the evaluation of the stiffness characteristics of the mixtures under the impact of F-T cycles and facilitated comparisons with the control samples.
3.1. Resilient modulus tests
Based on sieve analyses and Atterberg limit test results, mixtures with less than 70% and 20% of the particles passing through No. 10 (2 mm) and No. Two hundred (0.074 mm) sieves and a plasticity index below 10% were defined as Type 1, according to AASHTO T-307. The resilient modulus test was conducted by following the necessary steps such as connecting the drainage line and aligning the loading device with the triaxial chamber. A predetermined confining pressure of 41.4 kPa was applied to the samples enclosed by a rubber membrane ().
Figure 2. Preparation of mixtures for resilient modulus test.

The resilient modulus test was initiated by applying a haversine-shaped load for 500 repetitions, each with a load duration of 0.1 seconds and a rest period of 0.9 seconds. This conditioning sequence was followed by another 100 repetitions across the 15 sequences. The resilient modulus quantifies the response of a material to a brief dynamic pulsed load. It is defined as the ratio of deviatoric stress applied to the recovered (resilient) strain (σd/εr) (Bilodeau, Doré, and Schwarz Citation2011).
3.2. Plastic deformation tests
Plastic deformation tests specifically provide insight into how these materials respond under various conditions, contributing to an understanding of their durability and reliability when used in road base courses.
The samples used in these plastic deformation tests were those that had undergone the resilient modulus tests. The decision to use these mixtures was based on the observation that, during the resilient modulus test, the plastic vertical strain did not exceed 3%. This suggests that these specimens retained significant structural integrity, making them suitable candidates for further testing in plastic deformation tests (Bilodeau, Doré, and Schwarz Citation2011). Following the completion of the resilient modulus tests, the specimens were maintained in a triaxial chamber without removal. The same preconditioning load sequence as that used in the resilient modulus test was used. The specimens were then subjected to a consistent confining pressure (σ3) of 38.7 kPa and deviator stress (σd) of 108 kPa in accordance with previous studies (Bilodeau, Doré, and Schwarz Citation2011). This loading regime was applied for a total of 10,000 load repetitions.
3.3. F-T cycles
The ASTM D6035 was employed to study the long-term effects of freeze-thaw (F-T) cycles on the stiffness characteristics of the mixtures. The freezing and thawing temperatures for the F-T cycles were set at − 20°C and 20°C, respectively. The freeze-thaw cycle involved freezing the samples first and then allowing them to thaw completely. The samples were exposed to 1, 5, 10, and 20 freeze-thaw cycles. At the conclusion of each F-T cycle, resilient modulus tests and plastic deformation tests were performed on the mixtures ().
4. Experimental results and discussion
The base course material was evaluated experimentally by conducting a series of resilient modulus and plastic deformation tests in various freeze-thaw (F-T) conditions. The five distinct compositions that comprised different combinations of Natural Aggregate (NA) and Recycled Concrete Aggregates (RCA) were 100% NA, 75% NA. 25% RCA, 50% NA combined with 50% RCA, 25% NA in conjunction with 75% RCA, and 100% RCA. Initially, 10 NA + RCA control mixtures that had not gone through F-T cycles were created. Of these, five were allocated for the resilient modulus tests and the other five for the plastic deformation tests. Afterwards, the impact of the F-T cycles was evaluated by subjecting further mixtures to 1, 5, 10, and 20 F-T cycles and then measuring the resilient modulus and plastic deformation. To this end, 50 more mixtures of NA + RCA were created, with 25 of them used for resilient modulus tests and the other 25 for plastic deformation tests.
4.1. Control mixtures
The control mixtures were formulated with the following compositions: 100% NA, 75% NA and 25% RCA, 50% NA and 50% RCA, 25% NA and 75% RCA, and 100% RCA. The resilient modulus and plastic deformation tests were conducted on these mixtures to compare the outcomes after each specified F-T cycle.
4.1.1. Resilient modulus test results
The resilient modulus and plastic deformation tests were conducted on each control mixture. The tests were conducted in a non-frozen state to provide a baseline for assessing the effectiveness of the mixtures. The resilient modulus tests showed that the stiffness of the mixtures varied depending on the proportion of NA and RCA. displays the resilient modulus test results of the mixtures for the 1st (isotropic confining pressure of approximately 21 kPa), 7th (isotropic confining pressure of approximately 69 kPa), and 12th (isotropic confining pressure of approximately 103 kPa) loading series.
Figure 4. Results of resilient modulus tests for mixtures across the loading series.

