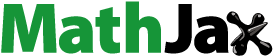
ABSTRACT
The capture of carbon and sequestration (CCS) activity is considered strategic in the context of world energy policy. In fact, CO2 emissions from fossil/conventional-fuel-fired power plants can be lowered by using CCS on the same. Various other methods have been developed to date to capture the carbon and store it. This article focuses on the various carbon capture technologies and the storage technologies such as pre-combustion, post-combustion, oxyfuel technology, and direct air capture (DAC) technology, including their subparts, along with the factors affecting the carbon capture technologies. The aim of the present study is to develop an overview of carbon dioxide removal (CDR) technologies and CO2 sequestration, including a vast coverage of the various factors that have a huge impact on CCS. It emerged that the existing technologies that deal with CO2 sequestration and capture are being used at large scale to produce derivatives including chemicals, polymers, building materials, and various other products. The newest technology that has been seen creating a huge effect is direct air capture, and commercial use of such technologies has been seen. Future potential application areas have been realised in this review work. In addition, this article explores policy recommendations for the future.
1. Introduction
During the recent decade, climate change has transformed into a worldwide concern issue from a peripheral matter since 2014–2018 was reported to be one of the warmest periods of history (Butler Citation2018). At Paris international climate summit in 2015, 177 governments decided on acting together to limit global warming rise below 2°C by 2030, lowering it to 1.5 °C by 2050. Although the Paris Agreement was the first global general agreement containing policy commitments on global warming, the pattern for mitigating global atmospheric warming goes back over 3 decades with the foundation of the Intergovernmental Panel on Climate Change (IPCC 1990) and the United Nations Framework Convention on Climate Change (UNFCCC Citation1992) (Palermo and Hernandez Citation2020). Later, the United Nations Climate Change Conference of the Parties (COP) has been held yearly by the top decision-making body of UNFCCC.
As it is well-known, the main cause of the global atmospheric temperature increasing is the concentration of greenhouse gases (GHGs) principally carbon dioxide (CO2) (Ledley et al. Citation1999; Montzka, Dlugokencky, and Butler Citation2011). The environmental CO2 concentration has been reaching more than 415 parts per million (ppm) in 2021, 50 percent more than the same quantity in 1995. According to the latest UN Environment Programme Emission Gap Report (UNEP Citation2021) (Olhoff and Christensen Citation2018), total GHG emissions should be limited to 39 Gt CO2 eq in 2030 to meet the agreed-upon climate goal of 2°C, but with present policies, it will be anticipated to be approximately 55 Gt, resulting in a roughly 15 Gt emission gap. As a result, more severe measures to mitigate the current trend of climate change should be implemented in those sectors, such as increasing the use of non-fossil fuel energy, using electric vehicles, imposing a carbon tax per unit of CO2 emission on those regions with high contributions, producing more sustainable construction materials, and carbon capture/storage technologies. In addition, it must be noted that most of the electricity generated today comes from fossil fuels such as coal and natural gas. However, the increasing adoption of renewable energy sources, such as solar and wind power, is reducing the proportion of electricity generated from fossil fuels. Additionally, many electric vehicle charging stations are powered by renewable energy sources, and some electric utilities offer customers the option to purchase renewable energy specifically to charge their electric vehicles. In the building sector, embodied carbon from building and construction materials accounts for over 10% of yearly worldwide GHG emissions, raising CO2 levels in the atmosphere (Churkina et al. Citation2020). The cement and concrete sectors emitted about 7% of this total. Cement production was responsible for 5% of global fossil CO2 emissions in 2020 (Mahasenan, Smith, and Humphreys Citation2003). Further, new methods are defined to reach net-zero emissions in 2050, notably employing CCS technology which can account for 42% of the carbon reduction. Energy-intensive manufacturing sectors such as cement require enough infrastructure to transport, re-use, and store the CO2 captured.
In this context, it becomes essential to minimise carbon emissions and remove CO2 from the atmosphere. One viable alternative is exploiting CO2 as a resource (Brennan and Owende Citation2010; Markewitz et al. Citation2012; Peters et al. Citation2011). In CCS technology the CO2 from the emitters is collected, conserved, and utilised in new products and services such as energy fuels (Rissman et al. Citation2020). Because of the high cost, as well as the technological and societal constraints, the other reduction options are yet favoured, and hence, the CCS technologies are viewed as the ultimate solution, still under research and development (Durmaz Citation2018). Academic organisations have proposed numerous CO2 reduction programmes and solutions as part of their efforts to combat global warming (Hansen et al. Citation2000). The carbon capture and use or storage method, which is a significant technology-driven mitigation option that turns CO2 from the exhaust into stored CO2 or value-added goods, is one of the options. Value-added goods, also known as value-added products or services, are items that have been modified or enhanced in some way to increase their value to the consumer. Depending on their life cycle, these goods can be a sustainable and environment-friendly option, as they can extend the lifespan of a product, reduce waste, and decrease the need for new resources. However, it is important to consider the full life cycle of value-added goods, including the materials and energy used in their production, transportation, use, and disposal. When the embedded carbon that was captured during the growth in use of the raw materials such as wood, plastic, and metal, used in the production of value-added goods is released back to the atmosphere at the end of their life cycle, it could contribute to climate change. Therefore, it is essential to carefully consider the environmental impact of value-added goods throughout their entire life cycle, and to select materials and production methods that minimise carbon emissions and minimise the environmental footprint. Additionally, it is important to promote practices that allow for the recovery of materials and energy at the end of their life cycle, such as recycling and composting. But because of its low sequestered levels and the need for a large amount of CO2 to satisfy climate change targets, it is not a substitute for CCS.
Using CO2 to make methanol and urea has a sequestration potential of less than 0.5 percent of total anthropogenic CO2 emissions. Furthermore, CCU is acknowledged as a crucial mitigating situation when storage options are limited. It’s estimated that by 2060, the International Energy Agency’s (IEA) Clean Technology Scenario (CTS) will use 250 million tonnes of CO2 per year in the conversion to fuels and chemicals (Ghiat and Al-Ansari Citation2021; Song Citation2006). CO2 sequestration through this CCU pathway can reach 878 Mt/year by 2060 with a storage limit of 10 Gt/year by 2060 added to this scenario. Depending on how the final product is used, CCU systems can store CO2 for a range of time periods. While most CCU methods only offer short-term sequestration, enhanced oil recovery, and mineralisation are long-term alternatives that can store CO2 for decades (Al‐Mamoori et al. Citation2017). CO2 sequestration through this CCU pathway can reach 878 Mt/year by 2060 with a storage limit of 10 Gt/year by 2060 added to this scenario depending on how the final product is used, CCU systems can store CO2 for a range of time periods.
While most CCU methods only offer short-term sequestration, enhanced oil recovery, and mineralisation are long-term alternatives that can store CO2 for decades (Al‐Mamoori et al. Citation2017). It is a known fact that CO2 is also used for producing Methanol, formaldehyde, dimethyl ether, light olefins, and gasoline. In addition, various researchers have merged CO2 and hydrogenation in which it has been used to produce formate and CH3OH (Kar, Goeppert, and Prakash Citation2019). In another research, the CO2 capture has been achieved with the help of polyethylene glycol (PEG) and its subsequent conversion has been carried out. The PEG/superbase was shown to be highly effective for quick, reversible, equimolar CO2 absorption. At mild reaction conditions, collected CO2 activated with DBU/PEG could be catalytically transformed into functional organic compounds (Yang et al. Citation2011). One research discovered that commercially obtained amino acid salts with a bulky N-group have an absorption capability that approaches equimolar levels in poly(ethylene glycol) (PEG) solution (Liu et al. Citation2012). Production of concrete has also been another research going on in the are of carbon capture. Some companies have recently invested in companies like Solidia and CarbonCure which use carbon dioxide to produce concrete. With a USD 20 million global competition, the NRG COSIA Carbon XPrize supports the development of new CO2 use prospects in North America. Canada, Japan, and the United Kingdom are among those countries that have invested much in research and development for CO2 utilisation. Several considerations are motivating a growing amount of attention to be paid to CO2 utilisation options. Its ability to aid in the achievement of climate change mitigation objectives is one of its most important features. Among the other motivations is technological leadership, the projected availability of low-priced and ample renewable energy (which could make CO2 conversion routes more economical), and the potential for CO2 utilisation to be either a stepstone or a small-scale substitute to CCS CO2 can be used commercially in some circumstances, such as in the production of building materials because it provides a superior product at a low cost than traditionally produced products. Products that require carbon, either because it provides their structure and qualities (carbon-containing compounds) or because the usage of carbon-free energy carriers, such as hydrogen or electricity, is difficult, could benefit from CO2 (for example, aviation fuels) (Muradov and Veziroğlu Citation2008). But the problem which is being faced nowadays is the greenhouse gases being generated in from the concrete. Greenhouse gases (GHGs), primarily nitrous oxide, CO2, and methane, can absorb a certain amount of heat depending on three molecular structures, which shapes the global warming impact (Nelson et al. Citation2004). The earth’s insulating blanket is overburdened with heat if the concentration of molecules in these gases is high, producing global warming (Sheldon and Crimmin Citation2022). The following is the percentage contribution to the greenhouse effect: Carbon dioxide accounts for 53%, methane for 17%, CFCs (chlorofluorocarbons) for 12%, nitrous oxide for 6%, and others for 12% (Dreyfus et al. Citation2022). In the case of CFCs, CFCs are a group of chemicals commonly used as refrigerants, solvents, and propellants. They have been phased out because they have been found to deplete the ozone layer, which protects the Earth from harmful ultraviolet radiation. As a result, the 1987 Montreal Protocol was established to phase out the production and consumption of CFCs Fluorocarbons (HFCs) are one of the most common alternatives to CFCs, as they do not deplete the ozone layer. However, HFCs are potent greenhouse gases, which means they contribute to global warming. Therefore, some countries are phasing out HFCs as well and promoting the use of more environmentally friendly alternatives such as hydrocarbons (HCs) and natural refrigerants like ammonia, carbon dioxide and hydrocarbons. Yet, natural refrigerants such as carbon dioxide are also utilised in modern revolutionary technology. These refrigerants may not be fully safe or environmentally benign, but they are regarded superior to conventional CFC refrigerants. As employing carbon dioxide as a natural refrigerant provides advantages over alternative non-environmentally friendly refrigerants, it is preferable to use carbon dioxide. As a result, using carbon capture technologies to reduce GHG emissions has become a necessity, and this article concentrates on carbon capture technologies and the use of the captured carbon in various applications. However, it has been discovered that there are three primary options for reducing carbon emissions from energy generation: (a) improving energy efficacy; (b) switching to zero/low emissions renewable resources; and (c) carbon capture, storage, and utilisation (which is the major focus of this article). Because of the foregoing, large-scale applications such as energy-intensive industries and power plants have limited possibilities for energy efficiency and conservation. Furthermore, due to topographical, technical, and social barriers, the transition to renewable energy is impeded in some areas. The topographical barriers in producing efficient energy through power plants can limit the availability of suitable locations for construction and increase the costs of transporting fuel and equipment. For example, if a fuel source is located far from a potential power plant site, the cost of transportation can be significant. Natural disasters can also disrupt power plant operations and lead to costly repairs or rebuilds. Technical barriers can be substantial, as updating infrastructure and technology can require large investments and pose design and operational challenges. For example, upgrading emissions control systems to meet stricter environmental regulations can be technically complex and costly. Additionally, the high capital costs associated with building and maintaining power plants can be a major barrier to efficiency. Social barriers can also play a significant role in power plant production. Public opposition to new construction, particularly in regard to environmental concerns, can result in delays or cancellations of projects. Competition for resources such as water and land can also limit power plant efficiency. Furthermore, economic considerations such as access to financing and energy market prices can impact the feasibility of projects, while political and policy issues can create regulatory barriers to efficiency. Further, there are a few well-known approaches for CCS technologies to be implemented in power plants that will be covered in the next sections: pre, post, oxy-fuel combustion, and DAC technique (Dixit et al. Citation2022). Furthermore, unique materials to be used in this sequestration process have been introduced such as bioinspired materials (BMs), and mineral wastes including the effect and performance of adsorbents and absorbents in the carbon capture and sequencing (Rego, Kurkuri, and Kigga Citation2021). In addition, adsorption and absorption are also strategies that come under post-combustion carbon capture including membrane separation and chemical capture (Liang et al. Citation2015). Further, in next the sections, various CDR and CCS technologies have been reviewed, explored, and discussed in depth. A special focus has been made on DAC methods which take the ambient air into account and process the capture carbon dioxide for further use which is in line with the SDG initiative started by the EU for the betterment of society towards sustainable development in the European union. According to this programme, there are various goals that have been set up by the EU member states to achieve a better solution including reducing poverty, health, climate change, and many more. This research article hence works towards one of the premier goals of the EU which is ‘Climate action’ which is towards controlling climate change through various actions Massai and Beyet (Citation2018). The aim of the paper is to explore the CCS and CDR technologies being used nowadays. Since the carbon present is posing threat to the environment. This article will be focused on the use of present technology to capture and enhance the combined effect of CCS and CDR on the environment. It should be noted that CDR is different from point-source CCS for heavy industry and fossil fuel power plants. CDR includes a wide range of methods that directly collect CO2 from the atmosphere and then permanently store it, producing negative emissions. CDR can handle residual CO2 emissions from industries that are difficult to decarbonise (including agriculture, aviation, and shipping), gradually removing them from the atmosphere. Prior to entering the environment, CO2 is captured by CCS in fossil power plants or heavy industries; CCS is a technique to lower CO2 emissions and can aid in achieving deep decarbonisation in the current power and industrial sectors.
