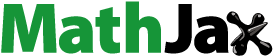
ABSTRACT
Scientific advances and technological requirements to develop carbon fibre-reinforced polymers (CFRP) with excellent strength-to-weight ratios led to the high consumption of CFRP composites. The mechanical recycling of CFRP is a simpler, more economical, and environmentally responsible solution for effectively recovering this structural material that contains epoxy resin and carbon fibre. CFRP laminates were placed on a Computer Numerical Control (CNC) and milling cutting at 1100, 1800, and 2500 rpm. The recovered CFRP particles were mixed with acrylonitrile butadiene styrene (ABS) using the melt intercalation approach. Recovered CFRP particles increased the molecular mobility and reduced the thermal stability of ABS. The main differences between the ABS and the composites were a more pronounced necking region in the ABS than in the composites and a notable reduction in strain. The strain of the ABS was 27.58%, while in the composites, it was 4.29, 4.02, and 3.51%, depending on the cutting speed. Thus, ductility decreased up to 87% in the composites. This work’s successful CFRP mechanical recycling method provides epoxy powder, individual carbon fibres, and CFRP particles, opening a research field of great economic and environmental relevance in developing new materials.
1. Introduction
It is well known that carbon fibres have a specific stiffness and resistance higher than that of steel or various metal alloys (van de Werken et al. Citation2019). Carbon fibre fabrics are impregnated with thermosetting resins, generally epoxy, to manufacture carbon fibre reinforced polymer (CFRP) composites, which have an excellent strength-to-weight ratio and are recognised for their multifunctional capacity and flexibility in the design (Rodríguez-González et al. Citation2024)). Due to the mentioned properties, the aerospace, transportation, sports, and infrastructure sectors demand a large amount of CFRP annually (Rani et al. Citation2021). All these components and its high consumption eventually lead to more plastic garbage, which forces researchers to work on their waste management and handling problems. Landfilling results in various groundwater problems, and incinerators release a significant amount of CO2 into the atmosphere. However, the energy used in the recycling of carbon fibres (CFs) is typically 96% less than that used in the production of virgin CFs (Khalid et al. Citation2022).
Despite the multiple structural advantages CFRP offers, it is necessary to highlight that these materials also have certain disadvantages. The conversion of polyacrylonitrile (PAN), as a precursor to carbon fibre, requires a very high amount of energy for its manufacture since it must go through oxidation, carbonisation, and graphitisation processes, considerably increasing its cost. For their part, thermosetting resins have the disadvantage of forming cross-linked structures during their curing process, so they cannot be melted and reprocessed, which is why CFRP composites cannot be recycled (Witik et al. Citation2013).
The growing global concern for sustainability and environmental conservation has driven significant research efforts towards developing innovative strategies for waste management and resource utilisation (Burelo et al. Citation2024; Kim et al. Citation2022; Manuel et al. Citation2023). The recycling and reuse of CFRP composites, whose global waste is expected to increase 20 kilotons annually by 2025, are essential for reusing these expensive, high-consumption wastes with high mechanical performance in development (Zhang et al. Citation2020).
On the one hand, the constituents (carbon fibre and epoxy resin) are expensive, but so are the various manufacturing processes used to make CFRP components. Suppose these components are rejected or end their functional usefulness, also known as end of life (EoL). In that case, they are sent to landfills or subjected to pyrolysis techniques or chemical solvents, eliminating the thermosetting resin (which has a high thermomechanical potential) and only recycling carbon fibre. However, both processes are expensive, polluting and damaging the structure of the carbon fibre, considerably reducing its mechanical properties (Caltagirone et al. Citation2021).
An alternative is to use mechanical recycling processes to recover CFRP (rCFRP) composites, including carbon fibre and epoxy resin, as reinforcements to improve thermoplastic polymers’ mechanical and thermal performance. The size reduction of the composite scrap is often accomplished by mechanical recycling procedures using various milling, grinding, and crushing equipment. Because the chopped recycled materials acquired through mechanical recycling have diminished the mechanical characteristics of rCFRP, they can be utilised as fillers or reinforcements in the creation of new composites (Arif et al. Citation2022; Khalid et al. Citation2021, Citation2023). Acrylonitrile butadiene styrene (ABS) is a thermoplastic polymer known for its low density and hardness (Kornilitsina et al. Citation2023). It is widely used in manufacturing pipes, automotive components, protective helmets, computer parts, personal care products, additive manufacturing, and toys (Alonso et al. Citation2023; Moreno-Núñez et al. Citation2023). ABS has exceptional chemical resistance, strong mechanical properties, recyclability, and ease of processing (Boğa Citation2021). Extensive research has documented the use of organic and inorganic particles in ABS matrix to achieve mechanical advantages, including carbon nanotubes (CNT), carbon fibre (CF), and mineral clays (Alghadi, Tirkes, and Tayfun Citation2020; Franco-Urquiza Citation2021; Franco-Urquiza et al. Citation2015, Citation2020; Valino et al. Citation2019; Wei et al. Citation2018). However, the effect of rCFRP particles as mechanical reinforcements in ABS is not documented, and that is the gap that this work aims to cover.