The behaviour of each mixture during the loading series yielded further insights. In the initial loading series (isotropic confining pressure of approximately 21 kPa), all mixtures showed their lowest MR values, as expected, since the samples had not yet been subjected to extensive loading. Notably, the 50% NA + 50% RCA and 25% NA + 75% RCA mixtures showed significantly higher MR values than the other mixtures, indicating a greater initial stiffness when subjected to these loading conditions. The MR values usually rose in the 7th (isotropic confining pressure of around 69 kPa) and 12th (isotropic confining pressure of approximately 103 kPa) loading series. This could be attributed to the compaction or densification effects that take place when the material is subjected to repeated loading, thus increasing its stiffness. The 50% NA + 50% RCA and 25% NA + 75% RCA mixtures showed relatively high MR values, confirming their superior stiffness properties.
The 100% NA mixture had the lowest mean resilient modulus (MR) of 220 MPa, as expected. The 75% NA and 25% RCA mix had a slightly higher MR of 235.5 MPa. Surprisingly, the 50/50 NA/RCA and 25% NA/75% RCA mixtures had the highest MR values of 321 and 290 MPa, respectively. The 100% RCA mixture had a lower average MR of 237 MPa, suggesting that a completely recycled mixture may not be the most suitable in terms of stiffness.
RCA increased the road base course’s stiffness, as seen in the higher resilient modulus values. RCA mixtures can improve stiffness and performance, but the optimal RCA proportion depends on the RCA percentage, quality, and mortar content. The mixtures’ stiffness modulus decreased due to the fragility of the mortar adhering to the RCA after a certain percentage of RCA was reached (Acosta Alvarez et al. Citation2019). This implies that a completely recycled mixture may not compact well under loading, thus reducing stiffness. In contrast, some researchers found that RCA has a higher resilient modulus than NA, indicating that RCA can increase the stiffness of road base courses.
A number of studies have uncovered various trends. Alengaram et al. (Citation2011) confirmed the finding that the modulus of resilience decreases as the RCA content increases. Aytekin and Mardani-Aghabaglou (Citation2022) found that RCA outperforms NA in terms of the resilient modulus when used as a pavement material. Radević et al. (Citation2020) studied the influence of using RCA in road construction and discovered that the amount of RCA in the mixtures affects the stiffness modulus, with larger proportions of RCA resulting in reduced stiffness.
4.1.2. Plastic deformation test results
Plastic deformation tests were conducted on the samples made with various mixtures of NA and RCA. The results of the plastic deformation tests for the control samples at the repeated load cycle (N) also varied from 250 to 10,000 were given in .
Figure 5. Plastic deformation test results for the control mixtures.

The 100% NA mixture’s plastic deformation rose from 0.015 to 0.07 at 250–10000 load cycles in a linear pattern, suggesting a consistent response to stress. The 75% NA + 25% RCA mixture’s plastic deformation began at 0.05 and increased to 0.2 at 250–10000 load cycles. After 5000 load cycles, the rate of increase slows, suggesting a near-steady state of deformation. The 50% NA + 50% RCA mixture had a deformation rate of 0.01 at 250 load cycles, rising to 0.085 at 10,000 load cycles. This lower rate compared to the other mixtures may mean higher stress resistance. The 25% NA + 75% RCA blend showed distinct patterns. Deformation rose from 0.02 to 0.35 at 10,000 load cycles, but slowed after 5500 cycles and flattened after 7500 cycles. This behaviour suggests a shift in the material’s deformation mechanism due to the higher RCA proportion. The 100% RCA mixture had a low deformation rate that gradually increased until 4500 load cycles. After that, deformation appears to level off, possibly indicating the material has reached its plastic limit. The deformation was higher than before, indicating lower stress resistance. These trends illustrate how each mixture behaves under stress in the beginning (0 F-T cycle).
These findings suggest that RCA samples are more likely to experience plastic deformation than NA samples. The greater plastic deformation in RCA samples can be attributed to a variety of causes, including the breaking of cement mortar adhering to the coarse aggregate particles, the separation of coarse aggregate particles, the presence of initial defects, and the older mortar in the RCA (Akbas, Subasi, and Iyisan Citation2023; Jayakody, Gallage, and Ramanujam Citation2019). Vega Araujo et al. (Citation2022) have reported similar results and patterns. They noticed that RCA had superior mechanical characteristics compared to NA, but it had a greater amount of plastic deformation. Reis et al. (Citation2021) studied the effectiveness of RCA in the base and sub-base layers. The findings indicated that a combination of 25% recycled concrete aggregate and 75% natural aggregate had the same resilient response and plastic deformation properties as a dense-graded coarse aggregate base used in the base and sub-base layers.
4.2. Effect of freeze-thaw cycles on stiffness properties
This section outlines the experimental results from a thorough examination of the impact of F-T cycles on the stiffness characteristics of different pavement base-course mixtures. The Resilient Modulus (MR) and plastic deformation (εp) are essential metrics for assessing the stiffness characteristics of the mixtures. The impact of the F-T cycles was evaluated by subjecting the mixtures to 1, 5, 10, and 20 F-T cycles and then measuring the resilient modulus and plastic deformation.
