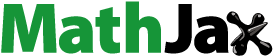
ABSTRACT
Transition metals can be introduced in therapeutic protein drugs at various steps of the manufacturing process (e.g. manufacturing raw materials, formulation, storage), and can cause a variety of modifications on the protein. These modifications can potentially influence the efficacy, safety, and stability of the therapeutic protein, especially if critical quality attributes (CQAs) are affected. Therefore, it is meaningful to understand the interactions between proteins and metals that can occur during the manufacturing process, formulation, and storage of biotherapeutics. Here, we describe a novel strategy to differentiate between ultra-trace levels of transition metals (cobalt, chromium, copper, iron, and nickel) interacting with therapeutic proteins and free metal in solution in the drug formulation using size exclusion chromatography coupled to inductively coupled plasma mass spectrometry (SEC-ICP-MS). Two monoclonal antibodies (mAbs) were coformulated and stored up to nine days in a scaled down model to mimic metal exposure from manufacturing tanks. The samples containing the mAbs were first analyzed by ICP-MS for bulk metal analysis, then studied using SEC-ICP-MS to measure the extent of metal-protein interactions. The SEC separation was used to differentiate metal associated with the mAbs from free metal in solution. Relative quantitation of metal-protein interaction was then calculated using the relative peak areas of protein-associated metal to free metal in solution and weighting it to the total metal concentration in the mixture as measured by bulk metal analysis by ICP-MS. The SEC-ICP-MS method offers an informative means of measuring metal-protein interactions during drug development.
Introduction
Transition metals found in biological systems are essential to key functions, including maintenance of protein structural integrity and catalytic enzyme activity.Citation1,Citation2 However, the presence of transition metals (e.g. Fe, Cu, Cr, Co and Ni) in therapeutic protein drug products can also serve as catalysts for a variety of interactions with proteins, including the generation of reactive oxygen species (ROS) that cause oxidation and result in modifications to proteins that can affect their stability and function.Citation3 The impact of metals on protein oxidation is of primary importance for therapeutic proteins such as monoclonal antibodies (mAbs)Citation4–8 because these type of molecules are an important class of biologic used as therapeutic agents in areas such as oncology, anti-inflammatory, and autoimmune disease.Citation9,Citation10 Therapeutic mAbs are particularly susceptible to oxidative stress from the presence of trace metals introduced at various stages of manufacturing, and various sources, such as raw materials contamination, glass vials, rubber stoppers, and stainless steel, can introduce the trace metals.Citation11,Citation12 Studies have shown that the protein concentration is a statistically significant factor impacting the metal leachability when in the presence of stainless steel (SS) because the protein can act as a complexing agent for metal ions, which suppresses the formation of a protective oxide layer on the surface of the SS.Citation13,Citation14 Brown and Merritt reported that the presence of protein produced corrosion on the outer layer of SS.Citation15
These transition metals can achieve multiple oxidation states, which increases the potential to generate ROS by facilitating the reduction of molecular oxygen, leading to radical generation and oxidative damage of the mAbs.Citation16,Citation17 Copper and iron in particular can cause production of free hydroxyl radicals by Fenton reactions. Indeed, Cu2+ and Fe3+ reduce sulfur-containing amino acids like cysteine and methionine, forming disulfide bonds and releasing Cu1+ and Fe2+ ions, which can then react with hydrogen peroxide from thiol compounds used as anti-oxidants and release hydroxyl radicals.Citation18,Citation19 This oxidative damage has the potential to affect the quality of the drug products if it affects efficacy and critical quality attributes (CQAs) of the molecule. Many studies have highlighted the modification of amino acids by trace metals such as Fe2+.Citation20–24 For example, Ouellette et al. revealed that fragmentation near the hinge region of four IgG1 molecules was catalyzed by the synergistic reaction of Fe2+ and histidine.Citation25 A similar study was performed by Glover and coworkers with copper, where they demonstrated the damaging interaction between copper and histidine residues of an IgG1 molecule, which also led to fragmentation at the hinge region.Citation26 The Food and Drug Administration requires monitoring and control of trace metal elements in drug formulations, but Pistacchio et al. highlighted that keeping the levels of both copper and iron below the allowed limits does not necessarily guarantee stable physicochemical properties and can lead to oxidation.Citation27
The detection of ultra-trace transition elements in drug product formulation and their potential interaction with the proteins is thus crucial to understanding modifications of biotherapeutic drugs. Conventional inductively coupled plasma-based methods such as atomic emission spectrometry or mass spectrometry (ICP-AES and ICP-MS, respectively) allow detection of trace metals in the drug product, but can only provide information about total metal content in solution. Methods that provide information about metal speciation, which can be used to understand the interactions between metals and proteins, are thus needed for biologics development.Citation28 Other methods such as native MS are well-suited to measure specific metal-protein binding,Citation29–32 but are generally not suitable to measure the total amount of free metal that may interact with a protein in solution. The coupling of high-performance liquid chromatography (HPLC) and ICP-MS has become the preferred tool to study such interactions.Citation33–35 This is principally due to the variety of available chromatographic separation methods, and the sensitivity of element-specific detection offered by an ICP-MS. The combination of HPLC and ICP-MS allows both identification and quantitation of specific metals within each chromatographically resolved species.