This work aims to determine the thermal and mechanical properties of ABS composites reinforced with rCFRP particles obtained from a mechanical recycling process by varying the cutting speed. The results will open a field of research around the mechanical recycling of CFRP composites, which is expected to be considered of great economic and environmental relevance in the next decade.
2. Materials and methods
2.1. Materials
A 3K carbon fibre fabric (3000 filaments per fibre) from Quintum was used to manufacture the CFRP composites. The fabric was a 1 × 1 plain weave weighing 198 g/m2, with a tensile strength of 2137.37 MPa and a modulus of elasticity of 227 GPa. The epoxy resin Epolam 2015 from Sika was used as the polymer matrix. According to the manufacturer’s technical datasheet, Epolam 2015 has a viscosity of 1.55 mPa.s and a density of 1.15 g/cc. Epolam 2015 Hardener, with amine groups, was used as a catalyst at a mixing ratio of 32 by weight with a useful life of 140 min at room temperature (25°C).
ABS Terluran® HI-10, supplied by BASF, was used as a polymer matrix. According to the technical datasheet, the ABS has a melt volume-flow rate of 5.5 cm3/10 min (220°C/10 kg), tensile strength of 38 MPa, and strain at break of 9%.
2.2. Methods
2.2.1. CFRP laminate manufacturing
Carbon fibre and epoxy resin laminates were manufactured in a controlled environment, which allowed the reproducibility of the process, avoiding significant variations that could alter the results of the experimentation. Thus, the CFRP laminates were prepared using the vacuum-assisted resin infusion (VARI) method. The vacuum pressure was −20 inHg, and the resin curing reaction took approximately 24 hours (~25°C). The orientation of the carbon fibre fabrics was quasi-isotropic, with a stacking sequence of The CFRP laminates had 18 layers of CF plain weave fabric and nominal dimensions of 300 mm x 300 mm x 4 mm. Two materials with different applications were placed on top of the substrate: A peel-ply fabric and a distribution mesh were placed to distribute the resin homogeneously. Finally, the entire laminate was covered with a vacuum bag sealed with butyl tape for optimal vacuum during the impregnation and curing process (). More information about composite manufacturing can be found in a previous research article (Franco-Urquiza and Rentería-Rodríguez Citation2023).
2.2.2. CFRP mechanical recycling
The laminates were cut into three 90 mm x 250 mm sections, marked 1, 2, and 3 in . The sections were numbered consecutively (4, 5, 6) to control the experiment adequately (). In each section, 12 cutting zones of 20 mm were drawn, as presented in . The sections were arranged horizontally on a Challenger CNC, model MM-430, and were firmly clamped to prevent vibrations or slipping during cutting.
Figure 2. Photographs corresponding to: (a) CFRP laminate sections and (b) cutting zones.

Carbide end mill cutters of 10 mm diameter, four flutes, and without coating were used. One cutter was used for each section, making the cut in the lateral part of the laminate and advancing through cutting zones, as presented in . In this way, the number of cutting zones and the amount of rCFRP collected can be measured as a function of the cutting speed, which varied from 1100 rpm, 1800 rpm, and 2500 rpm.
Figure 3. Mechanical recycling of CFRP laminates: (a) lateral cutting process and (b) milling of cutting zones.

The rCFRP was separated on a Ro-Tap laboratory sieve equipped with W.S. sieves. Stainless steel mesh Tyler between 1700 and 90 microns.
2.2.3. Extrusion process
The ABS/rCFRP composites containing a nominal percentage weight of 3% of rCFRP were melt blended in a single-screw extruder (L/D = 30, D = 19 mm) Beutelspacher model SB. The screw rotation speed was set at 5.5 Hz, and according to the processing recommendations datasheet, the suitable temperature profile was 180°C (feed section), 210, 200°C, and 190°C at the extrusion die. Two steps of a single extrusion process were required to homogenise the ABS/rCFRP composites, labelled as ABS/rCFRP-1100, ABS/rCFRP-1800, and ABS/rCFRP-2500, as referred to the cutting speed.
2.2.4. Injection molding
Dumbbell-like specimens (ASTM D638 type V) of ABS and ABS/rCFRP composites were injection moulded in a BabyPlast 6/12 microinjection machine. The temperature profile was 263°C in the plasticising zone, 254°C y 247°C, with a closed-mould pressure of 7.5 MPa. After injection, specimens were stored and kept in a desiccator with silica for further characterisation.
It is relevant to underline that before extrusion processes and injection moulding, the ABS and the ABS/rCFRP composites were dried in a drying oven Thermo Scientific Precision at 80°C for 24 h.