4.2.1. Resilient modulus test results
Resilient Modulus Tests were conducted on base mixtures with varying NA and RCA proportions after 1, 3, 5, 10, and 20 F-T cycles to assess the effect of F-T cycles on stiffness, as indicated by MR. To compare stiffness across F-T cycles, MR values were normalised. The MR at each F-T cycle was divided by the initial MR value before any F-T cycles, giving the ratio (MRN/MR0). show the effect of F-T cycles on MR values of various mixtures in the 1st (21 kPa), 7th (69 kPa), and 12th (103 kPa) series, as well as normalised MR values.
The F-T cycles had an immediate effect on the 100% NA mixture, reducing the MR value to 52%. This decreased further to 39% by the 20th cycle, showing a marked decrease in stiffness. At the 20th F-T cycle, the 1st loading series, which had lower loading conditions, showed a normalised MR of 0.47 times the initial value, implying that stiffness decreases even under reduced loading. These results suggest that ice lenses during freeze-thaw cycles can cause cracks in the asphalt layers of the base course, degrading pavement quality (Li et al. Citation2013).
Unexpectedly, mixtures with RCA showed an increased MR value after the first F-T cycle. This was observed in 75% NA and 25% RCA, 50% NA and 50% RCA, and 100% RCA mixtures. Notably, the 50% NA and 50% RCA combination resulted in a significant normalised MR increase of 1.15. A balanced NA and RCA mixture may provide enhanced initial rigidity, exceeding the 75% NA + 25% RCA and 100% RCA blends. The 50% NA + 50% RCA blend showed a significant stiffness increase at the 5th F-T cycle, reaching 1.31 times the original value, suggesting a balance between freezing compaction and thawing destruction. When cement in samples interacts with water, it forms a sturdier material enduring certain freeze-thaw cycles due to ice grain cohesion, known as the consolidation effect. Hence, the blend attains maximum stiffness at this freeze-thaw cycle equilibrium point (Ghorbani et al. Citation2021; Monu et al. Citation2020).
After the 10th and 20th F-T cycles, the trend reversed and all mixtures showed a decrease in normalised MR values. For the 50% NA + 50% RCA mixture, MR dropped to 1.06 times the initial value at the 20th F-T cycle, implying that repeated F-T cycles overcame the initial consolidation effect, causing a net decrease in stiffness. Notably, the 25% NA + 75% RCA mixture showed better resilience to repeated F-T cycles. Its stiffness reduction was gradual, with a normalised MR of 1.04 even after 20 F-T cycles. The normalised MR after 20 F-T cycles in the 12th loading series was 1.05, suggesting it could be suitable for high-load applications. After 5 F-T cycles, the mixtures’ internal structure weakened due to limited pore space. Freezing caused moisture to move from the particles’ interior to the surface, due to a temperature gradient. Moisture migration causes mixture degradation due to pore water frost expansion and decreased matric suction from increased saturation. Water content affects the degree of change. However, in freezing and thawing cycles, which cause major soil structure changes, restoration is not possible (Gong et al. Citation2022; Ishikawa et al. Citation2019; Liu et al. Citation2016; Valenza and Scherer Citation2007; Wei et al. Citation2023). These findings show a complex, nonlinear relationship between F and T cycles, material composition, and stiffness. This highlights the need to consider these factors in road design and material selection.
Studies have examined F-T cycles’ effect on RCA materials’ MR values, with varied results. Soleimanbeigi et al. (Citation2015) studied climate’s influence on recycled unbound aggregates’ MR and found F-T cycles had minimal, possibly beneficial, effects on the RCA used in the base course. Đokić et al. (Citation2020) studied pavement mixtures of natural and RCA. RCA has F-T resistance, but lower fragmentation and water absorption limit its use. However, the tested mixtures, including RCA, showed good resistance to fragmentation and wear, suggesting RCA can be used in various pavement layers. Rosa et al. (Citation2017) found that RCA materials’ MR decreased after 5 F-T cycles, then increased by 35% after 20 cycles. Ghorbani et al. (Citation2020) showed that F-T cycles improved RCA MR, maintaining its stiffness even after 20 cycles. Diagne et al. (Citation2015) found that the resilient modulus decreased by 53% after 20 freeze-thaw cycles with 100% recycled concrete aggregate.
4.2.2. Plastic deformation test results
Plastic deformation tests were repeated on samples made with varying mixtures of NA and RCA after different numbers of F-T cycles, and the results are shown in . Additionally, to gain a better understanding of the variation in plastic deformation values (εp) with the mixing ratio and F-T cycles, the accumulated plastic strains at N = 1000, N = 5000, and N = 10000 are outlined in . The figure illustrates the normalised plastic deformation values, which were calculated by dividing the εp at each F-T cycle (εpN) by the initial εp value before any F-T cycle (εp0).