The rest of the paper is organised as follows: in section 2, the manuscript discusses the various carbon capture technologies and an overview of them. Further in section 3, various uses of the captured carbon have been explained along with carbon derivatives. Section 4 discusses the different factors affecting carbon capture technologies including adsorbents. Section 5 and section 6 explain the new opportunities related to the captured carbon and the application potential of the captured carbon have been explained respectively. Finally, Section 7 concludes the manuscript with concluding remarks on factors that affect carbon capture technologies and their types. In the end, this paper finalises with a discussion of the application potential of carbon capture technologies and the related opportunities.
2. Carbon capture, storage, and technologies
As it has been seen that carbon dioxide in the air acts as greenhouse gases and those greenhouse gases are one of the major reasons for climate change including Earth’s magnetic field changes (Mikhaylov et al. Citation2020). In the past, various technologies have been invented and used for the storage and use of carbon. One of the methods to reduce the carbon in the environment is the permanent storage of carbon dioxide. Permanent storage is considered one of the best methods to reduce or eliminate carbon dioxide in the air, but the cost of storage plays a major role in capturing and storing carbon (Alturki Citation2022). Direct air carbon capture and storage method (DACCS) is a method that is used for capturing the CO2 from the air and store under the earth more than a mile underground (Gutknecht et al. Citation2018). This work has already been started at the industry level and companies have already started using these facilities for carbon storage underground specifically named the Orca plant situated in Iceland (O’Neill Citation2021), which captures up to 4,000 tons of CO₂ per year. Both CCS and CDR technology have their benefits and drawbacks. CDR and carbon capture and storage (CCS) are two methods that work together to get remove and store CO2.
Although there are alternative methods for removing CO2, carbon capture and storage (CCS) is currently the only one that can effectively lower emissions from industrial plants. Capturing CO2 at these specific sources and safely storing it underground is CCS’s greatest strength. Furthermore, the low quantity of CO2 in the atmosphere is one of the key drawbacks of extracting CO2 from the air through methods like direct air capture. Pre-combustion CCS involves processing fuel to produce a combination of hydrogen and carbon monoxide before combustion. Syngas is the product of the mixture’s reaction with water, which produces hydrogen and extremely concentrated carbon dioxide. Oxygen is utilised to burn the fuel in the CCS process of oxyfuel combustion, and the resulting exhaust gas has an extremely high concentration of CO2. This greatly facilitates the CCS technique by which CO2 is reacted with the sorbent before being separated. The utilisation of high amounts of oxygen in oxyfuel burning results in a large reduction of nitrogen oxide (NOx) and sulphur dioxide emissions. CCS indeed has some benefits, but it also has several drawbacks that we have discussed. Without government subsidies, the price of the end product will rise as existing factories and power plants adopt CCS technology. Enhanced oil recovery is one current application of the CO2 captured using CCS. Captured carbon dioxide is then injected into depleted oil wells by oil corporations, releasing oil that would have otherwise been inaccessible without the technique. More carbon dioxide (CO2) will be released into the air when that oil is eventually burned. If the CO2 caught through CCS is not equal to the CO2 emitted by the oil that was made available, then CCS will only increase the amount of greenhouse gas in the atmosphere. It’s also unclear how well CO2 can be stored for the foreseeable future. Locations of CO2 transportation and storage may pose threats, however, uncommon accidents may be during CO2 transportation, and there is always the chance that a leak could cause serious harm. There are a few technologies used for carbon sequencing namely Adsorption, Absorption, Membrane separation, and Chemical capture (Hussin and Aroua Citation2020) which are discussed in the next sections. In the next section, CCS technologies are listed along with an overview of the technologies (). One point which must be noted is that carbon use can complement carbon storage but not the other way around. Carbon storage is one of the options, but the use of carbon is another option that is more reliable than storage.
Figure 1. Overview of technologies (author’s elaboration).

2.1. Technology overview
The significant increase in greenhouse gas concentrations has raised environmental worries. Global warming is mostly caused by greenhouse gases (Shen et al. Citation2020; Yoro and Daramola Citation2020). More efficient energy use, the promotion of renewable energy and alternative fuels, and the use of CCS technologies can all help to reduce greenhouse gas emissions. This section explores the CCS technologies used for carbon capture and storage, as well as additional applications such as conversional and non-conversional use (see ). After the combustion process, CO2 is collected from the escaping flue gas using solvents (most preferably chemical) which is known as post-combustion capture. In the oxyfuel combustion process, oxygen in pure form is used. The term ‘recycled flue gas’ (RFG) refers to a portion of flue gas that is recycled to lower the temperature of the flame. CO2 and water vapour make up most of the flue gas produced at the exhaust. As a result, CO2 can be isolated easily by condensing water vapour.
CCS has emerged as the most effective approach for reducing greenhouse gas emissions. CCS technologies, according to the IEA, would remove roughly 20% of greenhouse gas emissions by 2050 IEA (Citation2019b). There is a need to reduce the energy penalties incurred because of CCS. When compared to conventional facilities, the adoption of Carbon Capture and Storage (CCS) technology in energy intensive sectors and power plants incurs significant capital expenditures (CAPEX) and operational expenditures (OPEX) (Yang et al. Citation2021). The increased costs are mostly due to the requirement for specialised equipment, such as capture systems, compression systems, and storage facilities, as well as increased energy usage to run the CCS process.
Another disadvantage of CCS technology is the added expense, which makes these technologies uncommon in large-scale industrial operations. CO2 capture accounts for about 75% of total CCS costs, making it the most expensive component of the technology (Yadav and Mondal Citation2022). The emission of carbon in the natural environment has created problems including greenhouse gas and climate change due to temperature change. It is evident from various scenarios that carbon dioxide is one of the major constituents which is creating more problems (Dias De Oliveira, Vaughan, and Rykiel Citation2005; Yang et al. Citation2008). Researchers around the world have observed this problem and started finding a solution to this. Moreover, scientists around the world have found different ways to capture carbon to save the environment. There are different technologies and methods which have evolved to date that not only capture the carbon but also try to use that captured carbon for different other applications (Reis et al. Citation2004). Some of the processes are listed and discussed in this section.
When evaluating the climate benefits of CO2 consumption, there are five main factors to consider:
CO2 is produced by several sources (from natural resources, conventional fuels, the air, and biomass), in this point the sources from which CO2 is being captured must be considered.
The service or product that is being replaced with a CO2-based product or service. Since CO2 is being used in producing products as well, this point explains the traditional product which are being replaced by product produced using CO2
Amount and type of energy in which CO2 is converted? Since CO2 is converted in other type of energies, this has to be taken into account in which type of energy it is being converted.
For how much time the carbon is retained in the product? Since there are various products that has been made from the use of the CO2, the time for which the carbon is retained by that product, has to be noticed.
The scope of the CO2 utilisation opportunity. The scope of the opportunity for CO2 utilisation is broad and growing as the need to reduce greenhouse gas emissions becomes more pressing. Some potential areas of CO2 utilisation include:
Enhanced oil recovery: CO2 can be injected into depleted oil reservoirs to help extract more oil.
Industrial processes: CO2 can be used as a raw material in various industrial processes, such as the production of urea fertiliser, methanol, and other chemicals.
As fossil/conventional fuel consumption drops, the climate benefits of displacement will diminish, and CO2 will increasingly have to be obtained from biomass or through DAC (Smith Citation2016). These CO2 sources can help some CO2 consumption applications achieve a carbon-neutral life cycle, and Building materials, for example, may potentially yield negative emissions of carbon permanently stored. Negative emission opportunities, on the other hand, are likely to be limited and must be examined in the context of the complete product life cycle. Material differences determine how long carbon may be retained for CO2 use applications (D’Alessandro, Smit, and Long Citation2010; Keskin, van Heest, and Sholl Citation2010; Morris and Wheatley Citation2008). Carbon retention times range from less than a year for fuels to more than one hundred years for polymer, with retention in building/construction materials lasting millions of years or more. To achieve climatic targets, the extent and speed at which CO2 uses can be scaled up will be critical. In the next part carbon capture technologies, which we have discussed above are listed and discussed below. shows the processing conditions required for CCS technologies.
Table 1. CCS technology processing conditions (Lai, Ngu, and Hashim Citation2021).
In the processing conditions of the CCS technologies have been given such as processing temperature, processing pressure, and CO2 concentration.
2.1.1. Pre-combustion processes
Precombustion refers to a process in which fossil fuels such as coal, natural gas, or petroleum are converted into a mixture of CO and hydrogen (H2) before they are burned (combusted) for energy production. The purpose of precombustion is to capture the CO2 emissions produced during the combustion process, so that they can be stored or utilised in other applications instead of being released into the atmosphere. This is one of the methods used in Carbon Capture and Storage (CCS) technology. Before traditional fuels are burned, CO2 is absorbed. With gasification processes, synthesis gas is created by partially oxidising a fuel (such as coal) in steam and oxygen/air under high pressure and temperature (Breault Citation2010). The majority of syngas consists of hydrogen, carbon monoxide, carbon dioxide, and trace amounts of methane as well as other gaseous components. Water (H2O) and CO can be transformed to CO2 and hydrogen (H2), resulting in a gas mixture high in CO2 and H2O. This combination could contain between 15% and 50% CO2. The H2-rich fuel can subsequently be burned while the CO2 is sequestered in various ways. Compared to post-combustion technology, which removes diluted CO2 (5% to 15% CO2 level) from low-pressure flue gas flows, the shifted synthesis gas stream is rich in CO2. This facilitates the extraction of H2 prior to its combustion. Despite the higher initial capital costs of basic gasification compared to those of standard pulverised coal-fired power plants, pre-combustion CO2 collection is frequently more efficient due to its high concentration. Today’s commercially available systems for absorbing CO2 from an integrated gasification combined cycle (IGCC) power plant rely mostly on physical/chemical adsorption techniques and cost approximately $60/tonne. Hydrogen (H) is the primary raw material utilised in numerous industries, including the chemical industry (for ammonia and methanol), refineries, and iron/steel industries. Moreover, it is anticipated to play a significant role in the decarbonisation of the transportation sector (Zhang and Zhang Citation2021). Pre-combustion capture is the separation and collection of carbonaceous components prior to fuel combustion. This technology is predominantly employed by IGCC power plants. illustrates the capture process. These advantages reduce the extraction procedure’s energy consumption and equipment requirements by a substantial margin. Pre-combustion capture possesses a significant amount of potential in terms of efficiency and pollution control (Olabi et al. Citation2022). In the pre-combustion process, as depicted in , air is taken in directly and the separation procedure is initiated immediately. The separated Oxygen is then delivered to the gasifier, while the caught CO2 is used to produce H2 for the combustion turbine. In the case of the pre-combustion process the thermodynamic force is the driving force that triggers a specific chemical reaction or physical process to take place. It is the shift from equilibrium that propels the system to a new state. For instance, the thermodynamic force can be the pressure or concentration gradient that drives the conversion of reactants into desired products. Chemical potential is frequently associated with reactants and products in pre-combustion processes. The direction and extent of a chemical reaction is determined by the difference in chemical potential between reactants and products. The change in Gibbs energy between reactants and products determines the viability of a pre-combustion reaction. Understanding chemical equilibrium is essential for optimising reaction conditions and maximising the intended product yield in pre-combustion processes. These concepts are essential to the analysis and design of pre-combustion processes, including fuel reforming, gasification, and other chemical reactions involving in the processing of fuels.
Figure 2. Pre-combustion capture technologies (Zhang, Borhani, and El-Naas Citation2018).

