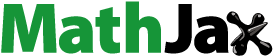
ABSTRACT
In this 3D numerical study, the effects of cathode and anode functional layer thicknesses on the performance of solid oxide fuel cells under different operating parameters are analysed and elucidated. The performance of the model is evaluated using direct ammonia and an equivalent amount of hydrogen fuel with functional layers ranging from 0 to 50 μm. The findings unveiled that the cathode functional layer has a stronger influence on cell performance than the anode functional layer. The parametric analysis also showed that the effect of temperature gradually weakened with the increase of the cathode functional layer which is the exact opposite of what anode functional layers do. This gives more promising result for the cathode functional layer than the anode functional layer, especially for hydrogen-powered cells. However, the anode functional layer works best when green ammonia is supplied directly to the solid oxide fuel cell. In this regard, the 10 μm functional layers of the anode and cathode layers achieved the highest possible power density using ammonia and hydrogen fuels under similar operating conditions. This suggests that the anode layer has outstanding performance than the cathode counterparts for ammonia-fuelled cells and the layers of the cathode have better performance for hydrogen-fuelled cells. It is also observed that the performance of the cell is decreasing while the thickness of the functional layer is increasing particularly after 10 μm. The study also confirmed that solid oxide fuel cells with functional layers have outstanding performance over corresponding cells without functional layers. Thus, the finding of this study concluded that the functional layer thickness is strongly dependent on the types of fuel and electrodes used in the cells.
1. Introduction
Today, carbon-rich fuels are a vital source of energy applied for powering human beings, and they will be anticipated to continue for the next few decades. Connecting with this, modern and efficient energy technologies are essential to convert the available limited fossil fuel into useful energy efficiently with no or trivial environmental impression in addition to renewable energy development. Amongst all kinds of power production systems, SOFCs running with intermediate to high temperatures are emerging as frontrunners in chemical to electrical energy transformation efficiency, fuel diversity, and negligible environmental impact.
Hydrogen has potential applications in stationary, mobile, and transportation, but production, storage, and infrastructure issues hinder its implementation. Hydrogen can be deposited in ammonia, which is cheaper, carbon-neutral, and has more stored energy than hydrogen. Solid oxide fuel cells (SOFCs) are essential for using ammonia as a cost-effective green fuel due to their high-temperature setup and ability to utilise various fuels. SOFCs are an all-ceramic device for green power generation, operating at temperatures ranging from 500 to 1000οC (Atkinson et al. Citation2004; Etemadi et al. Citation2016; Kakaç, Pramuanjaroenkij, and Zhou Citation2007; Mench Citation2008; Walther and Ahn Citation2011). They have high efficiency, essay scalability(built-in different sizes), and the flexibility of fuels (Jiang et al. Citation2006; Su et al. Citation2015). It also provides high-quality waste heat that can be recovered through cogeneration (Singhal Citation2000) with minimal pollutants and greenhouse gas emissions even when carbon-rich fuels are applied. For these reasons, they are receiving more thoughtfulness and nice looks from different stakeholders.
Furthermore, SOFCs are efficient and eco-friendly power-generating electrochemical devices that can be used in residential, commercial, industrial, and military applications (Singhal Citation2002). They contribute significantly to global energy needs and address environmental issues caused by fossil fuel burning. The core components of a SOFC are dense solid ceramic electrolytes (mainly YSZ) sandwiched in anode and cathode electrodes and interconnects. These components must be chemically and thermally compatible, stable, and have acceptable electrical conductivity and thermal expansion coefficients. Functional layers with sponge-like micro-pores can reduce mismatch stress between the anode and electrolyte, lowering contact resistance, stabilising components, equalising TECs, and facilitating electrochemical reactions. A thinner anode functional layer (AFL) than EAT is insufficient for fuel oxidation, while greater thickness leads to gas transit concentration loss and limited cell performance. Determining EAT is crucial to balance concentration and activation resistance, allowing high-performance SOFCs (Akduman and Soydan Citation2023). Thus, improving electrochemical cell performance via functional layers is crucial for the sustainable utilisation of SOFCs.