2.2.5. Thermogravimetric analysis
The thermal stability of ABS and ABS/rCFRP composites was evaluated by thermogravimetric analysis (TGA) using a TGA 55 analyser from TA Instruments. The TGA test was performed from 50°C to 800°C at a heating rate of 10°C/min. The purge of each of the samples was 20 ml/min using nitrogen gas supplied at 45 psi. Samples weighing approximately 20 mg were placed in an alumina crucible. It is necessary to emphasise that both ABS and carbon fibre composites are organic materials, so the TGA was carried out only under a nitrogen atmosphere to identify the starting temperature of degradation from a 1% weight loss and the decomposition temperatures of the organic components.
2.2.6. Ash content
The rCFRP content of the composites was determined following a standard method of ash content in plastics according to the ASTM D5630. Clean crucibles were weighed, recording the resulting value as . Five grams of the composite pellets were placed in the cleaned crucible, and the resulting values were recorded as
. Then, the crucible was placed on a burner for 1 h. The crucible was removed from the burner and placed in a muffle, Tomas Scientific Thermolyne F30420C-TS, at 900°C for 1 h. The residues were cooled in a desiccator until they reached room temperature. The weight was reported as
, and the ash content was calculated using Equation (1):
2.2.7. Differential scanning calorimetry
The thermal parameters were obtained in a Differential Scanning Calorimetry (DSC) 250 TA Instruments. DSC was verified using standard samples of Indium. DSC samples of ~10 mg in weight were taken from the extensometer marks of the dumbbell-like injected specimens and placed in aluminium pans. The first heating scan was from 25 to 180°C with an isothermal step of 2 min, followed by a cooling scan with an isothermal step of 2 min and a second heating scan. The tests were conducted at a heating/cooling rate of 5 °C/min under a nitrogen atmosphere.
2.2.8. Mechanical test
The mechanical characterisation of ABS and ABS/rCFRP composites was performed in a universal testing machine, Instron 647, equipped with a load cell of 10 kN. Ten tensile specimens were tested at a 10 mm/min crosshead rate at room temperature (23 ± 2°C). Young’s modulus (E) and yield strength (σy) were calculated from the engineering stress vs. strain curves, and the nominal strain (εt) was determined using method A, according to the ISO-527 (ISO Citation2019).
2.2.9. Scanning electron microscope
The failure surface of post-mortem tensile specimens was observed in a JEOL JSM 6610LV scanning electron microscope (SEM) at 10kV and high vacuum. Samples were metallised with gold via sputtering. Magnifications ranging from 500× and 1000× were used as indicated in each image.
3. Results and discussion
3.1. CFRP mechanical recycling
presents the rCFRP obtained for each of the three cutting speeds. The cut zones drawn with 20 mm length allow identifying the lateral advance of the cut to obtain rCFRP particles, as can be seen in .
The tool wears out quickly by increasing the cutting speed, so its effectiveness is decreased, which is clearly seen by reducing the cutting zones (). The above has an impact on the amount of rCFRP obtained. At 1100 rpm, 91 g of rCFRP are obtained, which decreases almost 50% when the cutting speed increases to 2500 rpm. By increasing the cutting speed, premature wear of the carbide coating occurs, promoted by the high friction between the flutes of the end milling cutter and the highly abrasive carbon fibre composites. In this way, reducing the cutting speed reduces friction, and a greater amount of rCFRP is obtained with time-consuming. The above opens the window of opportunities to experiment with advanced coatings on cutting tools.
The CFRP mechanical recycling process was stopped when it was identified that the cutter was delaminating long carbon fibre, resulting in no dust. At the end of the cutting process, the dust was collected, stored in a plastic bag, and placed in a desiccator to avoid humidity and external contamination.
Subsequently, the obtained rCFRP was sieved to separate relatively fine particles from coarse ones. Thus, particles of 1700 and 425 microns were obtained in the first sieve. Following, particle sizes between 425 and 90 microns were obtained in a second sieving. rCFRP particles larger than 1700 microns were not used. Similarly, particles smaller than 90 microns could not be processed because they adhered to the screw channels. In this work, rCFRP particles with a size between 1700 and 425 microns were used.
3.2. Thermogravimetric analysis
The thermogravimetric analysis allows the evaluation of the effects of residue content on the thermal stability of ABS/rCFRP composites. shows the TGA curves corresponding to ABS and ABS/rCFRP composites.
Figure 4. TGA curves corresponding to the neat ABS and ABS/rCFRP composites.

The ABS and the composites presented a similar behaviour, except for ABS/rCFRP-1100. The degradation process (1% weight decrease) in ABS occurred at 290°C, while for the ABS/rCFRP-1100, ABS/rCFRP-1800, and ABS/rCFRP-2500 composites, it occurred at 245°C, 275°C, and 284 °C, respectively. Degradation ended at approximately 517 °C for ABS and composites. A second degradation stage can be seen at around 620°C, ending at 750°C. In the case of the ABS/rCFRP-1100 composite, this second degradation started at 562°C and ended at around 693°C. In all cases, the second degradation would indicate the decomposition of residual organic material.