2.1.2. Post-combustion processes
CO2 is captured from gaseous streams generated just after the burning of fossil fuels or other carbon sources in these processes. Because the CO2 concentration in the gaseous streams is lower than 15%, the force for CO2 removal from gaseous streams is lower in these approaches. In this class of procedures, a variety of solvents can be utilised. The most frequent carbon capture methods are post-combustion systems, which deal with the capture of large volumes of CO2 generated from various sources that include the burning of fossils fuel (Korre, Nie, and Durucan Citation2010). The main potential techniques for post-combustion CO2 capture are absorption, adsorption, membrane separation, and cryogenic fractionation. Chemical fluids, such as various forms and a blend of amine, as well as aqueous ammonia, have been getting attention as a viable commercial method and a fertile research ground. The advancement of solvent that can limit the energy intake of the capture operation is the main problem facing the chemical absorption strategy, which is largely based on the reversible and specific nature of the chemical reaction between the solvent and CO2 in the flue gas. The absorption capacity, reaction kinetics, degradation rate, and regeneration energy of the target solvents are frequently considered (Korre, Nie, and Durucan Citation2010). The contact technique is another crucial aspect that influences the absorption process’ efficacy. Furthermore, CO2 is removed from combustion exhaust gases in several post-combustion techniques. CO2 can be extracted using a liquid solvent or other separation techniques. In an absorption-based approach, CO2 is absorbed by the solvent and then released by heating to provide a high purity CO2 stream. This method of CO2 absorption is widely used in the food and beverage sectors. The post-combustion process described above is shown in . It can be seen in the figure below that the CO2 is captured while processing the air with the combustion of the fuel, and then processed with the NOx and SOx in a treatment unit. While in precombustion CO2 is captured before the combustion of the fuel.
Figure 3. Post-combustion CO2 capture (Lai, Ngu, and Hashim Citation2021).

In particular, case shown in , post-combustion has been carried out. Typically, the capturing plant is situated among the flue gas desulphurisation unit and the stack. In the post-combustion capture process, flue gases produced from the burning of fossil fuel comprise, 2–12% oxygen, 4–15% CO2 by volume with nitrogen (N2) and other minimal constituents (i.e. H2O vapours), carbon monoxide (CO), oxide of nitrogen (NOx), and sulphur oxides (SOx)) and are released at atmospheric pressure (1 bar) and temperature levels between 40 and 150 degrees Celsius (|Drage et al. Citation2012). The amount of energy contained in the oxygen present in flue gas depends on several factors, such as the fuel being burned, the combustion efficiency, and the amount of excess air used. When excess air is used in combustion processes, the flue gas typically contains between 2% and 12% oxygen, depending on the specific conditions of the process. To calculate the energy content of a flue gas, one would need to consider the fuel being burned and its heat of combustion, along with any other components present in the flue gas that may contribute to its energy content, such as carbon dioxide (CO2) or water vapour (H2O). The heat of combustion values is specific to different fuels and can vary significantly. The post-combustion method typically absorbs CO2 using wet or dry adsorbents and the principles of adsorption and desorption.
2.1.3. Oxyfuel combustion processes
Oxyfuel combustion is one of the key methods being explored for CO2 extraction from power plants utilising CCS. This is achieved by burning fuel with basically pure oxygen as opposed to air. CO2 and water vapour make up most of the combustion products produced by burning. The high content of CO2 in flue gases facilitates its absorption following the water vapour (Al-Janabi et al. Citation2015). Due to the absence of nitrogen, nitrogen oxide emissions are eliminated, unless there is an air leak or impurities in the fuel. In particular, the absence of nitrogen results in elevated CO2 levels in the flue gas. The absence of nitrogen in the oxidant combination leads to lower volumes in the combustion container and changed combustion parameters as compared to combustion using conventional air (Habib et al. Citation2011). Currently, cryogenic techniques are employed to produce oxygen from the air. These conventional procedures are very expensive in current terms. Numerous academics are conducting research on prospective new technologies that use membranes to isolate oxygen from the environment/air. The membranes can be fabricated from polymers/ceramics. While membrane-based oxygen enrichment technologies have been used for many years, adsorption-based technologies have gained popularity in recent times. Compared to membrane-based technologies, adsorption-based technologies offer several advantages. Firstly, they can achieve higher oxygen concentrations, up to 95% or more, which is not possible with membrane-based technologies. At higher nitrogen levels on the permeate side, however, polymer membranes produce a large oxygen flux across the membrane. In contrast, ceramic membranes can deliver pure oxygen content with a lower flux. In recent decades, numerous researchers have paid special attention to membrane production. In the case of ceramic membranes, the objective is to achieve high oxygen flow and stability, whereas the objective for polymer membranes is to achieve high oxygen purity (Sunarso et al. Citation2008). In most oxy-combustion experiments, ionic and electronic conducting ceramic membranes are now required. Recent research and development efforts have also centred on the manufacture of the ceramic membrane to produce syngas via the partial oxidation of hydrocarbons. The method of creating syngas via partial oxidation of methane (POM) has attracted considerable interest. The ceramics of the lanthanum cobaltite perovskite class have been intensively investigated as membrane materials. SrFeCo0.5Ox modified perovskite ceramics (Balachandran et al. Citation1997), Sr1.4La0.6GaFeO3 brown millerite-structured ceramic (Schwartz, White, and Sammels Citation2000), Sr0.2La0.8Fe0.69Co0.1Cr0.2Mg0.01O3 + 50Ag/50Pd ceramic – metal-based dual-phase membranes (Chen, Prasad, and Gottzmann Citation1999), and chemically stable yttria-stabilised zirconia-Pd thin dual phase membranes have been developed for membrane when these membranes are subjected to air on the feeding sides and a hydrocarbons fuels (like, methane) on the permeate end, their capacity to generate high-oxygen fluxes rises. Burning of fuels for power production typically comprises four basic components comprising a separation unit for air, a boiler for the combustion of fuel, a processing unit for flue gases, and a carbon dioxide processing unit.
In coal-fired power stations, the usage of oxyfuel combustion can be separated into two categories: (a) oxy-PC and (b) oxy-CFB. Alternatively, oxyfuel combustion for gas turbine-based power plants can be classified into two groups based on the fluid: (a) CO2-based GT cycle and (b) water/liquid-based GT cycles (Stanger et al. Citation2015). Further describes the oxy-fuel combustion process in a schematic way.
Figure 4. Oxy-fuel combustion (Zhang, Borhani, and El-Naas Citation2018).

2.1.4. Direct air carbon capture and storage (DACCS)
This is one of the most viable techniques that exist to date as it can capture carbon directly from the air. This method relies on a liquid solvent and solid sorbent to absorb the gaseous CO2 and produce an exhaust stream that is deficient in CO2 (Olajire Citation2010). The major role of this technique is to gather CO2 from the environment and generate highly concentrated CO2. After storage, the CO2 can be used in different applications, but the goal is suggested to be CO2 Storage. DAC not only contains one technology, but it also has various subcategories which are very promising and has the potential of achieving the goal of a sustainable environment. In general, two methods follow the DAC technique for carbon capture namely liquid solvent and solid sorbent but in addition there are more methods that are still in the developing phase such as Cryogenic DAC and humidity swing adsorption (Ghiat and Al-Ansari Citation2021).
Cryogenic DAC utilises the sublimation point of CO2 to make solid CO2 from the air, which can be kept as solid CO2 or re-sublimated to generate highly gaseous CO2 (sublimation here refers to the phase change of the CO2 from solid to gas and vice-versa). Using anionic exchange resins, swing adsorption is a method for absorbing and releasing CO2 in the presence of moisture. While these sorbents might reduce energy consumption in dry climates, they can also increase water use because of their ability to hold CO2 in the absence of water. To reduce the requirement for heat, Voskian and Hatton propose an electro-swing mechanism in which CO2 adheres to Poly anthraquinone-carbon nanotube composite while charging, then releases it when discharging, resulting in high-quality CO2 flow (Voskian and Hatton Citation2019). Caustic calcined magnesia (MgO) has been used to recover CO2 from the air and an aqueous amino acid solution has been used to absorb CO2, which has been formed through the crystallisation of an insoluble carbonate salt in the presence of the guanidine molecule. Solid sorbents and liquid solvents are two of the most advanced approaches to DAC development, and this analysis provides a unique opportunity to analyse their present state of research and their scalability prospects. depicts the graphical representation of the DAC technology in which the process of carbon capture from the air has been demonstrated (McQueen et al. Citation2021).
Figure 5. DAC approach (McQueen et al. Citation2021).

CO2 molecules interact with hierarchically porous materials in the solid sorbent technique, which can remove CO2 from the inlet gas mixture. In addition, Sorbed CO2 from gas mixtures can be removed via physisorption or chemisorption, which are two different types of adsorptions. The heat of adsorption can be thought of as a measure of how tightly a CO2 molecule is bound to the sorbent’s surface. Physisorption happens when the heat of adsorption is less than or equal to 15 kcal mol−1, whereas chemisorption occurs when the heat of adsorption is more than or equal to 15 kcal mol−1 (Wilcox Citation2012). Zeolite-based chemisorption techniques are an exception to this rule. Zeolite-based chemisorption is an exception to the thermodynamic limits of efficiency because it is a non-equilibrium process. In traditional thermodynamic processes, the system is in equilibrium, meaning that there is no net flow of energy or matter. However, in chemisorption, the adsorbent (zeolite) and the adsorbate (gas) are not in equilibrium, as the adsorbate molecules are held in place on the surface of the adsorbent by chemical bonds. This allows for the separation of gases or other molecules to occur at lower energy levels, which is not possible in traditional thermodynamic processes. Additionally, the pore size of zeolites can be manipulated to selectively adsorb certain molecules, which further improves the efficiency of the process. Overall, zeolite-based chemisorption can overcome the thermodynamic limits of efficiency by using non-equilibrium processes and selective adsorption.
The amine surface functionalization of solid sorbents can boost their ability to interact with CO2 molecules, making them more CO2-selective. Surface functionalization refers to the process of modifying the surface properties of a material by introducing functional groups or molecules onto the surface.
Some of the materials being studied right now include activated carbon, zeolites, metal-organic frameworks (MOFs), carbon nanotubes, and silica materials as well as porous organic polymers and molecular sieved carbon (Zhou, Yu, and Ma Citation2021). In solvent-based approaches, structured packing is frequently employed to increase the surface area across both the gas and liquid phases. In the case of solvent DAC with structured packing, the surface area is increased while gas-side pressure drops are frequently minimised. To absorb CO2, a strong basic hydroxide solution is needed in the solvent-based technique. An anionic exchange follows, and the calcium carbonate precipitate pellets are the end result. To collect CO2 from precipitated calcium carbonate, the solvent method requires high temperatures. Having a powerful capture agent and a high amount of regeneration energy is not unique to liquid solvent DAC. Although CO2 is extremely diluted in the air, it still requires a strong base to separate it from the rest of the air, which adds to the energy needed for this separation. Because we are storing CO2 in this manner, direct air capture (DAC) is considered the most efficient method (Fujikawa, Selyanchyn, and Kunitake Citation2021).
3. Factors affecting the carbon capture technologies
It has been seen that many factors affect the carbon capture process and the carbon capture technologies. There are various substances and materials such as NaX@NaA and MOF-177-TEPA that exhibits some of the properties. The presence of these substances affects the amount of CO being captured. In the next section, various aspects of the adsorbents have been discussed.
3.1. Adsorbents in the carbon capture technologies and their performance
To avoid the need for amine scrubbing, solid adsorbent adsorption of CO2 in a gas mixture has been researched as an attractive and promising alternative to the PSA, TSA, and TVSA approaches. Amine scrubbing should be avoided in CO2 capture and separation processes because amines can react with the adsorbent material, causing deactivation or degradation of the adsorbent. This reaction can reduce the efficiency of the CO2 capture process and negatively impact the overall performance of the system. Amine scrubbing is a common method used in various industries to remove carbon dioxide (CO) from industrial gas streams. However, there are some concerns about the use of amine scrubbing, which suggests that it should be avoided in certain situations. One of the primary concerns is the high cost associated with amine scrubbing. This process requires a significant amount of energy and resources, making it an expensive solution for reducing CO2 emissions. Additionally, the high cost of amine scrubbing can make it less economically viable compared to other carbon capture technologies. Another issue is related to the environmental impact of using amines in scrubbing. Amines are known to be toxic and can pose a risk to both human health and the environment. Moreover, the production of amines requires a significant amount of energy and can lead to the emission of harmful pollutants.
There are also concerns about the potential negative impact of amine scrubbing on the quality of the gas stream. The scrubbing process can result in the removal of other gases, such as methane, which can have negative effects on the overall efficiency of the system. Additionally, the removal of methane can also contribute to the release of other greenhouse gases into the atmosphere. One example of a commercial plant using PSA for CO2 separation is the in Linde engineering (The Linde Group). This project uses PSA technology to remove CO2 from natural gas, producing a sales gas that meets the specifications for transportation through pipelines. In this case after the removal of the CO2, the final destination of the CO2 depends on the various factors mainly the project objectives. Some of the possible usages are storage or using the captured carbon for the variety of the industrial applications. One such technique id EOR, in this technique the CO2 is injected into the oil reservoir to boost the oil production. In addition, it can also be used in the manufacture of compounds, the carbonation of beverages, and other industrial processes. It is essential to observe that the precise destination of the captured CO2 will depend on economic viability, environmental factors, and the project’s objectives. Diverse initiatives may employ diverse strategies for CO2 management and utilisation.