Figure 8. The influence of F-T cycles on the εp values and normalised values (εpN/εp0) of the mixtures across various loading series.

The study showed that NA and RCA mix proportions and load cycles affected plastic deformation. Generally, plastic deformation increased with more load cycles and freeze-thaw cycles. For 100% NA samples, one freeze-thaw cycle caused a large increase in deformation after the same number of load cycles. As the number of freeze-thaw cycles increased, deformation values rose. For example, after 1000 load cycles, deformation increased from 0.028 to 0.058, 0.159, 0.159, and 0.464 with 1, 5, 10, and 20 F-T cycles, respectively. The deformation at 20 F-T was significantly higher than 0 F-T. The large increase is due to cyclic loading and environmental conditions affecting the microstructure, causing higher plastic deformation.
This implies that the repeated freeze-thaw process could introduce microcracks or change the porosity of the material, thus weakening the structural integrity of the material. At 5000 load cycles, the deformation increased, as expected. However, the increase in deformation with an increasing number of F-T cycles is more profound, indicating that the cumulative damage of repeated loading and environmental stress leads to a significant increase in deformation. By 10,000 load cycles, the deformation continues to increase with an increase in the number of F-T cycles, reaching a notably high value at 20 F-T cycles.
The 75% NA + 25% RCA mix had higher deformation values than the other mixtures under similar loading and freeze-thaw conditions. At 1000 load cycles, deformation was 0.2 with 0 F-T, and increased to 0.25, 0.15048, 0.162, and 0.18 for 1, 5, 10, and 20 F-T cycles. RCA appears to make materials more deformable. At 5000 load cycles, deformation was higher at higher F-T cycles, showing a combined effect of mechanical and environmental stressors. By 10,000 load cycles, deformation kept rising, with the highest values at 20 F-T cycles.
For the 50% NA and 50% RCA mixtures at 1000 load cycles, the deformation was 0.04 under 0 F-T cycles, higher than other mixes. When the F-T cycles increased to 1, the deformation rose to 0.052. Surprisingly, the deformation decreased to 0.038 when the F-T cycles increased to 5. The deformation increased to 0.034 and 0.04 with 10 and 20 F-T cycles, respectively. This indicates that the 50% NA and 50% RCA mix may lose deformation resistance after a single F-T cycle, but regain some of it at higher F-T cycles. At 5000 load cycles, the deformation was 0.082, approximately double that at 1000 load cycles. Deformation rose to 0.066 at 1 F-T cycle, then decreased to 0.05325 at 5 F-T cycles. At 10 and 20 F-T cycles, deformation increased to 0.0575 and 0.093. At 10,000 load cycles, deformation at 0 F-T cycles rose to 0.085.
The deformation rose to 0.076 after 1 F-T cycle, then decreased to 0.065 after 5 F-T cycles, increased to 0.072 at 10 F-T cycles, and stayed at 0.118 at 20 F-T cycles. The 50% NA and 50% RCA mix followed a pattern similar to RMT, with deformation increasing after the first F-T cycle, then decreasing after 5 F-T cycles. After 5 F-T cycles, deformation increases, indicating that the material becomes more deformable with repeated freeze-thaw cycles. The results suggest a complex relationship between mechanical stress (load cycles) and environmental stress (freeze-thaw cycles) on the mix’s deformation. Further investigation may explore the microstructural changes at different F-T cycles to explain this behaviour. These findings may be relevant for using the mix in areas with large temperature fluctuations.
The 25% NA and 75% RCA samples had higher deformation values than lower RCA content samples at all load cycles. At 20 F-T cycles, the deformation values were especially high, suggesting higher vulnerability to mechanical and environmental stresses in RCA-dominant mixes. The 0 F-T sample had a deformation of 0.1 at 1000 load cycles. Introducing F-T cycles caused deformation to rise. After 1 cycle, deformation was 0.168, and after 5 cycles, it was 0.14142. At 10 F-T cycles, deformation was 0.224784, and at 20 F-T cycles, it was 0.09296. This suggests deformation generally increases with more F-T cycles, except for a slight decrease at 20 F-T cycles. After 5000 load cycles, the 0 F-T sample had a deformation of 0.3475. Increasing the F-T cycles to 1, 5, 10, and 20 yielded deformation values of 0.2268, 0.20566, 0.27552, and 0.37576, respectively. Deformation increased with more F-T cycles, which was more pronounced than in samples with higher NA, suggesting RCA’s physical characteristics may be a factor. The deformation values at 20 F-T cycles were much higher than 0, 1, and 5 F-T cycles, indicating repeated freeze-thaw cycles significantly affect plastic deformation. This suggests the material may not be suitable for environments with extreme temperature changes.