Kagomiya et al., (Citation2017) fabricated anode-supported (AS)-SOFCs to study the power density dependence of the AFL. Their study confirmed that SOFCs achieved the highest power densities when 10 μm AFLs were introduced between AS and electrolyte. Functional layers of SOFCs are fabricated using techniques such as tap-casting, dip coating, screen printing, dry pressing, spray, and vacuum slip casting (Chen et al. Citation2008, Citation2019; Develos-Bagarinao et al. Citation2021; Kagomiya et al. Citation2017). Kagomiya et al., (Citation2015) also fabricated AS-SOFCs with Positive-Electrolyte-Negative electrode assembly (PENs) of Ni-YSZ/YSZ/LSM to study the performance of cells with and without AFLs. The AFL thicknesses considered in the study ranged from 20 to 80 μm. The findings show that higher power densities are achieved with AFLs compared to SOFCs without AFLs due to activation-induced polarisation and reduced ohmic resistance compared to diffusion resistance losses. Takahashi et al., (Citation2021) also fabricated a micro-anode functional coating between the anode and electrolyte in SOFC using a 3D printer and tested it using dry methane fuel. The study reveals that adding a functional layer to the anode support enhances SOFC performance, achieving a maximum power density of 302 mW/cm2 at 873K.
The redox reaction occurs at the triple phase boundary (TPB) interface, where gas, ion, and electron-conducting phases come together. The study of Konno et al., (Citation2012) found that only 10–20 μm thickness of the electrode-electrolyte interface is involved in electrochemical reactions to generate electricity in SOFCs. Increased effective thickness of the TPB leads to better power performance, as the characteristic length of ion conduction increases. Similarly, K. Chen et al., (Citation2008) explored methods to enhance the effective TPB density by integrating it with functional layers. They found that introducing an AFL in SOFCs significantly improved performance by 2.2 times in power and 1.4 times in the effective area of TPB.
X. Chen et al., (Citation2019) designed and fabricated multilayer AS-SOFCs, which were tested with humidified hydrogen, and achieved a maximum power density of 360 mA/cm2 at 0.75 V and 1073 K. The study highlights the significance of CFLs and AFLs in SOFC performance, highlighting the need for improved performance in these layers. X. Chen et al., (Citation2018) have also performed an experimental study to investigate the effects of an AFL on the electrochemical performance of AS-SOFCs. They found that the introduction of an AFL significantly enhanced the performance of the cells due to increased TPB. The cells were tested with humidified H2 (3% vol H2O) and ambient air at the anode and cathode side compartments, respectively, resulting in a peak power density of 906 mW/cm2 at 7.6 μm thick AFL and 973K. As well, an excellent power density of 558 mW/cm2 is obtained when the anode side is fed with diluted H2 (97vol (10% H2–90% N2).
A wide range of electrochemical active thickness (EAT) values has been reported in the literature. The determination of EAT is crucial for optimising SOFC electrodes and TPB, thereby increasing cell performance. Studies have shown that EAT increases with increasing effective ionic conductivity, decreasing exchange current density, and decreasing TPB length (Asmare Alemu and Ilbas Citation2020; Asmare et al. Citation2022). S. Chen et al., (Citation2020) also fabricated SOFC with AFLs and tested with humidified hydrogen, achieving maximum power densities of 365 and 598 mW/cm2 at 1073K without and with AFL, respectively. Gross et al., (Citation2007) prepared AFLs by impregnating ceria and catalytic metals into YSZ and testing with humidified hydrogen at 973K. Hassan et al., (Citation2002) also developed an optimised AFL for SOFC and tested it using hydrogen fuel and dry air as the oxidant. The study investigated the effect of AFL thickness and Ni content on cell performance. The generated current was measured at various thicknesses of AFL (2, 5, 8,16 μm) at a cell voltage of 0.7 V and a working temperature from 1023 to 1223K with an interval of 50K. The results showed that cells with 16 μm AFL had a lower current density and that increasing the volume content of Ni above 40% declined cell performance. Kenney and Karan (Citation2007) also studied the effects of microstructure of cathode electrodes on SOFC performance numerically. Their study revealed that increasing cathode layer porosity from 30 to 50% leads to improved oxygen distribution and electrochemical performance in cells. Yet, increasing porosity is detrimental due to TPB coverage and efficacious conductivity decrease with increasing porosity.
Furthermore, studies suggest that integrating a cathode electrode with a CFL can reduce cell polarisation resistance and improve cell operation. Connecting with this, Chrzan et al., (Citation2017) have fabricated Nano crystalline CFLs for SOFCs and observed that the performance of the cathode was enhanced. The conventional thickness of CFL in AS-SOFC is 10 μm, and the thickness of the entire cathode electrode is 50 mm (Molin et al. Citation2016). Develos-Bagarinao et al., (Citation2021) have manufactured CFLs in AS-SOFC. Their results confirm that the electrochemical performance of the cathode is significantly improved.