Many studies have used HPLC-ICP-MS for the quantification of proteins,Citation36,Citation37 and a number of these studies have used SEC-ICP MS with single or multiple element detection in biological matrices.Citation38–41 For example, Richarz and Brätter led a study where SEC-ICP MS was used to measure different elemental profiles in cytosols of cells from Alzheimer patients’ brains by analyzing copper, zinc, cadmium, lead, and silver. In their study, they compared the metal levels in the cytosols of brains with Alzheimer’s disease and control brains and discovered that there was an increased level of oxidized metallothioneins in Alzheimer’s disease brains.Citation42,Citation43 Gailer and coworkers demonstrated the coupling of HPLC and SEC-ICP-AES and performed the simultaneous analysis of copper, iron and zinc species in rabbit plasma.Citation35 The relatively high limit of detection of the ICP-based instrument used in that study did not hinder the analysis, as the metal concentration of the species found in the plasma samples ranged from 0.2 mg/L to 3.0 mg/L. Overall, their method allowed the detection of 12 metalloproteins and metallopeptides in less than 25 min. These studies highlight the utility of SEC-ICP-MS to measure metal-protein interactions in complex matrices. However, the metal concentrations found in a formulated drug product will be significantly lower than those in blood, plasma or urine, which means that previously reported methods are not sensitive enough to detect metals in formulated drug product.Citation44 Therefore, there is a need for an accurate and highly sensitive method that can identify metal-protein interaction at ultra-trace levels.
Herein, we describe an SEC-ICP-MS method capable of measuring metal-protein interactions present in a therapeutic drug product consisting of two co-formulated mAbs at ultra-trace levels. We first use ferritin and Cu,Zn-superoxide dismutase, two metalloproteins containing transition metals, to develop the method and provide proof of concept. We then analyzed co-formulated mAbs stressed using stainless steel coupons to simulate the level of metal that may be introduced through drug substance manufacturing. Moreover, we calculate the relative quantitation of metal-protein interaction and free-metal, using a relative peak area quantification approach combined with total metal determination. Our technique led to an improved understanding of metal-protein interactions in biologic formulations, and more importantly serves as a tool to better understand the role of metal-protein interactions in metal-induced protein degradation often associated with biologics manufacturing.
Results
Demonstration of the SEC-ICP-MS capability using metalloproteins
The first step of the study was to evaluate and optimize the separation of protein-associated metals and free metals species. Ferritin and Cu,Zn-superoxide dismutase (SOD) were chosen as appropriate metalloproteins for method development because of their high level of associated transition metals and diverse molecular weights. Both standards were used to optimize column choice and flow rates eventually leading to the parameters outlined in the LC-MS Method section.
illustrates the separation of two distinct peaks for the ferritin, eluting between 2.9 and 3.4 min in the UV trace, which was achieved using the final chromatographic conditions. In the ICP-MS chromatogram, the first peak, which appears at 2.9 min, corresponds to the aggregated (high molecular weight) species while the second and main peak eluting at 3.4 min corresponds to ferritin monomer, and matches the profile and intensities observed in the UV trace.Citation45 The retention time of the main ferritin peak, which corresponds to a molecular mass of 480 kDa, is aligned with the observations in the literature.Citation46,Citation47 A calibration curve using ferritin at iron concentrations varying between 5.0 and 100 µg/L was also generated and demonstrated good linearity within that range (Figure S2).
Figure 1. Chromatograms by SEC-UV (280 nm) and SEC-ICP-MS detection of a) equine ferritin and b) bovine Cu, Zn-SOD (UV trace off axis for clarity purposes).