Another example is the Clean Energy Centre in North Dakota, which uses Temperature Swing Adsorption (TSA) technology to upgrade biogas (Benson et al. Citation2014). Biogas is treated to remove impurities, including CO2, and the purified gas is then used as a renewable fuel source.
In addition, PSA: pressure swing adsorption Dry adsorbents are typically placed in a packed column with a CO2 stream, and the CO2 is drawn onto the adsorbent surface at 25–65°C ambient pressure and temperature, respectively, to begin the adsorption process. CO2 adsorption and desorption using PSA, TSA or a mix of both are cost-effective when producing pure CO2. It was the reversible reaction and the enhanced adsorption effectiveness achieved by the chemical functionalization of adsorbent surfaces that brought attention to the importance of adsorption in CO2 collection. As a result of the alarming rise in CO2 levels, from 290.70 ppm in the preindustrial era about 1880 to 409.80 in 2019, adsorption has been used as a means of reducing CO2 emissions, which in turn has led to the rise in global warming and climate change. Post-combustion and DAC carbon capture can benefit from the use of a variety of standard and developing solid adsorbents. At various CO2 capture situations including pre-combustion, post-combustion, and DAC, this review seeks to discover adsorbents with the highest CO2 adsorption performance. To better understand CO2 adsorbents, this article explains the physical and chemical properties of the various adsorbents and their synthesis processes. At 5.60 mmol g1, NaX@NaA core-shell microspheres have the maximum CO2 adsorption capacity measured under DAC circumstances, according to the study’s findings. The maximum post-combustion CO2 adsorption capacity was found for MOF-177-TEPA at 4.60 mmol g-1 due to the low CO2 diffusion resistance and easy access to active sites provided by the tetraethylenepentamine characteristics. The adsorption capacity of these adsorbents ranged from 0.0000026–48.71 mmol-1 at 1 bar for oxy-combustion processes. To use these adsorbents in the correct adsorption circumstances, their features must be understood and evaluated. Because of the poor durability, low productivity, and instability for prolonged cyclic adsorption-desorption, and expensive adsorption arrangement, as well as their elevated gas flow rate, longer flow switching time, high adsorption accommodation requirement, and a low tolerance for water and impurities in flue gases, this technology cannot be used for pilot-scale CO2 capture. To support pilot-scale carbon capture, the research proposes future upgrades. These helps offer information on how to capture CO2 from industrial exhaust gases (Colangelo, Petrillo, and Farina Citation2021).
To extract CO2 from various gas streams, the adsorption of CO2 is used. The literature reports on a wide range of adsorbents, including physical adsorbents such as silica materials, zeolites, activated carbons, MOFs, COFs, ordered porous carbons, alkali metal/metal oxide-based substances, ACFs, graphene, and CMSs, as well as composite adsorbents made by incorporating different adsorbents, and chemical adsorbents such as composite.
3.2. Costs related to carbon capturing
As we have seen before in carbon capture technologies the cost always plays a crucial role. Many factors affect the cost associated with carbon capture technologies. This section will shed a light on the cost factors. The cost estimation is provided for the pre, post, and oxyfuel combustion. For cost estimates, it is critical to distinguish between the cost per unit mass CO2 captured and removed.
Energy penalties for separating and compressing CO2, which reduces the efficiency of power generation, and cost penalties, which are the costs of building, installing, and operating the facility, make up the cost of carbon capture in electricity-generating power plants. Furthermore, there are the costs related such as captured costs and avoided costs.
In addition, in electricity generation power plants the term LCOE (Levelized cost of electricity) is used most often. The LCOE is the present value of electrical output throughout the lifespan of a power station. It is the estimated profitability necessary to construct and run an electricity production plant during a specific cost recovery time and is commonly represented in $/MWh. The same approach applies to the Levelized cost of production of materials, with cement, steel, etc. replacing the amount of electricity as the product.
(1) captured cost, which represents the rise in LCOE per unit CO2 captured and is roughly comparable to the breakeven CO2 sales price; and (2) avoided cost, which represents the rise in LCOE per unit net decline in CO2 emission severity of the plant and is comparable to the breakeven CO2 emissions penalty. These quantities are calculated as:
Captured cost [$/tCO2]-
Avoided cost [$/tCO2]-
In such equations, LCOE is in $/MWh, which consists of the capital and operating expenses for energy production and CCS. The benchmark scenario is the plant without carbon capture as the reference. The subscripts w/CCS and w/o CCS for LCOE refer, correspondingly, to facilities with and without carbon capture. CO2 captured represents the quantity of CO2 captured per megawatt-hour of electricity generated. ‘Net CO2 emissions’ consists of the plant’s emissions for power generation and CO2 capture excluding the quantity captured. The costs and emissions related to compression, transport, and storage may be included in the ‘LCOE with CCS’ and ‘Net Emissions with CCS’ terms, based on the underlying parameters. It also demonstrates the cost savings through learning for each technology, from a first-of-its-kind (FOAK) to an Nth-of-its-kind (Irlam Citation2017) (NOAK) plant (Kazemifar Citation2022). In conclusion, the rise in LCOE with CCS in a FOAK plant ranges from 45 to 70 % compared to the baseline situation without CCS. The costs associated with carbon capture for various power plant systems are summarised in . The table describes the cost of carbon capture and associated costs to it such as avoided cost.
Table 2. Cost estimate in power plans (Kazemifar Citation2022).
Another key consideration is that carbon sequestration systems may lack their objectives after a period of operation. In identical circumstances, a plant in Texas was shut down following three years of operation because it fell 17% short of its goal (Groom Citation2020). Due to the rarity of oxyfuel carbon, the captured costs for oxyfuel plants are $36–$67/tCO2 and the avoided cost is $45–$73/tCO2. Post-combustion capture from pulverised coal plants has the least cost, while pre-combustion capture from integrated gasification combined cycles facilities has the largest cost.
4. Use of captured carbon
In this section, we have discussed various ways and the components in which the captured carbon can be converted. Some of them are converting the captured carbon again in the form of gas which is CO and O2 (Jerndal, Mattisson, and Lyngfelt Citation2006). Methanol, methane, CO, fuels, bicarbonate, carbohydrates, polymers, formic acids, and other valuable compounds have all shown that CO2 may be used in a variety of techniques (see ).
Figure 6. Classification of CO2 use ((IEA), Citation2019a).

The shows the Graphical representation of techniques for Methanol, methane, CO, fuels, bicarbonate, carbohydrates, polymers, formic acids, and other valuable compounds that CO2 may be used. Further, the figure explains the use of carbon dioxide in various processes hence, but the potential processes have not been explained as the manuscript is strictly focused on carbon capture technologies. But in decaffeination, carbon dioxide (CO2) is often used as a solvent to extract caffeine from coffee beans. After the caffeine has been removed, the CO2 is typically recycled and reused in subsequent decaffeination processes. Understanding carbon emissions is more important than understanding carbon breakdown. Because carbon emissions differ based on the source and the magnitude of the emission source.
Depending on the application, carbon can be employed directly or indirectly. The conversion of captured carbon with the use of CCS technologies, which are listed in the following sections, is needed to facilitate the non-direct use of carbon IEA (Citation2019a).
CO2 incorporation into products or services does not always imply a reduction in emissions. Quantifying the potential climatic benefits is difficult and time-consuming, and it necessitates a life-cycle methodology. Using CO2 has a positive impact on the environment because it allows us to replace high-emission products and services, such as coal and gas, chemicals, and aged building resources, with low-emission alternatives (IEA Citation2019a.
4.1. Decomposition of carbon into CO and O2
The decomposition of carbon into carbon monoxide (CO) and oxygen (O2) is a chemical reaction that occurs when carbon is burned in a limited supply of air. When there is not enough oxygen available to completely burn the carbon, some of it will react to form CO instead of CO2. This can result in the production of poisonous and flammable CO gas, which is why it is important to ensure that fuels are burned in a well-ventilated area with a sufficient supply of air.
Many investigations into the breakdown of CO2 have been conducted till date.
In one research, dielectric-barrier discharge (DBD) reactors packed with BaTiO3 balls, glass beads of various sizes, and a mixture of a Ni/SiO2 catalyst and BaTiO3 balls were used to explore CO2 breakdown to CO and O2 at lower temperatures and ambient pressure (Zhang et al. Citation2017). The reaction was greatly influenced by the packing beads’ properties as well as the reactor’s design. XRD, BET and TEM were used to analyse the Ni/SiO2 catalyst samples. Using a Ni/SiO2 catalyst in conjunction with a DBD plasma appears to improve CO2 breakdown, and a plasma-assisted CO2 dissociation reaction mechanism has been postulated. Comparing the results of the BaTiO3 bead-packed stainless-steel mesh with a glass ball of 3 mm, the BaTiO3 bead-packed mesh with a Ni/SiO2 catalyst significantly improved CO2 conversion as well as the energy efficiency by a factor of 14.8, and that with a Ni/SiO2 catalyst by 11.5 in a DBD plasma at low temperatures (115 °C) (Akay Citation2020). In the description of CO2 decomposition has been shown. The coupling of a DBD plasma with a Ni/SiO2 catalyst has been shown to enhance the decomposition of CO2, and a reaction mechanism explaining the plasma-aided CO2 dissociation over the catalyst has been established.
Figure 7. CO2 decomposition into CO and O2 (Zhang et al. Citation2017).

4.2. CO2 derived fuels
Methane, methanol, gasoline, and aviation fuel are just a few of the fuels that can be made from the carbon in CO2. The CO2 is combined with hydrogen to form a carbon-containing fuel that is easier to handle and use than pure hydrogen. Hydrogen generation using water electrolysis has gained considerable interest as a method for reducing greenhouse gas emissions by utilising low-carbon sources such as renewable energy (Guilbert and Vitale Citation2021). Aircraft, for example, is an ideal use for CO2-derived fuels due to the difficulty of utilising other low-carbon energy carriers, such as electricity or hydrogen. CO2 and hydrogen can be used to produce methane and methanol in pilot and demonstration facilities, each utilising hundreds to thousands of tonnes per year. In , the method of chemical conversion of CO2 into other chemical products can be seen through the hydrogenation and syngas methods.
Figure 8. Conversion of CO2 into chemical intermediates ((IEA), Citation2019a).

Other chemical and biological methods for producing CO2 fuels are in the early phases of research or demonstration. A large amount of energy is required to convert CO2 into various fuels and chemical intermediates IEA (Citation2019a). It can be seen from that CO2 can also be converted into fuel by chemical conversion. Further chemical conversion is also divided into two parts which are direct chemical conversion and indirect chemical conversion. In direct conversion only the hydrogenation process in used. But in indirect use of the CO2 the syngas and reverse water gas shift have been used. Both methods are used to provide the fuels after processing the captured carbon (Acosta-Ramirez et al. Citation2012). The expenditure of producing methanol and methane by CO2 is currently 2 to 7 times greater than the expense of producing it from energy sources in the majority of the world’s locations. Carbon dioxide-derived methanol and methane can only compete if power costs are kept low enough to account for between 40 and 70 percent of the total production costs. Still to date, the most common and cost-effective is the use of electricity and low-carbon hydrogen (Liguori et al. Citation2020). In North Africa, Chile, and Iceland CO2 and low-cost renewable energy are available for the production of methane and methanol at commercial levels. The George Olah facility in Iceland, which uses hydrogen generated from renewable electricity to convert roughly 5600 tonnes of CO2 into methanol every year, is an excellent example. Over time, manufacturing costs for CO2-derived fuels are predicted to decline as capital expenditures are reduced and low-cost renewable energy is made available for feedstock CO2 generation. Methanol produced from carbon dioxide may become competitive in more places throughout the world depending on local market prices for methanol. As long as there is no strong (robust) CO2 price system, liquid fuels like diesel and aviation fuel generated from CO2 will remain uncompetitive. If a robust CO2 pricing mechanism were in place, the cost of emitting CO2 would grow, making it more expensive to generate traditional fuels that emit CO2. This would subsequently increase the market competitiveness of fuels made from CO2. A robust (strong) CO2 pricing system would increase the price of conventional liquid fuels, making CO2-based fuels a more appealing and competitive alternative.
CO2 methane and methanol can both help the climate, but only if they are produced using low-carbon energy. Emissions can be decreased by up to 93% for methanol and up to 74% for methane in the best-case scenario, based on a review of the relevant literature. To be accepted by current product quality standards, these products will need to undergo significant testing.