The most often used material as an anode, electrolyte, and cathode of SOFC is a porous cermet made of Ni and YSZ (Ni-YSZ), YSZ (8 mol% Y2O3–ZrO2), and LSM (La0.8Sr0.2MnO3), respectively. In addition to this, the functional layer is made of a mixture of electrodes and electrolytes in apt proportions. At the electrolyte/cathode contact, the mesoporous nanosized CFL structure lowered polarisation resistance by 40% (Shipilova et al. Citation2021). This study uses an anode support with 60% NiO and 40% YSZ, YSZ electrolyte, and an LSM cathode electrode, while functional layers AFL and CFL have 60% YSZ and 40% NiO, and 50% LSM and 50% YSZ, respectively.
Typically, functional layers increase the area of the TPB, which in turn reduces polarisation due to activation and ohmic resistance (Kagomiya et al. Citation2015). However, the ohmic resistance increases due to the thicker AFL (Chen et al. Citation2008) which is contrasted with the study of Kagomiya et al., (Citation2015). As well, raising the thickness of the AFL without a systematic approach enhances the concentration polarisation. This is because the thickness of the AFL is denser than that of the fuel electrode support (Kagomiya et al. Citation2015). Sivasankaran et al., Citation2022’s study examined the impact of AFL and cathode thickness on the performance of SOFCs made of Ni-GDC/GDC/LSCF anode, electrolyte, and cathode materials. The study characterised electrochemical cells using a 10 cm2 active surface area and operating temperature from 773K to 823K. Results showed that AFL did not improve SOFC performance, and attained a peak power density of 370 mW/cm2 at 823K and a 50 μm cathode thickness. This suggests a gap in the effective role of functional layers, necessitating a systematic study of a wider range of functional layer thicknesses.
To reduce polarisation resistance due to concentration, increasing anode support porosity is the most effective technique. However, this also increases cell polarisation due to the activation barrier. The effect of concentration and activation loss is non-linearly correlated, suggesting that smoothing the contact area of electrolytes and electrodes with a functional layer could help overcome these issues. Thus, in this study, the impact of the anode and cathode functional layers on the performance of the model was numerically examined using COMSOL Multiphysics. The degree of its impact on power density performance is also compared with SOFCs without functional layers. Related to this, AFL and CFL thicknesses ranging from 0 to 50 μm have been used for this numerical simulation.
2. Mathematical modeling of anode-supported solid oxide fuel cell
In this study, the performance of AS-SOFC with and without functional layers was numerically evaluated by developing a three-dimensional mathematical model using the commercially available COMSOL Multiphysics. For mass, momentum, energy, species, and charge, the governing partial differential equations have been linearised and solved using the finite element method (FEM). displays the geometric characteristics utilised in model development and numerical simulation. Further, the developed AS-SOFC model with different functional layers and its corresponding mesh is shown in . The operating variables coupled with fitting parameters and fundamental governing equations are also listed in respectively. The anode and cathode electrochemically active surface area per unit volume and their charge transfer coefficients were considered fitting parameters.
Figure 1. Model geometry of AS-SOFC coupled functional layers.

Table 1. The geometry of the model (Asmare Citation2022; Park et al. Citation2018; Shen et al. Citation2019).
Table 2. Input variable and fitting parameters.
Table 3. Governing equation (Asmare Citation2022; Asmare Citation2021, Citation2022; Asmare and Ilbas Citation2020; Asmare, Ilbas, and Yalcin Citation2021; Ilbas et al. Citation2020; Molla and Ilbas Citation2020).
2.1. Basic assumptions and working conditions
In this numerical study, isothermal temperature, uniform fluid velocity, partial pressure, and viscosity in all systems were the basic assumptions considered during model development. In addition to this, it is also assumed that no chemical reaction takes place in both gas flow channels, ammonia doesn’t participate in the electrochemical reactions, and the density of the reacting gas is constant on average when operating under stable conditions. The no-slip condition, the uniform potential at the gas channel interface and the electrodes, the negligible boundary layer at the gas flows, and zero fluxes at the electrode and electrolyte ends are also set as boundary conditions to solve various coupled partial differential equations.
2.2. Electrochemical modeling
The oxidation-reduction process between fuel and oxidiser, primarily air, is the electrochemical reaction. In this simulation, the anode is given wet hydrogen and pure ammonia, and the cathode electrodes are fed air. At the anode electrode surface, ammonia is thought to split into free hydrogen and nitrogen before reaching the electrochemical active site. Thus, the expected redox electrochemical reaction of ammonia and hydrogen-fuelled SOFC using oxide ion transfer is presented as follows.
Direct ammonia-fuelled SOFC
Hydrogen fuelled SOFC
The open-circuit voltage (VOC) and the operational voltage are equivalent. According to the formulae illustrated below (Cheddie Citation2013), the equilibrium voltage at the air electrode and the Nernst voltage are also equivalent.