For 100% RCA samples, deformation may level off or even decrease after 5 F-T cycles at 10,000 load cycles, suggesting RCA-dominant mixes may be resilient to freeze-thaw cycling. At 1000 load cycles, the 0 F-T cycle had 0.05 deformation. After one F-T cycle, deformation rose to 0.16714. After 5 cycles, it was 0.17947, and after 10 and 20 cycles, it was 0.23153 and 0.28496, respectively. Deformation increased with more F-T cycles, showing the damaging effect of freeze-thaw action on the 100% RCA mix. At 5000 load cycles, deformation was 0.24 without F-T cycles. With one F-T cycle, deformation rose to 0.22468. An interesting pattern was seen where the deformation stayed the same at 5, 10, and 20 F-T cycles (0.34798). This implies that beyond one F-T cycle, the deformation did not change much. At 10,000 load cycles, the deformation rose to 0.2575 with 0 F-T cycles. The deformation decreased to 0.26304 after 1 F-T cycle and further to 0.23153 after 5 F-T cycles. An increasing trend was seen at 10 and 20 F-T cycles, reaching 0.305784 and 0.35483 respectively.The 100% RCA mix showed some resilience at lower F-T cycles and high-load cycles, but deformation increased with more F-T cycles. The deformation increased with more load cycles and F-T cycles, but not linearly, with some fluctuations at 5000 load cycles. This could be due to RCA’s complex freeze-thaw behaviour compared to NA.
The experiment showed that cement hardening and absorption affected plastic deformation values, similar to the resilient modulus test. Prolonged freeze-thaw cycles may make mixtures more prone to plastic deformation, possibly due to decreased particle contact, resulting in increased total deformation and decreased stiffness. Plastic deformation changes are due to particle contact area, interlocking, and particle packing organisation (Hao and Pabst Citation2023; Li et al. Citation2019).
This research’s results on plastic deformation in pavement materials due to freeze-thaw cycles are similar to earlier studies. Bozyurt et al. (Citation2012) discovered that RCA samples’ cumulative plastic deformation ranged from 0.5% to 0.8%. Ghorbani et al. (Citation2020) found that plastic deformation values of RCA samples decreased with more freeze-thaw cycles, which is consistent with this study. They reported a decrease in RCA plastic deformation after 10 F-T cycles when exposed to 0, 10, and 20 F-T cycles. RCA’s plastic deformation decreased 20% after 10 freeze-thaw cycles and dropped another 31% over the next 10 cycles.
5. Conclusion
The study assessed how freeze-thaw cycles (F-T) affect the resilience and deformation of pavement mixtures with varying Natural Aggregate (NA) and Recycled Concrete Aggregate (RCA) ratios. It tested 100% NA, 75% NA/25% RCA, equal parts, 25% NA/75% RCA, and 100% RCA mixtures, providing key insights.
F-T cycles alter material composition and stiffness. All mixtures underwent stiffness changes due to F-T cycles. 100% NA mixtures softened, while RCA blends initially hardened. However, repeated F-T cycles eventually reduced stiffness, indicating that F-T cycle damage exceeded consolidation effects.
Mixtures with 50% NA + 50% RCA and 25% NA + 75% RCA had superior stiffness properties and highest MR values, indicating excellent initial stiffness and F-T cycle resistance.
100% RCA mixtures had lower MR values, suggesting they may not have the best stiffness properties. This could be due to RCA’s unique characteristics, which may not compact as well under loading, resulting in less stiffness.
Higher loads and freeze-thaw cycles caused more plastic deformation, especially in mixtures with more RCA. This implies mixtures with higher RCA content are more vulnerable to mechanical and environmental stress.
The 50% NA + 50% RCA and 100% RCA mixtures showed initial deformation increase after the first F-T cycle, but regained resistance at higher F-T cycles. This highlights the intricate interplay between mechanical and environmental stressors in shaping deformation behaviour.
RCA-dominant mixtures, notably 25% NA + 75% RCA and 100% RCA, behaved uniquely. Deformation values increased under F-T cycles, indicating higher susceptibility to stresses. However, 100% RCA samples withstood F-T cycling, particularly after 5 cycles.
Results revealed the interactions between mechanical loading and environmental factors in base-course materials with different NA and RCA ratios. This highlights the importance of considering these dynamics when selecting materials for road designs, especially in areas with large temperature changes. Further research is suggested, such as studying microstructural changes in the mixtures at different F-T cycles. This could optimise material properties and performance.
Acknowledgments
Merve AKBAS: Formal Analysis, Data Curation, Investigation, Resources,
Writing - Original Draft.