In addition, there are some economic aspects of CO2 derived fuels. The economic visibility of carbon dioxide denied fuels although is a complex topic which involves various factors including development in technology, policies arranged by governments and also the demand. One of the major challenges for these fuels is the cost of production the process of converting carbon dioxide into fuel needs energy and the current technologies used for this purpose is all processes are still relatively expensive compared to the traditional fossil fuels. So the economic feasibility depends on the amount of research and development carried out currently and in the future.
As previously mentioned, fossil fuels are one of the most significant sources of carbon dioxide. However, the proposed solution is not sustainable. Other sustainable alternatives include non-fossil fuel resources, DAC, biogenic sources, carbon neutrality, and the circular economy. However, additional discussion has been introduced regarding the non-fossil fuel sources of carbon dioxide. If the CO2 used originates from non-fossil fuel sources, the potential sustainability of CO2-based fuels is enhanced. Direct air capture, where CO2 is captured directly from the atmosphere, and biogenic sources, such as CO2 emitted from biological processes or waste streams, are non-fossil fuel sources of CO2. DAC was also discussed in depth in the preceding section. Additionally, CO2 from biogenic sources, such as refuse biomass or agricultural processes, can be regarded as a sustainable source. Biogenic CO2 is a component of the natural carbon cycle and does not contribute to the overall increase in atmospheric CO2. Utilising CO2 from these sources for CO2-based fuels can be advantageous to the environment, as it reduces waste emissions and provides an alternative to carbon derived from fossil fuels.
Another consideration is whether the CO2 used in CO2-based fuels is consistent with carbon neutrality and the circular economy. Carbon neutrality implies that the emissions released during the production and use of CO2-based fuels are matched by the capture or removal of an equal quantity of CO2 from the atmosphere. The circular economy concept prioritises minimising waste and maximising resource efficiency, including the use of CO2 as a valuable basic material rather than as a pollutant. The provenance of CO2 as a raw material for CO2-based fuels can contributes to a more sustainable and low-carbon future by promoting non-fossil fuel sources of CO2, such as direct air capture and biogenic sources, and assuring adherence to carbon neutrality and circular economy principles.
4.3. CO2 derived polymers
As an alternative to fossil fuels, CO2 can be utilised in the synthesis of compounds like synthetic rubber and polymers. The most technologically advanced process for converting CO2 to methane and methanol is the same as with fuels obtained from CO2. Once transformed into olefins and aromatics, methanol can be employed in a wide variety of industries, including plastics manufacturing, food processing, and healthcare.
In the manufacturing of plastics, foams, and resins, a polymer is employed. As part of the production process, CO2 can be substituted for fossil fuel-based raw materials. A lower-energy molecule is formed throughout the polymerisation, the opposite of the transformation of CO2 into fuel and chemical intermediates (carbonate). Polymer facilities that use CO2 as a raw material are now being operated by several companies (Zhu Citation2019).
A wide range of products can benefit from the use of polymers made from CO2. It can be seen from that chemical conversion of the captured carbon is possible through co-polymerisation. In these methods, the captured carbon needs to be added with heat, energy, and fossil-based raw materials. shows the chemical conversion of CO2 into plastics and resin through the co-polymerisation process.
Figure 9. CO2 derived polymers ((IEA), Citation2019a).

Because of their high market value and low energy requirements, CO2 polymer manufacturing can compete in the market. If CO2 is less expensive than the fossil fuel-based raw material it replaces, polymers can be produced for 15 to 30% less than their fossil counterparts. It has been more than a decade since a joint venture between Japanese chemicals giant Asahi Kasei Chemicals and Taiwanese conglomerate Chi Mei Corp has been producing roughly 150,000 tons of polycarbonates per year utilising CO2 as a raw source. Polymer processing with CO2 may be possible in regions where existing polymer facilities can be upgraded and fossil fuel prices are high, notwithstanding the small potential market for polymers.
As much as half of the polymer’s mass can be absorbed by CO2 in its synthesis. Life-cycle CO2 emissions reductions of 15% can be achieved with a polymer that contains 20% carbon dioxide by weight. A similar testing process is required for polymers containing large mass percentages of CO2 before they can be put on the market (Mohanty, Misra, and Hinrichsen Citation2000).
4.4. Captured carbon for use in building materials
CO2 can be used as a raw material in the production of building materials such as cement and aggregates, or it can be used as a substitute for water in the curing process of concrete. These applications use CO2 and calcium or magnesium to produce low-energy carbonate molecules, which are the building blocks of concrete. One of the most promising CO2 uses is CO2-cured concrete, whereas the integration of CO2 into the cement manufacturing process is still in its infancy. shows CO2-derived building/construction materials IEA (Citation2019a).
Figure 10. CO2-derived building/construction materials ((IEA), Citation2019a).

When compared to conventionally manufactured concrete, CO2-cured concrete has better performance, reduced manufacturing costs, and a smaller CO2 footprint than the latter. The reduction in cement consumption, which accounts for the majority of concrete’s costs and emissions during its entire lifespan, is primarily responsible for the climate advantages. Carbon Cure and Solidia Technologies, both based in the United States, are pioneers in the development and commercialisation of CO2 curing technology.
It’s still impossible to estimate how much CO2-cured concrete can cut emissions. Concrete’s carbon footprint can be decreased by as much as 80%, according to Carbon Cure, however, this claim has not been independently validated. For early adoption of these technologies, pre-cast concrete products and ready-mixed concrete that is cured with CO2 and water at the plant before being transported for use in the building are very promising.
Existing legislation and product standards may impede early adoption in some markets. Existing product standards can take a decade or more to update, and it is necessary to conduct multi-year experiments to prove that the products are both safe and ecologically benign. New CO2-derived building materials could benefit from a shift away from prescriptive regulations and towards performance-based ones. For the time being, these novel materials could be used in non-structural concrete applications that do not require significant mechanical strength (such as the construction of floors, roads, and ditches) (Mas et al. Citation2012). Further, the use of CO2 as a building material is a new concept, and there is little evidence to support a quantitative assessment of its practicality. However, some considerations that may influence the viability of utilising CO2 as a building material include the cost of capturing and purifying the CO2, the energy necessary to change it into a solid material, and the qualities of the final material, such as strength and durability. Furthermore, the availability and cost of other building materials, as well as the demand for sustainable construction methods, may all have a part in deciding the viability of employing CO2 as a building material. To completely assess the practicality of this notion, more research and development, as well as real-world testing, would be required.
4.5. COa use in agriculture
The use of CO2 in biological processes like algae production and greenhouse crop cultivation can boost yields. By far the most well-proven method of increasing crop yields, CO2 with low-temperature heat is used in industrial greenhouses, where it can improve output by 25% to 30%. With an estimated annual consumption of between 5 and 6.3 MtCO2, the Netherlands is the clear leader in greenhouse CO2 use. For example, industrial plants account for about 500 kilograms per year of CO2 emissions; on-site gas-fired boilers and cogeneration systems account for the rest. The use of other industrial CO2 sources or atmospheric CO2 taken directly from the atmosphere in place of these on-site systems could have a positive impact on the climate. Products and services with smaller carbon footprints and emissions reductions could benefit from CO2 utilisation. CCS can also be used in conjunction with it since the IEA has continually emphasised that CCS is an essential element of the portfolio of technologies required to meet climate targets. Particularly when it comes to investments in CO2 capture potential, technical improvement and (in rare situations) early development of CO2 transport infrastructure, the usage of CO2 can be beneficial.
However, to achieve the large reductions in emissions required to satisfy the Paris Agreement goals, CO2 usage cannot replace CO2 storage. Many CO2 use prospects are projected to be on a smaller scale, with very little potential for negative emissions, since technology and the market are still developing.
CO2 use could be a more attractive mitigation option if storage capacity for CO2 is restricted, according to an IEA scenario analysis, but it will not scale to the same degree as other methods used to deploy CO2. Clean Technology Scenario (CTS) estimated that, according to the Paris Climate Agreement, worldwide CO2 emissions from industry and fuel transformation will reach 250 million metric tonnes annually by 2060. Carbon dioxide (CO2) demand would grow three-fold in 2060 in a CTS scenario where the cumulative CO2 storage capacity is limited to just 10 GtCO2, the Limited CO2 Storage (LCS) scenario variant (). CO2 is converted into methanol, urea, and CO2-derived fuels by the combustion process (kerosene, gasoline, and diesel). CO2 storage and usage are expected to differ in magnitude, even though this scenario study solely covers the use of CO2 in energy and industrial applications (Paustian et al. Citation1998).
5. New opportunities and emerging markets in the area of captured CO2
In the area of carbon capture, it has been seen that there are various opportunities and those opportunities have attracted nearly everyone interested in climate change, technology, and circular economy including researchers, industry, investors, and governments. Further, this section deals with the emerging market in carbon capture in different categories such as chemicals, building materials from waste and minerals, and the use of CO2 in agriculture areas. A total of 10 MtCO2/yr of CO2 demand for the food industry and beverages could be met by each of the five key categories (fuels, chemicals, building materials from minerals, building materials from waste, and CO2 use to enhance the yields of biological processes), but commercial and legal constraints prevent this from happening. When an application is scalable and uses low-carbon energy and replaces a product with greater life-cycle emissions, the usage of CO2 can help achieve climate goals. Permanent carbon retention is a feature of some CO2-derived products, particularly building materials. There is a need for improved methodologies and a better understanding of the long-term climate advantages of CO2 use applications. CO2 utilisation is projected to remain limited in the short term, although early opportunities could be explored, particularly in the building materials sector. The early creation of a market for CO2-derived products and the advancement of technological standards can both be aided by the public purchase of low-carbon items. If a net-zero CO2 emission economy is to be achieved, CO2 extracted from biomass or the air might play a vital role, notably as a carbon source for aviation fuels and chemicals. Products requiring carbon could benefit from CO2 as a raw material. Carbon is necessary for the structure and properties of some molecules, and carbon-based fuels may be required even if electricity or hydrogen can’t be used directly (for example, in aviation).
While carbon is essential for the structure and properties of some molecules, it also plays an important role in many industrial processes and applications, including aviation. As we work to transition to cleaner energy sources, it may not be possible to completely eliminate the use of carbon-based fuels in certain applications. It has been stated that carbon dioxide will increasingly have to come from biomass or atmosphere to reach a net zero carbon dioxide economy. It has not been stated that it has only to come from biomass. It has been made to clarify the hypothesis which states that to achieve net zero carbon dioxide economy the carbon dioxide should come from biomass or atmosphere.
Carbon dioxide will increasingly have to come from biomass or the atmosphere to reach a net-zero CO2 economy. Approximately 230 million tons (Mt) of CO2 are emitted into the atmosphere every year. 130 million tonnes of CO2 are utilised in urea production, followed by oil and gas with 70 to 80 million tonnes of CO2 for increased oil recovery. Commercial uses include food and beverage processing; metal fabrication; cooling; fire suppression; and encouraging plant growth in greenhouses. CO2 is the primary source of energy for most commercial uses today.
CO2 may now be converted into new fuels, chemicals, and building materials using innovative methods. Governments, businesses, and investors are becoming increasingly interested in these chemical and biological conversion processes, although many are still in the early stages of development and face commercial and legal obstacles. Production of CO2-based fuels and chemicals consumes energy and hydrogen. The amount of energy required to manufacture hydrogen by combining it with CO2 depends on several factors, including the production method, the purity of the hydrogen produced, and the scale of the operation. In general, the production of hydrogen from CO2 can be an energy-intensive process, requiring more energy than is produced by the resulting hydrogen. The exact definition of ‘a lot’ is subjective and dependent on specific circumstances. For example, estimated production costs of methanol and methane from CO2 in most regions are 2 to 7 times higher than for their fossil counterparts. The major cost factor is typically electricity, accounting for between 40–70% of the production costs, and hence exceptionally low average grid electricity prices are required for CO2-derived methanol and methane to be competitive. Even under these conditions, the direct use of low-carbon hydrogen and electricity as a fuel will be a more cost-effective option in most cases.