Where, PO2, PH2, and PH2O represent the partial pressure of oxygen, water vapour, and hydrogen at the cathode and anode-electrolyte interface, correspondingly.
Likewise, the cell voltage (Vcell) drops as a result of diffusion, internal charge transfer, and conduction losses. Hence, the value of Vcell is calculated using EquationEquation (9)(9)
(9) and the voltage losses (
) in terms of activation (ηact), concentration (ηcon), and ohmic polarisation (ηohm) at each electrode is expressed in EquationEquation (10)
(10)
(10) .
Stefan diffusion model was used in this work to calculate species and mass balances in porous electrodes and gas channels. This is due to the fact that diffusion and convection are the main driving forces behind mass transport. The Brinkman equation is also used to calculate the velocity and pressure field for momentum balance over the porous medium. Also, the transfer of charge between ionic, electronic, and conductive phases is determined using Ohm’s law. Thus, provides a summary of the fundamental governing equations for charge carriers, species, conservation of momentum, energy, and mass. Fuller correlation is also used to calculate the molecular diffusion of gases; where M is the molecular weight in kg/mol and υ is the specific Fuller diffusion volume, as shown below;
Here,, µ, ρ,
,
,
and
denotes the density of the reactant species, the electrolyte thickness, the dynamic viscosity of gases, the change in entropy during the electrochemical reaction, the source term for the consumption and generation of mass at TPB during an electrochemical reaction, volumetric heat sources, the source term for momentum and velocity respectively.
3. Results and discussion
The effect of the electrode functional layer has been analysed and elucidated in this three-dimensional numerical study. The model’s performance is also evaluated in terms of polarisation and power density curves using ammonia and hydrogen gas. The parametric sweep analysis was carried out by changing the anode and cathode functional layers from non to 50 μm. The effect of functional layers (FLs) was investigated alternately, meaning that all parameters and the thickness of the remaining PEN were kept constant at the design point when the influence of the AFLs was studied. Similar procedures have been also employed while examining the effect of CFLs on SOFC performance.
For the baseline, a planar solid oxide fuel cell without FLs was developed and analysed using COMSOL Multiphysics. shows the polarisation and power density curves for the developed model without FLs. In relation to this, under similar operating conditions, the maximum possible power densities achieved by NH3- and H2-fuelled AS-SOFCs without introducing FLs are 400 and 413.8 mW/cm2, respectively. Thereafter, a numerical performance comparison between the anode and cathode functional layers of AS-SOFC has been performed. The result indicates that the CFL has a greater influence than the anode layers, especially for hydrogen-fuelled SOFC. Further, the effects of temperature decrease as the FL of the cathode increases, but this is opposite to the effects of AFLs. This yields a more promising result for the CFL than AFL in hydrogen-powered cells. However, when green ammonia is directly fed into a SOFC, the performance of AFL is superior to that of CFL. In this regard, under similar operating conditions, the maximum plausible power density achieved was 420 mW/cm2 for AFL and 440 mW/cm2 for CFL in hydrogen-fuelled AS-SOFC at 10 μm as illustrated in .
For ammonia-fuelled cells, the AFL and CFL give a power density of 430.97 and 419.04 mW/cm2 using identical layer thicknesses and working environments. This implies that the anode layer outperforms the cathode counterparts for ammonia, while the cathode layers outperform the anode counterparts for hydrogen-fuelled cells. It is also observed that the performance of the cell decreases as the thickness of the functional layer increases this is because a thicker functional layer results in higher ohmic and concentration losses. This study also concluded that the thickness of the FLs is strongly dependent on the type of fuel used.