Recep IYISAN: Conceptualisation, Validation, Visualisation, Writing - Review & Editing, Supervision, Project Administration.
Disclosure statement
No potential conflict of interest was reported by the author(s).
Additional information
Notes on contributors
Merve Akbas
Merve Akbaş is a research assistant in the Civil Engineering department at İstanbul Technical University. Her research interests include Soil Mechanics, Geotechnical Engineering, Environmental Geotechnics, and Sustainability.
Recep Iyisan
Recep Iyisan is a professor in the Civil Engineering department at İstanbul Technical University. His research interests encompass Earthquake Engineering, Soil Mechanics, and Environmental Geotechnics.
References
- Acosta Alvarez, D., A. Alonso Aenlle, A. J. Tenza-Abril, and S. Ivorra. 2019. “Influence of Partial Coarse Fraction Substitution of Natural Aggregate by Recycled Concrete Aggregate in Hot Asphalt Mixtures.” Sustainability 12 (1): 250. https://doi.org/10.3390/su12010250.
- Aghililotf, M., M. Palassi, and A. M. Ramezanianpour. 2021. “Mechanical and Durability Assessment of Unconfined Recycled Concrete Aggregates and Natural Aggregates Used in Road Constructions.” International Journal of Pavement Engineering 22 (12): 1518–1530. https://doi.org/10.1080/10298436.2019.1701190.
- Akbas, M., O. Subasi, and R. Iyisan. 2023. “The Effect of RCA Pavements on the Liquefaction-Induced Settlement.” Scientific Reports 13 (1): 6944. https://doi.org/10.1038/s41598-023-34239-z.
- Alengaram, U. J., A. Jumaat, M. Z. Salam, F. F. Jaafar, H. B. Saad, and H. B. Saad. 2011. “Properties of High-Workability Concrete with Recycled Concrete Aggregate.” Materials Research 14 (2): 248–255. https://doi.org/10.1590/s1516-14392011005000039.
- Aytekin, B., and A. Mardani-Aghabaglou. 2022. “Sustainable Materials: A Review of Recycled Concrete Aggregate Utilization as Pavement Material.” Transportation Research Record 2676 (3): 468–491. https://doi.org/10.1177/03611981211052026.
- Bamigboye, G. O., D. E. Bassey, D. O. Olukanni, B. U. Ngene, D. Adegoke, A. O. Odetoyan, and A. T. Nworgu. 2021. “Waste Materials in Highway Applications: An Overview on Generation and Utilization Implications on Sustainability.” Journal of Cleaner Production 283:124581. https://doi.org/10.1016/j.jclepro.2020.124581.
- Bestgen, J. O., M. Hatipoglu, B. Cetin, and A. H. Aydilek. 2016. “Mechanical and Environmental Suitability of Recycled Concrete Aggregate as a Highway Base Material.” Journal of Materials in Civil Engineering 28 (9): 04016067. https://doi.org/10.1061/(ASCE)MT.1943-5533.0001564.
- Bilodeau, J. P., G. Doré, and C. Schwarz. 2011. “Effect of Seasonal Frost Conditions on the Permanent Strain Behaviour of Compacted Unbound Granular Materials Used as Base Course.” International Journal of Pavement Engineering 12 (5): 507–518. https://doi.org/10.1080/10298436.2011.552605.
- Bozyurt, O., J. M. Tinjum, Y. H. Son, T. B. Edil, and C. H. Benson. 2012. “Resilient Modulus of Recycled Asphalt Pavement and Recycled Concrete Aggregate.” GeoCongress 2012: State of the Art and Practice in Geotechnical Engineering 3901–3910. https://doi.org/10.1061/9780784412121.400.
- Diagne, M., J. M. Tinjum, and K. Nokkaew. 2015. “The Effects of Recycled Clay Brick Content on the Engineering Properties, Weathering Durability, and Resilient Modulus of Recycled Concrete Aggregate.” Transportation Geotechnics 3:15–23. https://doi.org/10.1016/j.trgeo.2014.12.003.
- Đokić, O., A. Radević, D. Zakić, and B. Đokić. 2020. “Potential of Natural and Recycled Concrete Aggregate Mixtures for Use in Pavement Structures.” Minerals 10 (9): 744. https://doi.org/10.3390/min10090744.
- Edil, T. B., B. Cetin, and A. Soleimanbeigi. 2018. “Laboratory and Field Performance of Recycled Aggregate Base in a Seasonally Cold Region.” Sciences in Cold and Arid Regions 9:183–191. https://doi.org/10.3724/SP.J.1226.2017.00183.
- Ghorbani, B., A. Arulrajah, G. Narsilio, and S. Horpibulsuk. 2020. “Experimental Investigation and Modelling the Deformation Properties of Demolition Wastes Subjected to Freeze–Thaw Cycles Using ANN and SVR.” Construction and Building Materials 258:119688. https://doi.org/10.1016/j.conbuildmat.2020.119688.