The results would indicate that the ABS/rCFRP composites are thermally unstable due to rCFRP particles, including epoxy resin and carbon fibre. At the moment, the recycling process carried out in this work prevents the effective separation of the constituents; hence, some composites would contain a greater amount of carbon fibre, others a greater amount of epoxy resin, and others a greater amount of rCFRP particles. The previous causes the TGA analysis to present variations in the results. Carbon fibre has a much higher thermal conductivity capacity than thermoplastics and thermosets (epoxy resin). In this way, the energy supplied to the composite samples and a smaller amount of ABS favours molecular mobility, so the first degradation process occurs at lower temperatures than ABS. Similarly, in the second degradation, the rCFRP residues would promote thermal energy dissipation, making the composite thermally unstable and more pronounced in the composite containing residue particles obtained at lower shear rates.
The ash content test was carried out to determine the inorganic residue content using a greater amount of material than required by the TGA (). In this way, the content of inorganic residue in the ABS/rCFRP composites could be compared, revealing whether the two single-screw extrusion steps allowed homogeneous mixing. presents the ash content values obtained for the ABS and the composites.
Figure 5. Photographs corresponding to the ash content test residues: (a) ABS/rCFRP-1100, (b) ABS/rCFRP-1800, and (c) ABS/rCFRP-2500.

Table 1. Ash content and mechanical parameters of neat ABS and ABS/rCFRP composites.
The standard deviation values were insignificant, so they are not presented in the results. It can be seen that the composites contain a meagre percentage of inorganic residue, which could be part of the carbon fibre or epoxy resin. In any case, the ash content is very similar in the composites, so it can be deduced that the two single-screw extrusion steps used allow a homogeneous mixing at a low weight percentage of rCFRP.
3.3. Differential scanning calorimetry analysis
shows the DSC endotherms of ABS and ABS/rCFRP composites corresponding to the second heating.
Figure 6. DSC endotherm of ABS and ABS/rCFRP composites. The dotted line refers to the onset, and the arrows point to the endset.

ABS is an amorphous material, so DSC tests were carried out to understand the composites’ glass transition temperature (Tg) behaviour. Both ABS and ABS/rCFRP composites showed a similar onset, indicated by the dashed line in . However, the endset of the composites showed a slight shift towards lower temperatures concerning the Tg of net ABS.
Contrary to what was expected, where a rigid filler should hinder the molecular movement of the polymer chains by increasing the Tg of the composite, the addition of rCFRP to the ABS matrix seems to have a slight impact on the molecular mobility. The above has been attributed to the effect produced by carbon fibres’ conductive capacity, enhancing the polymer chains’ thermal mobility, and reducing the composites’ Tg (Kazi et al. Citation2019).
3.4. Mechanical test
shows the representative stress vs. strain curves of ABS and ABS/rCFRP composites, and the failure of the dumbbell-like specimens (ASTM D638 type V) of ABS/rCFRP composites. The materials presented a similar mechanical behaviour, with an elastic region followed by a drop in tension and strain at a practically constant tension level until the failure of the specimen.
Figure 7. Stress vs. strain curves of: a) neat ABS, (a) neat ABS, (b) ABS/rCFRP composites, (c) post-mortem tensile specimens.

The main differences between the ABS and the composites were a much more pronounced necking region in the ABS than in the composites and a notable reduction in strain. In ABS/rCFRP composites, the mechanical behaviour is practically the same. Some differences can be seen in , such as the reduction in strain as rCFRP particles obtained at high cutting speeds are used. The rCFRP particles prevent necking during material yielding, so the stress drop is much less evident than in neat ABS (Maspoch et al. Citation2009). In addition, the particles prevent the strain of the chains, considerably reducing their stretching capacity. presents the mechanical parameters determined from the stress vs strain curves.
The stiffness of ABS does not present significant changes with the presence of rCFRP particles. The tensile strength of the composites is lower than that of ABS, but there are no significant variations concerning the particles obtained by different cutting speeds, so the rCFRP particles are likely acting as a filler and not as a reinforcement. The ABS strain decreases notably in the composites. The strain is the mechanical parameter that showed a trend concerning the rCFRP particles obtained by different cutting speeds. In this way, the particles obtained at a higher cutting speed decrease the stretchability of the ABS/rCFRP composites, from 84.5% for 1100 rpm to 87.2% for 2500 rpm.
As detailed in the materials section, carbon fibre and epoxy resin have mechanical properties much higher than ABS. The absence of molecular compatibility between the constituents of the rCFRP particles and the ABS polymer chains would promote the low mechanical performance presented by the composites. This lack of compatibility means that the rCFRP particles are ineffective in stress transmission and act as stress concentrators that accelerate the premature failure of the compounds.
3.5. Scanning electron microscope analysis
shows the SEM micrographs corresponding to the failure zone of the ABS and the composites. ABS shows a smooth, tear-free failure surface, typical of a neat polymer (). On the contrary, the failure surfaces of the composite specimens revealed tearing and cavitations ().