demonstrate the effects of anode and cathode functional layers when varied from 0 to 50 μm (10, 20, 30, 40, 50) at a constant electrolyte thickness of 15 μm. The figure explained that the performance of the cell is increasing up to 10 μm thicken of functional coating and the plausible peak power density was obtained at 10 μm. It is also observed that the performance of the cells decreases as the thickness of the AFL increases. In this regard, the maximum power density varies from 420 mW/cm2 at 10 µm to around 340 mW/cm2 at 50 µm AFL thickness. On the other hand, the performance of the cell integrated with CFL changes from 440 mW/cm2 at 10 µm to 408.54 mW/cm2 at 50 µm thickness. This shows that the CFL outperforms the AFL using hydrogen fuel but the result is reversed in ammonia-fuelled cells as presented in . The reason is due to higher ohmic losses in cells with thicker AFLs and poor conductivity within the AFL. Also, ammonia needs a slightly higher temperature to facilitate the endothermic dissociation into free hydrogen and nitrogen. For these reasons, the anode functional layer plays a huge role in promoting the electrochemical reaction of hydrogen released from ammonia at the anode-electrolyte interface. Hydrogen, on the other hand, does not require much temperature since it is a free reactive gas that is read for electrochemical reactions. Consequently, functional layers in the anode are less needful for pure hydrogen compared with the air electrode. However, the cathode functional layer must be included because oxygen is less reactive than hydrogen. The addition of CFL can accelerate the reaction of oxygen so that the SOFC has good performance. The benefits of CFL are mainly due to the increased oxygen concentration in the TPB interface region, which enlarges the reactive area, and the increased cross-section of the current transport path, which significantly reduces the cathodic ohmic polarisation. Aligned with this, significant efforts have been made to improve the performance of SOFCs by employing composite cathodes (Hong and Suk Citation2006). The air electrode composed of La1-xSrxMnO3+δ (LSM) and YSZ has been intensively investigated to diminish the polarisation of the cathode. It has been reported that the addition of YSZ to the LSM cathode increases the TPB area, extends the reaction sites to the electrode, and significantly reduces the cathode polarisation.
The performance of the model is also evaluated using a 10 µm thickness of AFL and CFL that gives a maximum power density. According to the findings of this numerical study, a solid oxide fuel cell integrated with both AFL and CFL outpaces a SOFC coupled with single functional layers as indicated in . The results revealed that the peak power density obtained using hydrogen is 600.78 mW/cm2, which is 43% and 36.5% higher than AS-SOFC combined with AFL and CFL, respectively. However, using ammonia gives a power density of 578.23 mW/cm2, which is also higher by 34.16 and 37.99% from AS-SOFC combined with AFL and CFL, respectively. As can be understood easily from the above figure, the numerical performance of hydrogen-fuelled cells is slightly higher than the corresponding NH3-AS-SOFC under similar operating conditions. The numerical power density value obtained for hydrogen is higher by 22.5 mW/cm2 from ammonia-supplied AS-SOFC.
Figure 6. The effects of the functional layer on the performance of the solid oxide fuel cell.

Further, Kagomiya et al., (Citation2017) experimental work on the effect of anode functional layer thickness (10 μm) on power density in AS-SOFCs was used for model validation and elucidation. As shown in , the numerical simulation results of the study for anode functional layered AS-SOFC are slightly agreeing with the experimental results of Kagomiya et al., (Citation2017). The primary reason for using hydrogen-fuelled AS-SOFC for model validation for both cathode and anode functional effects on the performance of AS-SOFC is that no data has been reported using direct ammonia-supplied AS-SOFC to date. Furthermore, the slight variations in the observed results are due to differences in cell component materials and geometrical component thicknesses, which must receive special attention.
Figure 7. Model validation using the experimental result of Kagomiya et al. (Citation2017).

4. Conclusion
The primary goal of this research is to improve cell performance by introducing different functional layers. Accordingly, a 3D numerical model of AS-SOFC is developed and a numerical analysis has been conducted to evaluate its performance with and without functional layers using ammonia and hydrogen as primary fuel. The performance of this numerical model is examined with functional layers ranging from 0 to 50 μm thickness. The nonlinear governing equations for momentum, mass, charge balance, gas-phase matter, and energy coupled with electro-kinetic equations are changed into linear equations and solved by finite element analysis methods using COMSOL Multiphysics software. Therefore, the conclusion of the present study reveals that;
Solid oxide fuel cells with functional layers have outstanding performance compared to corresponding cells without functional layers when operated under similar working circumstances.
For hydrogen-fuelled cells, the cathode functional layer has a greater influence on SOFC performance than the anode functional layer, whereas the anode functional layer works best when green ammonia is supplied directly to the SOFC. Nonetheless, both achieve the highest power density at the 10 μm functional layer.
Another surprising finding of the study confirmed that the thickness of the functional layer is strongly dependent on the type of fuel and electrodes used in the cells.
The performance of hydrogen-fuelled is higher than the corresponding ammonia-fuelled cells under a similar working environment in both cases. The main reason for this is the lower ohmic polarisation of hydrogen fuel cells compared to ammonia fuel cells.
Furthermore, the model was validated and the numerical simulation results of this study were found to be in good agreement with the experimental results of Kagomiya et al., (Citation2017).
Acknowledgments
I am enthusiastic to acknowledge Bahir Dar Energy Center of Bahir Dar Institute of Technology, Bahir Dar University for its continuous support.
Disclosure statement
No potential conflict of interest was reported by the author(s).
Data availability statement
The datasets for this numerical investigation are not available as all operational and fitting parameters, as well as the software used for numerical analysis, are supplied in the manuscript.