- Ghorbani, B., A. Arulrajah, G. Narsilio, S. Horpibulsuk, and M. Leong. 2021. “Resilient Moduli of Demolition Wastes in Geothermal Pavements: Experimental Testing and ANFIS Modelling.” Transportation Geotechnics 29:100592. https://doi.org/10.1016/j.trgeo.2021.100592.
- Gokce, A., S. Nagataki, T. Saeki, and M. Hisada. 2011. “Identification of Frost-Susceptible Recycled Concrete Aggregates for Durability of Concrete.” Construction and Building Materials 25 (5): 2426–2431. https://doi.org/10.1016/j.conbuildmat.2010.11.054.
- Gong, F., S. Jacobsen, P. Li, Z. Wang, K. Maekawa, and M. Koniorczyk. 2022. “Modeling of Path-Dependent Phase Change in Sorption and Freezing of Pore Water for Cementitious Materials.” Journal of Building Engineering 57:104969. https://doi.org/10.1016/j.jobe.2022.104969.
- Gong, F., E. Sicat, D. Zhang, and T. Ueda. 2015. “Stress Analysis for Concrete Materials Under Multiple Freeze-Thaw Cycles.” Journal of Advanced Concrete Technology 13 (3): 124–134. https://doi.org/10.3151/jact.13.124.
- Haider, I., B. Cetin, Z. Kaya, M. Hatipoglu, A. Cetin, and H. A. Ahmet. 2014. “Evaluation of the Mechanical Performance of Recycled Concrete Aggregates Used in Highway Base Layers.” Geo-Congress 2014: Geo-Characterization and Modeling for Sustainability 3686–3694. https://doi.org/10.1061/9780784413272.357.
- Hao, S., and T. Pabst. 2023. “Experimental Study and Mathematical Description of Gradation Effect on the Mechanical Characteristics of Crushed Waste Rocks.” International Journal of Geomechanics 23 (2): 04022276. https://doi.org/10.1061/(ASCE)GM.1943-5622.0002610.
- Ishikawa, T., T. Lin, S. Kawabata, S. Kameyama, and T. Tokoro. 2019. “Effect Evaluation of Freeze-Thaw on Resilient Modulus of Unsaturated Granular Base Course Material in Pavement.” Transportation Geotechnics 21:100284. https://doi.org/10.1016/j.trgeo.2019.100284.
- Jayakody Arachchige, S. P., C. Gallage, and A. Kumar. 2012. “Assessment of Recycled Concrete Aggregates for Road Base and Sub-Base.” In Proceedings of the second international conference on geotechnique, construction materials and environment, Japan, 575–579. The GEOMATE International Society.
- Jayakody, S., C. Gallage, and J. Ramanujam. 2019. “Performance Characteristics of Recycled Concrete Aggregate as an Unbound Pavement Material.” Heliyon 5 (9): e02494. https://doi.org/10.1016/j.heliyon.2019.e02494.
- Jitsangiam, P., K. Boonserm, T. Phenrat, S. Chummuneerat, P. Chindaprasirt, and H. Nikraz. 2015. “Recycled Concrete Aggregates in Roadways: Laboratory Examination of Self-Cementing Characteristics.” Journal of Materials in Civil Engineering 27 (10): 04014270. https://doi.org/10.1061/(asce)mt.1943-5533.0001245.
- Li, Z., L. Liu, S. Yan, M. Zhang, J. Xia, and Y. Xie. 2019. “Effect of Freeze-Thaw Cycles on Mechanical and Porosity Properties of Recycled Construction Waste Mixtures.” Construction and Building Materials 210:347–363. https://doi.org/10.1016/j.conbuildmat.2019.03.184.
- Li, L., S. Saboundjian, J. Liu, and X. Zhang. 2013. “Permanent Deformation Behavior of Alaskan Granular Base Materials.” ISCORD 2013: Planning for Sustainable Cold Regions 428–435. https://doi.org/10.1061/9780784412978.042.
- Liu, K., J. Yan, Q. Hu, Y. Sun, and C. Zou. 2016. “Effects of Parent Concrete and Mixing Method on the Resistance to Freezing and Thawing of Air-Entrained Recycled Aggregate Concrete.” Construction and Building Materials 106:264–273. https://doi.org/10.1016/j.conbuildmat.2015.12.074.
- Li, L., Y. Yang, Y. Gao, and Y. Zhang. 2021. “Healing Characterisations of Waste-Derived Bitumen Based on Crack Length: Laboratory and Modelling.” Journal of Cleaner Production 316:128269. https://doi.org/10.1016/j.jclepro.2021.128269.