Figure 8. SEM micrographs of the failure surface of: a) neat ABS, (a) neat ABS, (b) ABS/rCFRP-1100, (c) ABS/rCFRP-1800, and (d) ABS/rCFRP-2500.
At high magnifications, the micrographs of the failure surfaces of the ABS/rCFRP composites reveal two different zones since there are areas where the individual carbon fibres can be observed. However, there are also areas with evident agglomerations related to epoxy resin and rCFRP particles, as presented in .
Figure 9. High magnifications of SEM micrographs concerning the failure surface of: (a) neat ABS, (b) ABS/rCFRP-1100, (c) ABS/rCFRP-1800, and (d) ABS/rCFRP-2500. The composite surfaces show carbon fiber and agglomerated epoxy resin. Orange arrows point to individual carbon fibers; green arrows point to epoxy resin agglomerates; orange dashed rectangles frame rCFRP particles.

Therefore, the failure of the composites could occur due to the combination of defects during the mechanical tension test. On the one hand, the individual carbon fibres are found in the ABS matrix, but it is unclear whether the fibres are embedded in the ABS (). Smooth cavitations indicate the detachment of the fibres from the ABS matrix, demonstrating low adhesion between the ABS and the carbon fibres, which reduces the strength of the ABS/rCFRP composites.
Figure 10. SEM micrographs that show the combination defects on the failure surfaces of ABS/rCFRP composites: (a) low adherence of single carbon fibers, (b) epoxy resin agglomerated, and (c) rCFRP particles. Orange arrows point to individual carbon fibers and voids; green dashed rectangle highlights epoxy resin agglomerates; orange dashed rectangle frames rCFRP particles.

On the other hand, the epoxy resin particles seem to be agglomerated within the ABS matrix (), and the rCFRP particles remain tightly bound, demonstrating that the shear of single-screw extrusion is not high enough to separate these particles (). In both cases, the agglomerated epoxy resin and the rCFRP particles would act as stress concentrators that accelerate the premature failure of the specimen during the tensile tests.
4. Conclusions
Mechanical recycling from the use of commercial cutters is feasible. The rCFRP particles consisted of epoxy resin powder, individual carbon fibres, and rCFRP particles, which served as thermoplastic ABS reinforcement. This work obtained CFRP particles in a range of 1700 and 425 microns.
High cutting speeds promote premature wear of the carbide coating of the end milling cutters, and consequently, a lower amount of rCFRP particles is obtained. Low cutting speeds consume time.
The cutting speed does not seem to influence the quality of the rCFRP particles since no significant variations are observed in the thermal and mechanical properties of the compounds.
The cutting speed of the rCFRP particles does not significantly influence the thermal properties of ABS composites. The introduction of rCFRP reduced the thermal stability of ABS, and the thermal conductivity of carbon fibre increases the molecular movement of the polymer chains, reducing the Tg of ABS.
ABS/rCFRP composites fail mechanically due to the combined effects produced by mechanical recycling. On the one hand, the poor adhesion between the individual carbon fibres restricts the rigidity of the ABS, while the agglomeration of the epoxy resin and the presence of rCFRP particles act as stress concentrators, reducing the strain of the ABS composites. Therefore, coupling agents are required to improve the affinity between the ABS matrix and the rCFRP particles to increase the thermal and mechanical properties.
The variations found in the thermal and mechanical properties of ABS/rCFRP composites could be attributed to the heterogeneous separation of the reinforcement constituents (epoxy resin, carbon fibre, and rCFRP particles), the amount of these constituents present in the composite, and the agglomeration of the particles.
It is necessary to emphasise the high cost of developing CFRP parts and components for the industry and the severe environmental damage they cause. This work is the first in a series of research articles related to the mechanical recycling of CFRP composites, which is feasible for reusing costly materials with relevant engineering properties to develop new products.
Author contributions
Conceptualisation, E.A.F.-U., C.Z.-P. and V.A.G.-C.; methodology, C.V.-L., C.Z.-P. and V.A.G.-C; validation, C.V.-L., C.Z.-P., M.B., E.A.F.-U and C.D.T.-Q.; formal analysis, C.V.-L., C.Z.-P. and E.A.F.-U; investigation, C.V.-L., C.Z.-P. and V.A.G.-C; resources, E.A.F.-U and C.D.T.-Q.; data curation, C.V.-L., C.Z.-P. and V.A.G.-C; writing – original draft preparation, C.V.-L., C.Z.-P., E.A.F.-U and C.D.T.-Q.; writing – review and editing, C.V.-L., C.Z.-P., M.B, E.A.F.-U and C.D.T.-Q.; supervision, C.Z.-P., M.B, E.A.F.-U and C.D.T.-Q.; project administration E.A.F.-U and C.D.T.-Q.; funding acquisition, E.A.F.-U and C.D.T.-Q. All authors have read and agreed to the published version of the manuscript.