Additional information
Notes on contributors
Molla Asmare
Dr. Molla Asmare, an assistant professor of Energy Systems Engineering, at Bahir Dar Energy Center, Bahir Dar Institute of Technology, Bahir Dar University, Ethiopia, has a first degree in Chemistry an MSc in Sustainable Energy Engineering and a P.hD In Energy Systems Engineering. He has several published articles in renewable energy and environmental issues. He won the second prize in applied science and technology at the international students science congress of Turkey. He also worked as Energy experts at bureau level. His research interest is fuel cell, advanced energy storage and conversion, E-Mobility, hydrogen storage, bioenergy, solar energy, combustion and modelling.
References
- Akduman, O. Y., and A. M. Soydan. 2023. “A New Method for Manufacturing Anode Supported Solid Oxide Fuel Cells with AFL.” International Journal of Hydrogen Energy 51:1512–1522. https://doi.org/10.1016/j.ijhydene.2023.08.046.
- Appari, S., L. Maier, S. Tischer, S. Jayanti, O. Deutschmann, and V. M. Janardhanan. 2011. “Micro-kinetic modeling of NH3 decomposition on Ni and its application to solid oxide fuel cells.” Chemical Engineering Science 66 (21): 5184–5191. https://doi.org/10.1016/j.ces.2011.07.007.
- Asmare, M. 2022. “Investigating the Effects of Reactant Gas Flow Geometrical Shape on the Performance of Solid Oxide Fuel Cell.” International Journal of Sustainable Engineering 15 (1): 325–334. https://doi.org/10.1080/19397038.2022.2150936.
- Asmare Alemu, M., and M. Ilbas. 2020. “Direct Ammonia Powered Solid Oxide Fuel Cells: Challenges, Opportunities, And Future Outlooks.” Journal of Engineering and Applied Sciences Technology 2 (3): 1–11. https://doi.org/10.32438/ijet.203011.
- Asmare, M., and M. Ilbas. 2020. “Direct ammonia fueled solid oxide fuel cells: A comprehensive review on challenges, opportunities and future outlooks.” International Journal of Energy Technology 70–91. https://doi.org/10.32438/IJET.203011.
- Asmare, M., M. Ilbas, F. M. Cimen, C. Timurkutluk, and S. Onbilgin. 2022. “Three-dimensional numerical simulation and experimental validation on ammonia and hydrogen fueled micro tubular solid oxide fuel cell performance.” International Journal of Hydrogen Energy 47 (35): 15865–15874. https://doi.org/10.1016/j.ijhydene.2022.03.057.
- Asmare, M., M. Ilbas, and S. Yalcin. 2021. “Numerical modelling and comparative analysis of direct ammonia fuelled protonic and oxygen- ion conducting tubular solid oxide fuel cell.” International Journal of Hydrogen Energy 46 (74): 36878–36889. https://doi.org/10.1016/j.ijhydene.2021.08.230.
- Atkinson, A., S. Barnett, R. J. Gorte, J. T. S. S. Irvine, A. J. Mcevoy, M. Mogensen, S. C. Singhal, and J. Vohs. 2004. “Advanced Anodes for High-Temperature Fuel Cells.” Nature Materials 3 (1): 17–27. https://doi.org/10.1038/nmat1040.
- Cheddie, D. F. 2013. “Modelling of Ammonia-Fed Solid Oxide Fuel Cells.” Materials and Processes for Energy: Communicating Current Research and Technological Developments 504–511.
- Chen, K., X. Chen, Z. Lü, N. Ai, X. Huang, and W. Su. 2008. “Performance of an Anode-Supported SOFC with Anode Functional Layers.” Electrochimica Acta 53 (27): 7825–7830. https://doi.org/10.1016/j.electacta.2008.05.063.
- Chen, S., D. Gu, Y. Zheng, H. Chen, and L. Guo. 2020. “Enhanced Performance of NiO–3YSZ Planar Anode-Supported SOFC with an Anode Functional Layer.” Journal of Materials Science 55 (1): 88–98. https://doi.org/10.1007/s10853-019-04007-4.
- Chen, X., J. Lin, L. Sun, T. Liu, J. Wu, Z. Sheng, and Y. Wang. 2018. “Improvement of Output Performance of Solid Oxide Fuel Cell by Optimizing the Active Anode Functional Layer.” Electrochimica acta 298:112–120. https://doi.org/10.1016/j.electacta.2018.12.078.