Fuels like aviation jet fuel can be made easier to handle and utilise thanks to the carbon in CO2. Chemicals and polymers can use CO2 as a raw material in place of fossil fuels. Reacting CO2 with minerals or waste streams, such as iron slag, to create carbonates for building materials is a less energy-intensive option (Quadrelli et al. Citation2011). It is impossible to predict the future market demand for CO2 products and services. Due to its infancy and dependency on governmental frameworks, it is extremely difficult to predict the market for new technologies. However, in most cases, the expense of using low-carbon hydrogen or electricity would be more cost-effective than using CO2 for fuels and chemicals in large quantities because of their lower carbon footprints. Commercial and regulatory issues are the main impediments to CO2 utilisation in the foreseeable future, rather than technological ones. Each of the five CO2-derived product and service categories (1. fuels, 2. chemicals, 3. building materials derived from minerals, 4. building materials derived from waste, and 5. CO2 use to promote plant growth) are examined for the near-term potential to increase the market to at least 10 Mt CO2 use annually. For food and beverages alone, this level of CO2 consumption is nearly as high as the present demand for CO2. The cost of producing CO2-based fuels and chemicals is now several times greater than the cost of producing their conventional counterparts. One of the primary reasons for this is the high expense of producing hydrogen. In places where both inexpensive renewable energy and CO2 are available, such as Chile or Iceland, commercial production is viable. Polymers derived from CO2 could be cheaper to make than those obtained from fossil fuels, but the market for these materials is still modest. When compared to traditional concrete, the prices and performance of CO2-cured concrete are significantly better than those of conventionally manufactured concrete. Using trash and CO2 to produce construction materials can also be cost-effective because it eliminates the expense of disposing of waste in a conventional manner. In the case of CO2-cured concrete, the CO2 is permanently stored in the product, with extra climate benefits arising from the lower cement intake. It may be necessary for some concrete goods to conduct trial runs and update product specifications before they can be widely deployed (Mülhaupt Citation2013). A life-cycle evaluation and a grasp of market dynamics are necessary to accurately determine the climate benefits of CO2 consumption. To achieve climate benefits, carbon dioxide utilisation must be scalable, use low-carbon energy, and replace an alternative product with larger emissions. In the long run, to achieve climatic benefits, a net-zero CO2 emission energy system would require CO2 to be obtained from biomass or air. Building materials, for example, can reduce CO2 emissions more than things that emit CO2 into the atmosphere, such as fuels and chemicals, because of their ability to store carbon.
6. Application potential and opportunities
There are different application potentials and opportunities such as power generation, industrial processes, bioenergy and biomass, and research and development but at the same time immense research is needed in this area. Robust life cycle evaluations based on the data set and explicit methodological principles are required to create future policies and investment decisions. Businesses, governments, and research institutions are actively investigating these development and implementation opportunities for CCSU technologies. Enhanced policy frameworks, financial incentives, and international partnerships are accelerating the application of CCSU for a clean and sustainable environment.
In Application potential and future opportunities in CO2 capture have been discussed.
Table 3. Application potential and future opportunities in CO2 capture.
Negative emissions from the utilisation of CO2 are also extremely unlikely. To compete with conventional processes, many CO2 usage methods must be recognised in climate policy frameworks or where lower-carbon products are offered as incentives for consumers.
Negative emissions from the use of CO2 are also highly unlikely. This means that it is highly unlikely that we will be able to generate substantial negative emissions by utilising CO2 through different methods, such as CCS or DAC. Negative emissions refer to the removal of carbon dioxide from the atmosphere, which is essential for mitigating the effects of climate change. Utilisation of CO2 aims to capture CO2 emissions produced by industries and power plants and store or use them in some manner, such as injecting them underground for permanent storage, utilising them for enhanced oil recovery, or producing fuels. CCS and DAC technologies exist and have been implemented on a limited scale, but they face significant obstacles, such as expensive costs, technical impracticability, and environmental concerns. Even if these technologies become more cost-effective and scalable in the future, it is possible that they will not be able to deliver the required negative emissions at the required scale and in a timely manner to meet the climate objectives. Therefore, relying solely on negative emissions from CO2 utilisation as a solution to climate change is not a viable option, and it is necessary to reduce greenhouse gas emissions at their sources.
For items created from CO2 that have been shown to have a positive impact on climate change, the use of public procurement can be a successful method. Initially, the CO2 consumption market is likely to be small, although early opportunities do exist. Some of the opportunities are listed below,
CCS systems could absorb CO2 emissions from industrial operations, power stations, and other sources and either store them underground or utilise them in industrial processes. There is opportunity for businesses to develop and market CCS technology to aid in emission reduction.
Carbon offsetting is investing in initiatives that decrease or eliminate CO2 emissions to offset emissions produced by a person or business. This market is already well-established and expanding, with firms and people eager to pay for carbon offsets.
The move to renewable energy sources including wind, solar, and hydropower can aid in reducing CO2 emissions. As demand for clean energy rises, companies involved in the development and manufacture of renewable energy technology may discover possibilities to offer their products and services.
Technology to use CO2 as a raw material for goods such as polymers, fuels, and chemicals are being produced. Businesses capable of developing and commercialising these technologies may discover possibilities in the market for CO2 consumption.
Governments and organisations are establishing carbon pricing mechanisms, such as carbon taxes and cap-and-trade systems, to encourage businesses to decrease carbon emissions. On the market for CO2 consumption, there may be chances for firms that can assist other companies in complying with these rules.
This includes building materials, but also polymers, and industrial carbon dioxide utilisation in greenhouses, which are some of the early potentials. Early implementation prospects can be found in industrial locations that have low-carbon energy, low-cost raw materials, and customers all in close proximity. These include aviation fuels and chemicals produced from biogenic or atmospheric CO2 that can help achieve a net-zero emission economy in the future. R&D for hydrogen production containing low carbon should be done at the same time as this. This broad spectrum of potential CO2 applications includes both direct consumption and the transformation of CO2 (via a variety of processes) into chemicals, fuels, and construction materials and products (conversion).
Conversion paths, despite their energy-intensive nature, are receiving increasing attention and support from industry, governments, and investors. Some companies have recently invested in companies like Solidia and CarbonCure. Both of which use carbon dioxide to produce concrete. With a USD 20 million global competition, the NRG COSIA Carbon XPrize supports the development of new CO2 use prospects in North America. Canada, Japan, and the United Kingdom are among those countries that have invested much in research and development for CO2 utilisation. Several considerations are motivating a growing amount of attention to be paid to CO2 utilisation options. Its ability to aid in the achievement of climate change mitigation objectives is one of its most important features. Among the other motivations is technological leadership, the projected availability of low-priced and ample renewable energy (which could make CO2 conversion routes more economical), and the potential for CO2 utilisation to be either a stepstone or a small-scale substitute to CCS CO2 can be used commercially in some circumstances, such as in the production of building materials because it provides a superior product at a low cost than traditionally produced products. Products that require carbon, either because it provides their structure and qualities (carbon-containing compounds) or because the usage of carbon-free energy carriers, such as hydrogen or electricity, is difficult, could benefit from CO2 (for example, aviation fuels) (Muradov and Veziroğlu Citation2008). In addition there are some economic challenges to the production of the generation of the CO2 derived fuels such as variety of factors, including technology development, government policies, and market demand. One of the main challenges for CO2 derived fuels is the cost of production. The process of converting CO2 into fuels requires energy, and the current technologies used for this process are still relatively expensive compared to traditional fossil fuels. However, as the demand for carbon-neutral fuels increases, and more research and development is done, the cost of production is expected to decrease. Another factor that affects the feasibility of CO2 derived fuels is government policies and incentives. Many governments are implementing policies and providing funding to support the development and deployment of carbon-neutral technologies, including synthetic fuels. These policies can help to drive down the cost of production and create demand for these fuels. Finally, the market demand for carbon-neutral fuels is also an important factor. As consumers become more environmentally conscious, the demand for low-carbon transportation fuels is expected to increase. This could create a market for synthetic fuels, which would provide a viable alternative to traditional fossil fuels. Overall, while the economics feasibility of CO2 derived fuels is still uncertain, many experts believe that these fuels have the potential to play an important role in reducing greenhouse gas emissions and creating a more sustainable energy system in the future.
7. Conclusions
In this article, an overview of carbon capture technologies has been discussed in detail, along with the different uses of the captured carbon and their advantages and disadvantages. Four major technologies have been explored and discussed in length, such as pre, post, oxyfuel, and direct air capture. It has been observed that the CCS technologies have the upper edge over the CDR technologies as CO2 can be captured at the point of source, which leads to the capture and storage of huge amounts of CO2. But in the case of the CDR technologies, the carbon is captured from open air, and plants directly capture the CO2, which is very little in comparison to the CCS technologies. The governing bodies (United Nations Framework Convention on Climate Change (UNFCCC) and its member states, Intergovernmental Panel on Climate Change (IPCC) and the IEA) have decided to reduce carbon emissions and lower the temperature by 1.5 ℃ by 2050, which is only possible if the technologies are used effectively, and carbon emission sources are reduced to a great extent to curb greenhouse gases. Nowadays, the DACCS have been immensely used because new plants have been setup. According to the report, the Orca plant, which is a DACCS technology-based plant situated in Iceland, captures up to 4,000 tons of CO2 per year. At the same time some disadvantages of these technologies related to the use of the technologies in oil recovery and storage issues, have also been observed. But it has been seen that the technologies have been by far successful in serving their purpose. Because the captured carbon has not only been stored but has also been used in various areas such as agriculture, as chemical intermediates, and building. In addition, the cost analysis of the captured carbon turns out to be a deciding factor in the areas of carbon capture and some plants with more captured cost have been abandoned such as technologies based on IGCC. The lowest associated cost for the carbon captured has been seen in subcritical post-combustion, where the associated cost was 44.6 $/tCO2. Hence, the appropriate use of the technologies carries huge importance in different scenarios. Whereas carbon capture seems important, storage of carbon is also critical to the environment.
Glossary of the terms
CO | = | carbon monoxide |
CCU | = | Carbon capture and utilisation |
CDR | = | Carbon Dioxide Removal |
CCS | = | Carbon capture and sequestration |
DBU | = | 1,8-diazabicyclo[5.4.0]undec-7-ene |
PEG | = | Polyethylene glycol |
PSA | = | Pressure Swing Adsorption |
TSA | = | Temperature Swing Adsorption |
TSVA | = | Temperature Vacuum Swing Adsorption |
DBD | = | Dielectric-barrier discharge |
oxy-PC | = | Oxyfuel Combustion-Pulverised coal |
oxy-CFB | = | Oxyfuel Combustion-Circulating fluidised bed |
oxy-GT | = | Oxyfuel combustion for gas turbine-based power plants |
Mt/year | = | Mega tonnes per year also million tonnes per year |
Gt/year | = | Giga tonnes per year |
t | = | tonnes |
Disclosure statement
No potential conflict of interest was reported by the author(s).
Additional information
Notes on contributors
Narinder Singh
Narinder Singh is a civil engineer with a PhD in civil engineering from the University of Salerno, Italy, and a specialization in additive manufacturing. With over 6–7 years of experience in the field, he has expertise in additive manufacturing techniques and their applications in civil engineering. During his Ph.D., he worked on the in-house seismic isolator produced by using additive manufacturing. Further, he focused on the development of novel 3D printing techniques, and his work has been published in several reputable academic journals. Dr. Singh also has experience in various recycling of plastic solid waste materials such as HDPE and LDPE and handled various government-sponsored projects based on the recycling of plastic solid waste while working in India. After completing their PhD, he joined the Department of Engineering at the University of Naples (Parthenope), Naples, Italy, as a research fellow, where he continued his research in additive manufacturing and contributed to several projects involving the 3D printing of construction materials. Narinder Singh's expertise in additive manufacturing has allowed them to work on a diverse range of projects, including the development of materials, the recycling of plastic waste, the design and fabrication of structural components, and the use of 3D printing for on-site construction. He is the author of several scientific papers published in international peer-reviewed journals and conference proceedings.
Ilenia Farina
Ilenia Farina is a researcher in the Department of Engineering at the University of Naples Parthenope. She received her PhD in Energy Science and Engineering and her Master Degree in Civil Engineering from the University of Naples “Parthenope”. In 2013, she received a Master of Science in “Materials Science and Engineering” at the University of Sheffield (UK). Her research interests deal with the development of innovative materials for sustainable constructions. She has conducted experimental studies to demonstrate the employability of plastic fibres obtained from waste plastic as dispersed reinforcement in cementitious materials. Furthermore, she has also developed new fibre geometries, with the aim of enhancing the thermo-mechanical performance of fibre reinforced concrete and mortars. Her current research activity focuses on the development of new fibre-reinforced materials using conventional raw materials as well as inorganic waste materials. She is author of several scientific papers published in international peer-reviewed journal and international conference proceedings.
Antonella Petrillo
Antonella Petrillo PhD, is a professor at the Department of Engineering of the University of Naples “Parthenope”, Italy. She received her PhD in Mechanical Engineering from University of Cassino. Her research interests include multi-criteria decision analysis, industrial plant, logistic, manufacturing and safety. She serves as an Associate Editor for the International Journal of the Analytic Hierarchy Process. She is a member of AHP Academy and a member of several editorial boards.