Acknowledgments
The authors would like to acknowledge the support of Tecnológico de Monterrey, and Consejo Nacional de Humanidades Ciencias y Tecnologías (CONAHCyT). Additionally, they want to acknowledge the technical support provided by Centro de Ingenieria y Desarrollo Industrial (CIDESI) Querétaro for the support in the experiments.
Disclosure statement
No potential conflict of interest was reported by the author(s).
Data availability statement
The data presented in this study are available on request from the corresponding author.
Additional information
Funding
Notes on contributors
Carolina Vega-Leal
Carolina Vega-Leal is an Innovation and Development Engineer graduated from the Monterrey Institute of Technology and Higher Education (ITESM) Campus Querétaro. She is currently studying a Master’s degree in Science Engineer at the Monterrey Institute of Technology and Higher Education. She has participated and won second place at the 4th International Congress of Chemistry, Energy and Environmental Sustainability “Dr. Mario Molina Henriquez”. Among its main interests are the automotive industry and the aeronautical industry. Her dream is to add value to the recycling of end-of-life composite materials used in Formula 1.
Cecilia Zárate-Pérez
Cecilia Zárate Pérez Nanotechnology Engineer from the Technological University of Querétaro (UTEQ) of the state of Querétaro Mexico with a Master’s Degree in Science and Technology with a specialty in Design and Development of Mechanical Systems from the Center for Industrial Engineering and Development (CIDESI) of the same state. She has participated in student mobility to the Ivy Tech Community College in Lafayette, Indiana, United States and to the Madrid Institute of Advanced Studies in Getafe, Spain. She is currently part of the advanced polymers and biopolymers research group at CIDESI. She is an accredited test analyst specialized in materials characterization as well as training oriented in extrusion material processing. Her main interests are focused on the circular economy and the reincorporation of polymers into value chains.
Victor A. Gomez-Culebro
Victor A. Gomez-Culebro is a Mechanical Engineer from the Technological Institute of Tuxtla Gutiérrez (ITTG) of the State of Chiapas. M.C and T Víctor Gómez Culebro has a Specialty in Mechatronics Technologist and a Master’s Degree in Science and Technology from the Center for Industrial Engineering and Development (CIDESI) in the city of Santiago de Querétaro. He currently works as a Project Engineer at CIDESI in the composite materials laboratory in the vacuum assisted infusion (VARI), resin transfer molding (RTM) and AUTOCLAVE manufacturing processes. He has worked on projects such as: Torque Shaft for applications in turbines, CubeSat type nanosatellites, Bio-laminates, and aircraft interior research project.
Manuel Burelo
Manuel Burelo received his Bachelor’s degree in Chemical Engineering from Universidad Juárez Autónoma de Tabasco, México. A master’s degree in Materials Sciences and Engineering and Doctor’s degree in Chemical Sciences from Universidad Nacional Autónoma de México, UNAM. He received the Santander University Scholarship for young professors and researchers in 2018, the Agilent Technologies 2019 scholarship for developing research techniques, and the award for the best doctoral thesis in polymer science and engineering by the Mexican Polymeric Society (SPM) in 2020. He has published 13 articles in indexed journals (h-index =7). He has the Mexican Researcher Certification (SNI) from CONAHCYT, Mexico. Dr. Burelo is currently a postdoctoral researcher at the Institute of Advanced Materials for Sustainable Manufacturing at the Tecnológico de Monterrey, Queretaro, Mexico. His research lines are synthesis and characterization of bio-based polymers, polymer degradation, recycling, and sustainable chemistry in polymers and materials.
E. A. Franco-Urquiza
E. A. Franco-Urquiza is an aeronautical engineer and Ph.D. in polymers from the Polytechnic University of Catalonia (UPC) in Barcelona, Spain. Dr. Franco worked as a UPC research professor from 2005 to 2014. He has two Postdoctoral stays at the University of Toronto Institute for Aerospace Studies (UTIAS) in Canada. He is a member of the SNI level 2, has published over 50 indexed articles, and supervised 10 postgraduate theses. He leads the “advanced polymers and bio composites” research group at CIDESI, contributing to developing and acquiring infrastructure to attract projects in polymers and composites in coordination with academia, industry, and government. His main academic interests are the reuse of polymer composites and the development of intelligent polymers.
Cecilia D. Treviño-Quintanilla
Cecilia D. Treviño-Quintanilla earned her B.S in Chemical Engineering from the Tecnológico de Monterrey in Monterrey. Dr. Treviño starts her graduate studies in the Tecnológico de Monterrey. She had to have a research stay at Rice University in Houston, Tx., where she was working on research in material science and the latest on nanotechnology. As part of her PhD studies, she stays at the University of Houston where she was trained in Materials Science. At the same time, she was working as an intern in the Research Center of Total Petrochemicals & Refining USA. Currently Dr. Treviño works as a full-time researcher of the Institute of Advanced Materials and Sustainable Manufacturing of the Tecnológico de Monterrey as Core Researcher of the Advanced Polymers Research Unit. With different projects on additive manufacturing of composites materials, biodegradable composites, thermal modeling, and degradation of polymers. Dr. Treviño is a member of the National System of Researchers (SNI). She has collaboration with different research centers in Mexico as CIDESI and CINVESTAV. She has conducted research with the sponsorship of Total Petrochemicals and Refining USA. She is working in collaboration with the Southwest Research Institute on the chemical degradation of industrial rubbers.