- Chen, X., W. Ni, X. Du, Z. Sun, T. Zhu, Q. Zhong, and M. Han. 2019. “Electrochemical Property of Multi-Layer Anode Supported Solid Oxide Fuel Cell Fabricated Through Sequential Tape-Casting and Co-Firing.” Journal of Materials Science and Technology 35 (4): 695–701. https://doi.org/10.1016/j.jmst.2018.10.015.
- Chrzan, A., J. Karczewski, D. Szymczewska, and P. Jasinski. 2017. “Nanocrystalline Cathode Functional Layer for SOFC.” Electrochimica Acta 225:168–174. https://doi.org/10.1016/j.electacta.2016.12.128.
- Develos-Bagarinao, K., T. Ishiyama, H. Kishimoto, H. Shimada, and K. Yamaji. 2021. “Nanoengineering of Cathode Layers for Solid Oxide Fuel Cells to Achieve Superior Power Densities.” Nature Communications 12 (1): 1–12. https://doi.org/10.1038/s41467-021-24255-w.
- Etemadi, A., S. Ghorbani, M. Masoumpour, and M. Dadkhah. 2016. “The Numerical Analysis of an Anode-Supported High Temperature DIR- PSOFC Operating Conditions with Considering the Maximum Allowable Temperature Difference.” Journal of Heat and Mass Transfer Research 1 (2): 15–25.
- Ferguson, R., M. Fiard, and R. Herbin. 1996. “Three-Dimensional Numerical Simulation for Various Geometries of Solid Oxide Fuel Cells.” Journal of Power Sources 58 (2): 109–122. https://doi.org/10.1016/0378-7753(95)02269-4.
- Gross, M. D., J. M. Vohs, and R. J. Gorte. 2007. “An Examination of SOFC Anode Functional Layers Based on Ceria in YSZ.” Journal of the Electrochemical Society 154 (7): B694. https://doi.org/10.1149/1.2736647.
- Hassan, A. A. E., N. H. Menzler, G. Blass, M. E. Ali, H. P. Buchkremer, and D. Stöver. 2002. “Development of an Optimized Anode Functional Layer for Solid Oxide Fuel Cell Applications.” Advanced Engineering Materials 4 (3): 125–129. https://doi.org/10.1002/1527-2648(200203)4:3<125:AID-ADEM125>3.0.CO;2-D.
- Hong, S. A., and W. N. Suk. 2006. “Application of Sol-Gel Techniques in Fabrication of Fuel Cells.” Studies in Surface Science and Catalysis 159:79–84. https://doi.org/10.1016/S0167-2991(06)81541-7.
- Ilbas, M., B. Kumuk, M. A. Alemu, and B. Arslan. 2020. “Numerical investigation of a direct ammonia tubular solid oxide fuel cell in comparison with hydrogen.” International Journal of Hydrogen Energy 45 (60): 35108–35117. https://doi.org/10.1016/j.ijhydene.2020.04.060.
- Ilbas, M., B. Kumuk, M. A. M. A. Alemu, and B. Arslan. 2020. “Numerical investigation of a direct ammonia tubular solid oxide fuel cell in comparison with hydrogen.” International Journal of Hydrogen Energy 45 (60): 35108–35117. https://doi.org/10.1016/j.ijhydene.2020.04.060.
- Jiang, W., R. Fang, J. A. Khan, and R. A. Dougal. 2006. “Parameter Setting and Analysis of a Dynamic Tubular SOFC Model.” Journal of Power Sources 162 (1): 316–326. https://doi.org/10.1016/j.jpowsour.2006.06.086.
- Kagomiya, I., S. Kaneko, K. Kakimoto, K. Park, and K. H. Cho. 2015. “Fabrication and Power Densities of Anode-Supported Solid Oxide Fuel Cells with Different Ni-YSZ Anode Functional Layer Thicknesses.” Fuel Cells 15 (1): 90–97. https://doi.org/10.1002/fuce.201400138.
- Kagomiya, I., S. Kaneko, Y. Yagi, K. I. Kakimoto, K. Park, and K. H. Cho. 2017. “Dependence of Power Density on Anode Functional Layer Thickness in Anode-Supported Solid Oxide Fuel Cells.” Ionics 23 (2): 427–433. https://doi.org/10.1007/s11581-016-1860-5.
- Kakaç, S., A. Pramuanjaroenkij, and X. Y. Zhou. 2007. “A Review of Numerical Modeling of Solid Oxide Fuel Cells.” International Journal of Hydrogen Energy 32 (7): 761–786. https://doi.org/10.1016/j.ijhydene.2006.11.028.
- Kenney, B., and K. Karan. 2007. “Engineering of Microstructure and Design of a Planar Porous Composite SOFC Cathode: A Numerical Analysis.” Solid State Ionics 178 (3–4): 297–306. https://doi.org/10.1016/j.ssi.2006.12.009.