Francesco Colangelo
Francesco Colangelo has a Ph.D. in Environmental Engineering from University of Basilicata. Even the PhD thesis, focused on innovative techniques to optimize the use of wastes materials in concrete, received an award. He has a Master of Science in Environmental Research ITC from University of Rome. Currently he is Full Professor of Materials Science and Technology at the University of Naples Parthenope. His main research fields are the recycling of wastes materials in concrete and in the geoenvironmental and civil applications, the application of Life Cycle Assessment methodology to different processes for preparation of innovative building material, the evaluation of durability of mortars and concrete, the stabilization/solidification of hazardous wastes and the synthesis of geopolymer eco-sustainable materials based on industrial waste. The above activities are also done in co-operation with international research centres “ Centre Scientifique et Technique du Bâtiment” (Paris), “Geopolymer Institute” (Saint-Quentin – France) and “Sustainable Technology Research Centre, Kingston University” (London). He has been and still is scientific manager of a number of research projects with public and private companies. Since 2012 he is a senior member of RILEM. He is director of Director of Laboratory of Environmental Material and Safety at the Department of Engineering of University of Naples Parthenope. All the above researches are proved by more than seventy papers, mostly on international journals, and presentation to international and Italian conferences.
Fabio De Felice
Fabio De Felice is a professor at at the Department of Engineering of the University of Naples “Parthenope”. He received his PhD in Mechanical Engineering at the University of Cassino and Southern Lazio. His current research focuses on multi-criteria decision-making analysis (with emphasis on AHP and ANP) and industrial, project and supply chain management. He has always been interested in the study of innovative processes aimed at improving industrial production. Expert in business management systems oriented towards process optimization and corporate digitalization. Careful observer of the dynamics of entrepreneurial growth of the digital economy. He is a member of the editorial boards of several international organizations and journals and has authored/co-authored numerous articles in the areas of decision science and business management. He is director of Director of Laboratory of LAPIS Lean Advanced Production and Industrial Sustainable systems lab at the Department of Engineering of University of Naples Parthenope.
References
- Acosta-Ramirez, A., M. Bertoli, D. G. Gusev, and M. Schlaf. 2012. “Homogeneous Catalytic Hydrogenation of Long-Chain Esters by an Osmium Pincer Complex and Its Potential Application in the Direct Conversion of Triglycerides into Fatty Alcohols.” Green Chemistry 14 (4): 1178–1188. https://doi.org/10.1039/c2gc15960k.
- Akay, G. 2020. “Plasma Generating—Chemical Looping Catalyst Synthesis by Microwave Plasma Shock for Nitrogen Fixation from Air and Hydrogen Production from Water for Agriculture and Energy Technologies in Global Warming Prevention.” Catalysts 10 (2): 152. https://doi.org/10.3390/catal10020152.
- Al‐Mamoori, A., A. Krishnamurthy, A. A. Rownaghi, and F. Rezaei. 2017. “Carbon Capture and Utilization Update.” Energy Technology 5 (6): 834–849. https://doi.org/10.1002/ente.201600747.
- Al-Janabi, N., P. Hill, L. Torrente-Murciano, A. Garforth, P. Gorgojo, F. Siperstein, and X. Fan. 2015. “Mapping the Cu-BTC Metal–Organic Framework (HKUST-1) Stability Envelope in the Presence of Water Vapour for CO2 Adsorption from Flue Gases.” Chemical Engineering Journal 281:669–677. https://doi.org/10.1016/j.cej.2015.07.020.
- Alturki, A. 2022. “The Global Carbon Footprint and How New Carbon Mineralization Technologies Can Be Used to Reduce CO2 Emissions.” ChemEngineering 6 (3): 44. https://doi.org/10.3390/chemengineering6030044.
- Balachandran, U., M. S. Kleefisch, T. P. Kobylinski, S. L. Morissette, and S. Pei. 1997. Oxygen ion conducting dense ceramic. U. S. Patent 5,580,497. United States (Chigago). https://patentimages.storage.googleapis.com/ea/ae/8c/2d4b80704ecb29/US5639437.pdf.
- Benson, S., Srinivasachar, S., Laudal, D., and Browers, B. 2014. Evaluation of Carbon Dioxide Capture from Existing Coal Fired Plants by Hybrid Sorption Using Solid Sorbents. U. North Dakota and Envergex. Final project meeting. DOE award DE-FE0007603. https://www.osti.gov/servlets/purl/1182546.
- Breault, R. W. 2010. “Gasification Processes Old and New: A Basic Review of the Major Technologies.” Energies 3 (2): 216–240. https://doi.org/10.3390/en3020216.
- Brennan, L., and P. Owende. 2010. “Biofuels from Microalgae—A Review of Technologies for Production, Processing, and Extractions of Biofuels and Co-Products.” Renewable and Sustainable Energy Reviews 14 (2): 557–577. https://doi.org/10.1016/j.rser.2009.10.009.
- Butler, C. D. 2018. “Climate Change, Health and Existential Risks to Civilization: A Comprehensive Review (1989–2013).” International Journal of Environmental Research and Public Health 15 (10): 2266. https://doi.org/10.3390/ijerph15102266.
- Chen, C. C., R. Prasad, and C. F. Gottzmann. 1999. Solid Electrolyte Membrane with Porous Catalytically-Enhancing Constituents. Chicago, United States: Google Patents.
- Churkina, G., A. Organschi, C. P. Reyer, A. Ruff, K. Vinke, Z. Liu, B. K. Reck, T. Graedel, and H. J. Schellnhuber. 2020. “Buildings as a Global Carbon Sink.” Nature Sustainability 3 (4): 269–276. https://doi.org/10.1038/s41893-019-0462-4.
- Colangelo, F., A. Petrillo, and I. Farina. 2021. “Comparative Environmental Evaluation of Recycled Aggregates from Construction and Demolition Wastes in Italy.” Science of the Total Environment 798:149250. https://doi.org/10.1016/j.scitotenv.2021.149250.
- D’Alessandro, D. M., B. Smit, and J. R. Long. 2010. “Carbon Dioxide Capture: Prospects for New Materials.” Angewandte Chemie International Edition 49 (35): 6058–6082. https://doi.org/10.1002/anie.201000431.
- Dias De Oliveira, M. E., B. E. Vaughan, and E. J. Rykiel. 2005. “Ethanol as Fuel: Energy, Carbon Dioxide Balances, and Ecological Footprint.” BioScience 55 (7): 593–602. https://doi.org/10.1641/0006-3568(2005)055[0593:EAFECD]2.0.CO;2.
- Dixit, F., K. Zimmermann, M. Alamoudi, L. Abkar, B. Barbeau, M. Mohseni, B. Kandasubramanian, and K. Smith. 2022. “Application of MXenes for Air Purification, Gas Separation and Storage: A Review.” Renewable and Sustainable Energy Reviews 164:112527. https://doi.org/10.1016/j.rser.2022.112527.
- Drage, T. C., C. E. Snape, L. A. Stevens, J. Wood, J. Wang, A. I. Cooper, R. Dawson, X. Guo, C. Satterley, and R. Irons. 2012. “Materials Challenges for the Development of Solid Sorbents for Post-Combustion Carbon Capture.” Journal of Materials Chemistry 22 (7): 2815–2823. https://doi.org/10.1039/C2JM12592G.
- Dreyfus, G. B., Y. Xu, D. T. Shindell, D. Zaelke, and V. Ramanathan. 2022. “Mitigating Climate Disruption in Time: A Self-Consistent Approach for Avoiding Both Near-Term and Long-Term Global Warming.” Proceedings of the National Academy of Sciences 119 (22): e2123536119. https://doi.org/10.1073/pnas.2123536119.
- Durmaz, T. 2018. “The Economics of CCS: Why Have CCS Technologies Not Had an International Breakthrough?” Renewable and Sustainable Energy Reviews 95:328–340. https://doi.org/10.1016/j.rser.2018.07.007.
- Fujikawa, S., R. Selyanchyn, and T. Kunitake. 2021. “A New Strategy for Membrane-Based Direct Air Capture.” Polymer Journal 53 (1): 111–119. https://doi.org/10.1038/s41428-020-00429-z.
- Ghiat, I., and T. Al-Ansari. 2021. “A Review of Carbon Capture and Utilisation as a CO2 Abatement Opportunity within the EWF Nexus.” Journal Of CO2 Utilization 45:101432. https://doi.org/10.1016/j.jcou.2020.101432.
- Groom, N. 2020. Problems Plagued US CO2 Capture Project Before Shutdown: Document. London (UK): Reuters.
- Guilbert, D., and G. Vitale. 2021. “Hydrogen as a Clean and Sustainable Energy Vector for Global Transition from Fossil-Based to Zero-Carbon.” Clean Technologies 3 (4): 881–909. https://doi.org/10.3390/cleantechnol3040051.
- Gutknecht, V., S. Ó. Snæbjörnsdóttir, B. Sigfússon, E. S. Aradóttir, and L. Charles. 2018. “Creating a Carbon Dioxide Removal Solution by Combining Rapid Mineralization of CO2 with Direct Air Capture.” Energy Procedia 146:129–134. https://doi.org/10.1016/j.egypro.2018.07.017.
- Habib, M., H. Badr, S. Ahmed, R. Ben‐Mansour, K. Mezghani, S. Imashuku, G. la O’, Y. Shao‐Horn, N. Mancini, and A. Mitsos. 2011. “A Review of Recent Developments in Carbon Capture Utilizing Oxy‐Fuel Combustion in Conventional and Ion Transport Membrane Systems.” International Journal of Energy Research 35 (9): 741–764. https://doi.org/10.1002/er.1798.
- Hansen, J., M. Sato, R. Ruedy, A. Lacis, and V. Oinas. 2000. “Global Warming in the Twenty-First Century: An Alternative Scenario.” Proceedings of the National Academy of Sciences 97 (18): 9875–9880. https://doi.org/10.1073/pnas.170278997.
- Hussin, F., and M. K. Aroua. 2020. “Recent Trends in the Development of Adsorption Technologies for Carbon Dioxide Capture: A Brief Literature and Patent Reviews.” Journal of Cleaner Production. (2014–2018)253:119707. https://doi.org/10.1016/j.jclepro.2019.119707.
- IEA (, I. E. A.) 2019a. Putting CO2 to usehttps://iea.blob.core.windows.net/assets/50652405-26db-4c41-82dc-c23657893059/Putting_CO2_to_Use.pdf.
- IEA (, I. E. A.) 2019b. The Role Of CO2 Storage https://www.iea.org/reports/the-role-of-CO2-storage.
- Irlam, L. 2017. “Global Costs of Carbon Capture and Storage.” Global CCS Institute 1: 16.
- Jerndal, E., T. Mattisson, and A. Lyngfelt. 2006. “Thermal Analysis of Chemical-Looping Combustion.” Chemical Engineering Research and Design 84 (9): 795–806. https://doi.org/10.1205/cherd05020.
- Kar, S., A. Goeppert, and G. S. Prakash. 2019. “Integrated CO2 Capture and Conversion to Formate and Methanol: Connecting Two Threads.” Accounts of Chemical Research 52 (10): 2892–2903. https://doi.org/10.1021/acs.accounts.9b00324.
- Kazemifar, F. 2022. “A Review of Technologies for Carbon Capture, Sequestration, and Utilization: Cost, Capacity, and Technology Readiness.” Greenhouse Gases: Science and Technology 12 (1): 200–230. https://doi.org/10.1002/ghg.2131.
- Keskin, S., T. M. van Heest, and D. S. Sholl. 2010. “Can Metal–Organic Framework Materials Play a Useful Role in Large‐Scale Carbon Dioxide Separations?” ChemSuschem 3 (8): 879–891. https://doi.org/10.1002/cssc.201000114.
- Korre, A., Z. Nie, and S. Durucan. 2010. “Life Cycle Modelling of Fossil Fuel Power Generation with Post-Combustion CO2 Capture.” International Journal of Greenhouse Gas Control 4 (2): 289–300. https://doi.org/10.1016/j.ijggc.2009.08.005.
- Lai, J. Y., L. H. Ngu, and S. S. Hashim. 2021. “A Review of CO2 Adsorbents Performance for Different Carbon Capture Technology Processes Conditions.” Greenhouse Gases: Science and Technology 11 (5): 1076–1117. https://doi.org/10.1002/ghg.2112.
- Ledley, T. S., E. T. Sundquist, S. E. Schwartz, D. K. Hall, J. D. Fellows, and T. L. Killeen. 1999. “Climate Change and Greenhouse Gases.” Eos Transactions American Geophysical Union 80 (39): 453–458. https://doi.org/10.1029/99EO00325.
- Liang, Z. H., W. Rongwong, H. Liu, K. Fu, H. Gao, F. Cao, R. Zhang, T. Sema, A. Henni, and K. Sumon. 2015. “Recent Progress and New Developments in Post-Combustion Carbon-Capture Technology with Amine Based Solvents.” International Journal of Greenhouse Gas Control 40:26–54. https://doi.org/10.1016/j.ijggc.2015.06.017.