References
- Alghadi, A. M., S. Tirkes, and U. Tayfun. 2020. “Mechanical, Thermo-Mechanical and Morphological Characterization of ABS Based Composites Loaded with Perlite Mineral.” Materials Research Express 7:015301. https://doi.org/10.1088/2053-1591/ab551b.
- Alonso, A., M. Lázaro, D. Lázaro, and D. Alvear. 2023. “Thermal Characterization of Acrylonitrile Butadiene Styrene-ABS Obtained with Different Manufacturing Processes.” Journal of Thermal Analysis and Calorimetry 148:10557–10572. https://doi.org/10.1007/s10973-023-12258-2.
- Arif, Z. U., M. Yasir Khalid, W. Ahmed, H. Arshad, and S. Ullah. 2022. “Recycling of the Glass/Carbon Fibre Reinforced Polymer Composites: A Step Towards the Circular Economy.” Polymer-Plastics Technology & Materials 61 (7): 761–788. https://doi.org/10.1080/25740881.2021.2015781.
- Boğa, C. 2021. “Investigation of Mechanical and Fracture Behavior of Pure and Carbon Fiber Reinforced ABS Samples Processed by Fused Filament Fabrication Process.” Rapid Prototyping Journal 27 (6): 1220–1229. https://doi.org/10.1108/RPJ-11-2020-0296.
- Burelo, M., A. Martínez, J. David Hernández-Varela, T. Stringer, M. Ramírez-Melgarejo, A. Y. Yau, G. Luna-Bárcenas, and C. D. Treviño-Quintanilla. 2024. “Recent Developments in Synthesis, Properties, Applications and Recycling of Bio-Based Elastomers.” Molecules 29 (2): 387. https://doi.org/10.3390/molecules29020387.
- Caltagirone, P. E., R. S. Ginder, S. Ozcan, K. Li, A. M. Gay, J. Stonecash, K. X. Steirer, et al. 2021. “Substitution of Virgin Carbon Fiber with Low-Cost Recycled Fiber in Automotive Grade Injection Molding Polyamide 66 for Equivalent Composite Mechanical Performance with Improved Sustainability.” Composites Part B: Engineering 221:109007. https://doi.org/10.1016/j.compositesb.2021.109007.
- Franco-Urquiza, E. A., J. F. May-Crespo, C. A. Escalante Velázquez, R. Pérez Mora, and P. González García. 2020. “Thermal Degradation Kinetics of ZnO/Polyester Nanocomposites.” Polymers 12:1753. https://doi.org/10.3390/POLYM12081753.
- Franco-Urquiza, E. A., and A. V. Rentería-Rodríguez. 2023. “Effect of Nanoparticles on the Mechanical Properties of Kenaf Fiber-Reinforced Bio-Based Epoxy Resin.” Textile Research Journal 91 (11–12): 1313–1325. https://doi.org/10.1177/0040517520980459.
- Franco-Urquiza, E. A. 2021. “Clay-Based Polymer Nanocomposites: Essential Work of Fracture.” Polymers 13 (15): 2399. https://doi.org/10.3390/polym13152399.
- Franco-Urquiza, E. A., J. Cailloux, O. Santana, M. Lluisa Maspoch, and J. Cesar Velazquez Infante. 2015. “The Influence of the Clay Particles on the Mechanical Properties and Fracture Behavior of Pla/o-MMT Composite Films.” Advances in Polymer Technology 34:21470. https://doi.org/10.1002/ADV.21470.
- ISO. 2019. “ISO 527-1:2019 Plastics – Determination of Tensile Properties – Part 1: General Principles.”
- Kazi, M., M. Billah, A. R. L. Fernando, N. L. Martinez, S. Chacon, R. B. Wicker, and D. Espalin. 2019. “Thermal Analysis of Thermoplastic Materials Filled with Chopped Fiber for Large Area 3D Printing.” Solid Freeform Fabrication Symposium: 892–898. https://doi.org/10.26153/TSW/17325.
- Khalid, M. Y., A. Al Rashid, Z. Ullah Arif, W. Ahmed, H. Arshad, and A. Ali Zaidi. 2021. “Natural Fiber Reinforced Composites: Sustainable Materials for Emerging Applications.” Results in Engineering 11:100263. https://doi.org/10.1016/j.rineng.2021.100263.