- Meesala, C. R. 2019. “Influence of Different Types of Fiber on the Properties of Recycled Aggregate Concrete.” Structural Concrete 20 (5): 1656–1669. https://doi.org/10.1002/suco.201900052.
- Monu, K., G. R. RN, G. S. Pandey, and S. Singh. 2020. “Performance Evaluation of Recycled-Concrete Aggregates and Reclaimed-Asphalt Pavements for Foam-Mix Asphalt Mixes.” Journal of Materials in Civil Engineering 32 (10): 04020295. https://doi.org/10.1061/(ASCE)MT.1943-5533.0003356.
- Radević, A., I. Isailović, M. P. Wistuba, D. Zakić, M. Orešković, and G. Mladenović. 2020. “The Impact of Recycled Concrete Aggregate on the Stiffness, Fatigue, and Low-Temperature Performance of Asphalt Mixtures for Road Construction.” Sustainability 12 (10): 3949. https://doi.org/10.3390/su12103949.
- Reis, G. S. D., M. Quattrone, W. M. Ambrós, B. Grigore Cazacliu, and C. Hoffmann Sampaio. 2021. “Current Applications of Recycled Aggregates from Construction and Demolition: A Review.” Materials 14 (7): 1700. https://doi.org/10.3390/ma14071700.
- Rosa, M. G., B. Cetin, T. B. Edil, and C. H. Benson. 2017. “Freeze–Thaw Performance of Fly Ash–Stabilized Materials and Recycled Pavement Materials.” Journal of Materials in Civil Engineering 29 (6): 04017015. https://doi.org/10.1061/(ASCE)MT.1943-5533.0001844.
- Saberi, S. S., A. Mohamed, and A. S. Eltwati. 2021 August. “Mechanical and Physical Properties of Recycled Concrete Aggregates for Road Base Materials.” Journal of Physics Conference Series 1973 (1): 012236. IOP Publishing. https://doi.org/10.1088/1742-6596/1973/1/012236.
- Soleimanbeigi, A., R. F. Shedivy, J. M. Tinjum, and T. B. Edil. 2015. “Climatic Effect on Resilient Modulus of Recycled Unbound Aggregates.” Road Materials and Pavement Design 16 (4): 836–853. https://doi.org/10.1080/14680629.2015.1060250.
- Toka, E. B., and M. Olgun. 2022. “Performance of Granular Road Base and Sub-Base Layers Containing Recycled Concrete Aggregate in Different Ratios.” International Journal of Pavement Engineering 23 (11): 3729–3742. https://doi.org/10.1080/10298436.2021.1916819.
- Valenza, J. J., II, and G. W. Scherer. 2007. “A Review of Salt Scaling: II. Mechanisms.” Cement and Concrete Research 37 (7): 1022–1034. https://doi.org/10.1016/j.cemconres.2007.03.003.
- Vega Araujo, D., J. E. Salcedo Fontalvo, R. Jimenez, D. M. Palacios Del Barre, and C. A. Fresneda Saldarriaga. 2022. “Granular Subbase Improvement with Recycled Concrete Aggregates in Tropical Areas.” IIUM Engineering Journal 23 (2): 59–71. https://doi.org/10.31436/iiumej.v23i2.2367.
- Wang, M., Q. Yu, Y. Xiao, and W. Li. 2022. “Resilient Modulus Behavior and Prediction Models of Unbound Permeable Aggregate Base Materials Derived from Tunneling Rock Wastes.” Materials 15 (17): 6005. https://doi.org/10.3390/ma15176005.
- Wei, Z., W. Yang, C. Zhai, Y. Sun, W. Tang, A. Chen, and Y. Wang, Y. Wang. 2023. “Freezing Characteristics and Microstructural Damage Evolution of Granular Materials in Cold Regions Under Freezing–Thawing Cycles.” Environmental Earth Sciences 82 (7): 191. https://doi.org/10.1007/s12665-023-10865-8.
- Wu, S., and C. Marty. 2023. “Three-Year Field Performance of a Low Volume Road with 100% Reclaimed Asphalt Pavement Cold Mix with Rejuvenator.” Transportation Research Record 2677 (7): 532–544. https://doi.org/10.1177/03611981231153648.
- Zaharieva, R., F. Buyle-Bodin, and E. Wirquin. 2004. “Frost Resistance of Recycled Aggregate Concrete.” Cement and Concrete Research 34 (10): 1927–1932. https://doi.org/10.1016/j.cemconres.2004.02.025.
- Zhi, D., F. Gong, Z. Wang, Y. Zhao, and T. Ueda. 2023. “RBSM-Based Mesoscale Study of Frost Deterioration for Recycled Concrete Considering Air-Entrainment in Old and New Mortar.” Journal of Building Engineering 68:106210. https://doi.org/10.1016/j.jobe.2023.106210.