- Liguori, S., K. Kian, N. Buggy, B. H. Anzelmo, and J. Wilcox. 2020. “Opportunities and Challenges of Low-Carbon Hydrogen via Metallic Membranes.” Progress in Energy and Combustion Science 80:100851. https://doi.org/10.1016/j.pecs.2020.100851.
- Liu, A. H., R. Ma, C. Song, Z. Z. Yang, A. Yu, Y. Cai, L. N. He, Y. N. Zhao, B. Yu, and Q. W. Song. 2012. “Equimolar CO2 Capture by N‐Substituted Amino Acid Salts and Subsequent Conversion.” Angewandte Chemie International Edition 51 (45): 11306–11310. https://doi.org/10.1002/anie.201205362.
- Mahasenan, N., S. Smith, and K. Humphreys 2003. The Cement Industry and Global Climate Change: Current and Potential Future Cement Industry CO2 Emissions. Proceedings of the 6th International Conference on Greenhouse Gas Control Technologies, Kyoto, Japan, October 2002 1–4.
- Markewitz, P., W. Kuckshinrichs, W. Leitner, J. Linssen, P. Zapp, R. Bongartz, A. Schreiber, and T. E. Müller. 2012. “Worldwide Innovations in the Development of Carbon Capture Technologies and the Utilization of CO 2.” Energy & Environmental Science 5 (6): 7281–7305. https://doi.org/10.1039/c2ee03403d.
- Mas, B., A. Cladera, T. Del Olmo, and F. Pitarch. 2012. “Influence of the Amount of Mixed Recycled Aggregates on the Properties of Concrete for Non-Structural Use.” Construction and Building Materials 27 (1): 612–622. https://doi.org/10.1016/j.conbuildmat.2011.06.073.
- Massai, L., and C.-M. Beyet. 2018. “Current Developments in Carbon & Climate Law.” Carbon & Climate Law Review 12 (3): 272–277. https://doi.org/10.21552/cclr/2018/3/13.
- McQueen, N., K. V. Gomes, C. McCormick, K. Blumanthal, M. Pisciotta, and J. Wilcox. 2021. “A Review of Direct Air Capture (DAC): Scaling Up Commercial Technologies and Innovating for the Future.” Progress in Energy 3 (3): 032001. https://doi.org/10.1088/2516-1083/abf1ce.
- Mikhaylov, A., N. Moiseev, K. Aleshin, and T. Burkhardt. 2020. “Global Climate Change and Greenhouse Effect.” Entrepreneurship & Sustainability Issues 7 (4): 2897. https://doi.org/10.9770/jesi.2020.7.4(21).
- Mohanty, A., M. A. Misra, and G. Hinrichsen. 2000. “Biofibres, Biodegradable Polymers and Biocomposites: An Overview.” Macromolecular Materials and Engineering 276 (1): 1–24. https://doi.org/10.1002/(SICI)1439-2054(20000301)276:1<1:AID-MAME1>3.0.CO;2-W.
- Montzka, S. A., E. J. Dlugokencky, and J. H. Butler. 2011. “Non-CO2 Greenhouse Gases and Climate Change.” Nature 476 (7358): 43–50. https://doi.org/10.1038/nature10322.
- Morris, R. E., and P. S. Wheatley. 2008. “Gas Storage in Nanoporous Materials.” Angewandte Chemie International Edition 47 (27): 4966–4981. https://doi.org/10.1002/anie.200703934.
- Mülhaupt, R. 2013. “Green Polymer Chemistry and Bio‐Based Plastics: Dreams and Reality.” Macromolecular Chemistry and Physics 214 (2): 159–174. https://doi.org/10.1002/macp.201200439.
- Muradov, N. Z., and T. N. Veziroğlu. 2008. ““Green” Path from Fossil-Based to Hydrogen Economy: An Overview of Carbon-Neutral Technologies.” International Journal of Hydrogen Energy 33 (23): 6804–6839. https://doi.org/10.1016/j.ijhydene.2008.08.054.
- Nelson, D. D., B. McManus, S. Urbanski, S. Herndon, and M. S. Zahniser. 2004. “High Precision Measurements of Atmospheric Nitrous Oxide and Methane Using Thermoelectrically Cooled Mid-Infrared Quantum Cascade Lasers and Detectors.” Spectrochimica Acta Part A: Molecular and Biomolecular Spectroscopy 60 (14): 3325–3335. https://doi.org/10.1016/j.saa.2004.01.033.
- Olabi, A., K. Obaideen, K. Elsaid, T. Wilberforce, E. T. Sayed, H. M. Maghrabie, and M. A. Abdelkareem. 2022. “Assessment of the Pre-Combustion Carbon Capture Contribution into Sustainable Development Goals SDGs Using Novel Indicators.” Renewable and Sustainable Energy Reviews 153:111710. https://doi.org/10.1016/j.rser.2021.111710.
- Olajire, A. A. 2010. “CO2 Capture and Separation Technologies for End-Of-Pipe Applications–A Review.” Energy 35 (6): 2610–2628. https://doi.org/10.1016/j.energy.2010.02.030.
- Olhoff, A., and J. M. Christensen 2018. Emissions Gap Report 2018. UNEP DTU Partnership: Copenhagen, Denmark.
- O’Neill, S. 2021. Direct Air Carbon Capture Takes Baby Steps—Giant Strides are Needed. Elsevier.
- Palermo, V., and Y. Hernandez. 2020. “Group Discussions on How to Implement a Participatory Process in Climate Adaptation Planning: A Case Study in Malaysia.” Ecological Economics 177:106791. https://doi.org/10.1016/j.ecolecon.2020.106791.
- Paustian, K., C. V. Cole, D. Sauerbeck, and N. Sampson. 1998. “CO2 Mitigation by Agriculture: An Overview.” Climatic Change 40 (1): 135–162. https://doi.org/10.1023/A:1005347017157.
- Peters, M., B. Köhler, W. Kuckshinrichs, W. Leitner, P. Markewitz, and T. E. Müller. 2011. “Chemical Technologies for Exploiting and Recycling Carbon Dioxide into the Value Chain.” ChemSuschem 4 (9): 1216–1240. https://doi.org/10.1002/cssc.201000447.
- Quadrelli, E. A., G. Centi, J. L. Duplan, and S. Perathoner. 2011. “Carbon Dioxide Recycling: Emerging Large‐Scale Technologies with Industrial Potential.” ChemSuschem 4 (9): 1194–1215. https://doi.org/10.1002/cssc.201100473.
- Rego, R. M., M. D. Kurkuri, and M. Kigga. 2021. “Sustainable Green Approaches in Sorption-Based Defluoridation: Recent Progress.” Green Technologies for the Defluoridation of Water 1: 141–174.
- Reis, D. D. C., P. B. Golgher, A. S. Silva, and A. Laender 2004. Automatic Web News Extraction Using Tree Edit Distance. Proceedings of the 13th international conference on World Wide Web, New York, NY, USA.
- Rissman, J., C. Bataille, E. Masanet, N. Aden, W. R. Morrow III, N. Zhou, N. Elliott, R. Dell, N. Heeren, and B. Huckestein. 2020. “Technologies and Policies to Decarbonize Global Industry: Review and Assessment of Mitigation Drivers Through 2070.” Applied Energy 266:114848. https://doi.org/10.1016/j.apenergy.2020.114848.
- Schwartz, M., J. H. White, and A. F. Sammels. 2000. Solid State Oxygen Anion and Electron Mediating Membrane and Catalytic Membrane Reactors Containing Them. Chigaco, Unites States: Google Patents.
- Sheldon, D. J., and M. R. Crimmin. 2022. “Repurposing of F-Gases: Challenges and Opportunities in Fluorine Chemistry.” Chemical Society Reviews 51 (12): 4977–4995. https://doi.org/10.1039/D1CS01072G.
- Shen, M., W. Huang, M. Chen, B. Song, G. Zeng, and Y. Zhang. 2020. “(Micro) Plastic Crisis: Un-Ignorable Contribution to Global Greenhouse Gas Emissions and Climate Change.” Journal of Cleaner Production 254:120138. https://doi.org/10.1016/j.jclepro.2020.120138.
- Smith, P. 2016. “Soil Carbon Sequestration and Biochar as Negative Emission Technologies.” Global Change Biology 22 (3): 1315–1324. https://doi.org/10.1111/gcb.13178.
- Song, C. 2006. “Global Challenges and Strategies for Control, Conversion and Utilization of CO2 for Sustainable Development Involving Energy, Catalysis, Adsorption and Chemical Processing.” Catalysis Today 115 (1–4): 2–32. https://doi.org/10.1016/j.cattod.2006.02.029.
- Stanger, R., T. Wall, R. Spörl, M. Paneru, S. Grathwohl, M. Weidmann, G. Scheffknecht, D. McDonald, K. Myöhänen, and J. Ritvanen. 2015. “Oxyfuel Combustion for CO2 Capture in Power Plants.” International Journal of Greenhouse Gas Control 40:55–125. https://doi.org/10.1016/j.ijggc.2015.06.010.
- Sunarso, J., S. Baumann, J. Serra, W. Meulenberg, S. Liu, Y. Lin, and J. D. Da Costa. 2008. “Mixed Ionic–Electronic Conducting (MIEC) Ceramic-Based Membranes for Oxygen Separation.” Journal of Membrane Science 320 (1–2): 13–41. https://doi.org/10.1016/j.memsci.2008.03.074.
- UNFCCC. 1992. https://unfccc.int/resource/docs/convkp/conveng.pdf
- UNEP. 2021. https://www.unep.org/resources/annual-report-2021
- Voskian, S., and T. A. Hatton. 2019. “Faradaic Electro-Swing Reactive Adsorption for CO 2 Capture.” Energy & Environmental Science 12 (12): 3530–3547. https://doi.org/10.1039/C9EE02412C.
- Wilcox, J. 2012. Carbon Capture. Springer Science & Business Media. https://doi.org/10.1007/978-1-4614-2215-0.
- Yadav, S., and S. Mondal. 2022. “A Review on the Progress and Prospects of Oxy-Fuel Carbon Capture and Sequestration (CCS) Technology.” Fuel 308:122057. https://doi.org/10.1016/j.fuel.2021.122057.
- Yang, Z.-Z., L.-N. He, Y.-N. Zhao, B. Li, and B. Yu. 2011. “CO2 Capture and Activation by Superbase/Polyethylene Glycol and Its Subsequent Conversion.” Energy & Environmental Science 4 (10): 3971–3975. https://doi.org/10.1039/c1ee02156g.
- Yang, B., Y. M. Wei, L. C. Liu, Y. B. Hou, K. Zhang, L. Yang, and Y. Feng. 2021. “Life Cycle Cost Assessment of Biomass Co-Firing Power Plants with CO2 Capture and Storage Considering Multiple Incentives.” Energy Economics 96:105173. https://doi.org/10.1016/j.eneco.2021.105173.
- Yang, H., Z. Xu, M. Fan, R. Gupta, R. B. Slimane, A. E. Bland, and I. Wright. 2008. “Progress in Carbon Dioxide Separation and Capture: A Review.” Journal of Environmental Sciences 20 (1): 14–27. https://doi.org/10.1016/S1001-0742(08)60002-9.
- Yoro, K. O., and M. O. Daramola. 2020. “CO2 Emission Sources, Greenhouse Gases, and the Global Warming effect.” in Advances in Carbon Capture, 3–28. Elsevier.
- Zhang, Z., T. N. G. Borhani, and M. H. El-Naas. 2018. “Carbon Capture.” Exergetic, Energetic and Environmental Dimensions 997–1016. https://doi.org/10.1016/b978-0-12-813734-5.00056-1.
- Zhang, R., and J. Zhang. 2021. “Long-Term Pathways to Deep Decarbonization of the Transport Sector in the Post-COVID World.” Transport Policy 110:28–36. https://doi.org/10.1016/j.tranpol.2021.05.018.
- Zhang, K., G. Zhang, X. Liu, A. N. Phan, and K. Luo. 2017. “A Study on CO2 Decomposition to CO and O2 by the Combination of Catalysis and Dielectric-Barrier Discharges at Low Temperatures and Ambient Pressure.” Industrial & Engineering Chemistry Research 56 (12): 3204–3216. https://doi.org/10.1021/acs.iecr.6b04570.
- Zhou, Z., F. Yu, and J. Ma. 2021. “Nanoconfinement Engineering for Enchanced Adsorption of Carbon Materials, Metal–Organic Frameworks, Mesoporous Silica, MXenes and Porous Organic Polymers: A Review.” Environmental Chemistry Letters 20 (1): 1–33. https://doi.org/10.1007/s10311-021-01355-z.
- Zhu, Q. 2019. “Developments on CO2-utilization Technologies.” Clean Energy 3 (2): 85–100. https://doi.org/10.1093/ce/zkz008.