- Khalid, M. Y., Z. Ullah Arif, W. Ahmed, and H. Arshad. 2022. “Recent Trends in Recycling and Reusing Techniques of Different Plastic Polymers and Their Composite Materials.” Sustainable Materials and Technologies 31:e00382. https://doi.org/10.1016/j.susmat.2021.e00382.
- Khalid, M. Y., Z. Ullah Arif, M. Hossain, and R. Umer. 2023. “Recycling of Wind Turbine Blades Through Modern Recycling Technologies: A Road to Zero Waste.” Renewable Energy Focus 44:373–389. https://doi.org/10.1016/j.ref.2023.02.001.
- Kim, K.-W., D.-K. Kim, W. Han, and B.-J. Kim. 2022. “Comparison of the Characteristics of Recycled Carbon Fibers/Polymer Composites by Different Recycling Techniques.” Molecules 27 (17): 5663. https://doi.org/10.3390/molecules27175663.
- Kornilitsina, E. V., E. A. Lebedeva, D. K. Trukhinov, S. A. Astaf’eva, and M. Balashoyu. 2023. “The Influence of Functional Additives on the Mechanical Properties of ABS Plastic.” Polymer Science, Series D 16 (3): 572–575. https://doi.org/10.1134/S1995421223030140.
- Manuel, B., J. David Hernández-Varela, D. I. Medina, and C. D. Treviño-Quintanilla. 2023. “Recent Developments in Bio-Based Polyethylene: Degradation Studies, Waste Management and Recycling.” Heliyon 9 (11): e21374. https://doi.org/10.1016/j.heliyon.2023.e21374.
- Maspoch, M. L., E. Franco‐Urquiza, J. Gamez‐Perez, O. O. Santana, and M. Sánchez‐Soto. 2009. “Fracture Behaviour of Poly[ethylene–(Vinyl Alcohol)]/organo‐Clay Composites.” Polymer International 58 (6): 648–655. https://doi.org/10.1002/pi.2574.
- Moreno-Núñez, B. A., C. Gustavo Abarca-Vidal, C. D. Treviño-Quintanilla, U. Sánchez-Santana, E. Cuan-Urquizo, and E. Uribe-Lam. 2023. “Experimental Analysis of Fiber Reinforcement Rings’ Effect on Tensile and Flexural Properties of OnyxTM–Kevlar® Composites Manufactured by Continuous Fiber Reinforcement.” Polymers 15:1252. https://doi.org/10.3390/POLYM15051252.
- Rani, M., P. Choudhary, V. Krishnan, and S. Zafar. 2021. “A Review on Recycling and Reuse Methods for Carbon Fiber/Glass Fiber Composites Waste from Wind Turbine Blades.” Composites Part B: Engineering 215:108768. https://doi.org/10.1016/j.compositesb.2021.108768.
- Rodríguez-González, J. A., V. Renteria-Rodríguez, C. Rubio-González, and E. A. Franco-Urquiza. 2024. “Spray-Coating of Graphene Nanoplatelets on Sisal Fibers and Its Influence on Electromechanical Behavior of Biocomposite Laminates.” Journal of Reinforced Plastics and Composites 43 (1–2): 3–15. https://doi.org/10.1177/07316844231152911.
- Valino, A. D., J. R. C. Dizon, A. H. Espera, Q. Chen, J. Messman, and R. C. Advincula. 2019. “Advances in 3D Printing of Thermoplastic Polymer Composites and Nanocomposites.” Progress in Polymer Science 98:101162. https://doi.org/10.1016/j.progpolymsci.2019.101162.
- van de Werken, M. S. R. Nekoda, M. R. Taha, and M. Tehrani. 2019. “Investigating the Effects of Fiber Surface Treatment and Alignment on Mechanical Properties of Recycled Carbon Fiber Composites.” Composites Part A: Applied Science and Manufacturing 119:38–47. https://doi.org/10.1016/j.compositesa.2019.01.012.
- Wei, H., W. Nagatsuka, H. Lee, I. Ohsawa, K. Sumimoto, Y. Wan, and J. Takahashi. 2018. “Mechanical Properties of Carbon Fiber Paper Reinforced Thermoplastics Using Mixed Discontinuous Recycled Carbon Fibers.” Advanced Composite Materials 27 (1): 19–34. https://doi.org/10.1080/09243046.2017.1334274.
- Witik, R. A., R. Teuscher, V. Michaud, C. Ludwig, and J. A. E. Månson. 2013. “Carbon Fibre Reinforced Composite Waste: An Environmental Assessment of Recycling, Energy Recovery and Landfilling.” Composites Part A: Applied Science and Manufacturing 49:89–99. https://doi.org/10.1016/j.compositesa.2013.02.009.
- Zhang, J., V. S. Chevali, H. Wang, and C.-H. Wang. 2020. “Current Status of Carbon Fibre and Carbon Fibre Composites Recycling.” Composites Part B: Engineering 193:108053. https://doi.org/10.1016/j.compositesb.2020.108053.