- Konno, A., H. Iwai, M. Saito, and H. Yoshida. 2012. “Effect of Characteristic Lengths of Electron, Ion, and Gas Diffusion on Electrode Performance and Electrochemical Reaction Area in a Solid Oxide Fuel Cell.” Heat Transfer - Asian Research 41 (8): 700–718. https://doi.org/10.1002/htj.20373.
- Kumuk, B., M. Asmare Alemu, and M. Ilbas. 2022. “Investigation of the Effect of Ion Transition Type on Performance in Solid Oxide Fuel Cells Fueled Hydrogen and Coal Gas.” International Journal of Hydrogen Energy 47 (5): 3409–3415. https://doi.org/10.1016/j.ijhydene.2021.10.212.
- Mench, M. M. 2008. “Fuel Cell Engines.” Fuel Cell Engines. https://doi.org/10.1002/9780470209769.
- Molin, S., A. Chrzan, J. Karczewski, D. Szymczewska, and P. Jasinski. 2016. “The Role of Thin Functional Layers in Solid Oxide Fuel Cells.” Electrochimica Acta 204:136–145. https://doi.org/10.1016/j.electacta.2016.04.075.
- Molla, A., and M. Ilbas. 2020. “Direct ammonia fueled solid oxide fuel cells: A comprehensive review on challenges, opportunities and future outlooks.” International Journal of Energy Technology 2 (June): 70–91. https://doi.org/10.32438/IJET.203011.
- Park, J. M., D. Y. Kim, J. D. Baek, Y. J. Yoon, P. C. Su, and S. H. Lee. 2018. “Effect of Electrolyte Thickness on Electrochemical Reactions and Thermo-Fluidic Characteristics Inside a SOFC Unit Cell.” Energies 11 (3): 473. https://doi.org/10.3390/en11030473.
- Shen, Q., S. Li, G. Yang, N. Huang, J. Yuan, and B. Sundén. 2019. “Analysis of Heat and Mass Transport Characteristics in Anode-Supported Solid Oxide Fuel Cells at Various Operating Conditions.” Numerical Heat Transfer 75 (8): 509–522. https://doi.org/10.1080/10407782.2019.1608773.
- Shipilova, A. V., A. A. Solov’ev, E. A. Smolyanskii, S. V. Rabotkin, and I. V. Ionov. 2021. “The Effect of Thin Functional Electrode Layers on Characteristics of Intermediate Temperature Solid Oxide Fuel Cell.” Russian Journal of Electrochemistry 57 (2): 97–103. https://doi.org/10.1134/S1023193521020038.
- Singhal S. C. 2000. “Advances in solid oxide fuel cell technology.” Solid State Ionics 135 (1–4): 305–313. https://doi.org/10.1016/S0167-2738(00)00452-5.
- Singhal, S. C. 2002. “Solid Oxide Fuel Cells for Stationary, Mobile, and Military Applications.” Solid State Ionics 152–153:405–410. https://doi.org/10.1016/S0167-2738(02)00349-1.
- Su, S., X. Gao, Q. Zhang, W. Kong, and D. Chen. 2015. “Anode-Versus Cathode-Supported Solid Oxide Fuel Cell: Effect of Cell Design on the Stack Performance.” International Journal of Electrochemical Science 10 (3): 2487–2503. https://doi.org/10.1016/S1452-3981(23)04863-0.
- Takahashi, K., H. Fujita, Y. Ishikawa, and T. Nakagaki. 2021. “Microfabrication of Anode Functional Layer in SOFC by 3D Printer.” MATEC Web of Conferences 333:17001. https://doi.org/10.1051/matecconf/202133317001.
- Vafaeenezhad, S., A. R. Hanifi, M. A. Laguna-Bercero, T. H. Etsell, and P. Sarkar. 2022. “Microstructure and Long-Term Stability of Ni–YSZ Anode Supported Fuel Cells: A Review.” Materials Futures 1 (4): 042101. https://doi.org/10.1088/2752-5724/ac88e7.
- Walther, D. C., and J. Ahn. 2011. “Advances and Challenges in the Development of Power-Generation Systems at Small Scales.” Progress in Energy and Combustion Science 37 (5): 583–610. https://doi.org/10.1016/j.pecs.2010.12.002.
- Xu, H., B. Chen, P. Tan, W. Cai, W. He, D. Farrusseng, and M. Ni. 2018. “Modeling of all porous solid oxide fuel cells.” Applied Energy 219 (March): 105–113. https://doi.org/10.1016/j.apenergy.2018.03.037.