In addition to ferritin, Cu,Zn-SOD was analyzed to evaluate the chromatographic conditions (). By comparing the profiles of both the UV and SEC-ICP-MS traces, the major peak at 4.2 min exhibits both UV absorbance, and Cu and Zn signal. However, the UV trace also reveals that the standard chosen is not fully pure and contains other absorbing species at a retention time of 4.4 min without association of Cu and Zn ions. The peak areas of each resolved peak were evaluated, and the corresponding Cu,Zn-containing species accounted for 55% of the total area. This implies that the protein standard contains species other than the metalloprotein that account for about 45%.
Study of metal-protein interaction in co-formulated mAbs
Total content of metals in monoclonal antibodies SS study samples
Transition metals can be introduced in the manufacturing process through various means, such as raw material contamination, and direct contact with unit operations. The most commonly used grade of stainless steel used in biopharmaceutical applications is 316 L, which is an alloy containing mainly chromium, iron, and nickel, with minor amounts of copper.Citation11,Citation48 The stainless steel contact through unit operations is a major source of the metal contamination in biologics drug products, as this contact takes place in various tanks and stainless steel piping.Citation13 During manufacturing and storage, biologics formulations are in contact with stainless steel at different fill volume per unit contact surface areas. In this study, a smaller volume per unit contact surface (2.0 mL/cm2 was chosen to represent a high level of interaction between the drug product and the SS coupons and incubation occurred for up to 9 days. In large-scale production, tanks generally have higher volume per unit surface, of the order of 10 mL/cm2 or more. Bulk metal analysis of the four stainless steel-stressed co-formulated mAb samples was performed in triplicate by ICP-MS/MS following the acid digestion protocol. The total concentrations of metals of interest are reported in .
Table 1. Total metal content in mAb stress study samples (n = 3, 95% confidence interval).
Compared to the t=0 sample, the samples with the SS coupons showed higher levels of chromium, iron, and nickel, which is consistent with the composition of SS 316 L, and the specified metal leached over the course of the experiment. Interestingly, the cobalt concentration did not change, probably because this element is not present in high concentration in the SS grade used in the study.
Metal speciation in mAb samples by SEC-ICP-MS
The measurement of metal-protein interaction was conducted for the stressed co-formulated mAb samples. The samples were first diluted to a final protein concentration of 1 mg/mL before injecting 5 µL into the SEC-ICP-MS system. These conditions allowed partial separation of the co-formulation mAbs, as displayed in the UV spectra obtained at 280 nm (). Although the primary separation mechanism of SEC is based on hydrodynamic radius, the partial separation of the co-formulated mAbs suggests that secondary interactions such as differences in hydrophobicity of the two molecules also affect the separation, as described in the work of Yan et al.Citation49 The separation of the two mAbs was confirmed by measuring the molecular weight of each peak by native SEC-MS (Figure S1 and Table S3).
Figure 2. UV chromatograms of a) 10 µg loading that allows for co-formulation separation and b) 400 µg loading that allows for metal-protein binding affinity analysis.

While loading the column with a total of 5 µg of protein allowed UV detection of the co-formulated mAbs (), it did not permit the detection of metal-protein interaction by ICP-MS, as only background noise was detected due to the low level of metal present in the samples. Therefore, the injection amount was increased 80-fold to 400 µg of protein injected on column. This increase in the protein loading on the column resulted in peak broadening and saturation of the detector (). Moreover, as we increased the loading, a small peak is detected at 2.9 min, indicating the detection of minor aggregate species.
The optimized loading of SS-stressed mAb samples helped facilitate the detection of protein interaction with chromium, cobalt, nickel, and iron, as depicted in . The SEC-ICP-MS chromatograms obtained for chromium, cobalt, and nickel () consisted of a single peak between 3.19 and 3.83 min, which correlated with the protein retention time detected by UV. Later eluting peaks were observed between 5 and 7 minutes, which indicates free metal in solution that is eluting in the permeation limit of the column. In the case of the Fe chromatogram (), two distinct peaks in the time-range of protein elution indicates association of Fe with the protein, including a peak that likely represents protein aggregate at 2.85 min and the main peak at 3.45 min. Similar to chromium, cobalt and nickel, late-eluting species containing Fe were observed between 5.15 and 7.40 min, indicating free iron or iron associated with smaller molecules. Further investigation is under way to identify the free iron species leading to the presence of two peaks in the free iron region. The analysis of the formulation buffer without mAb confirmed the retention time of the free metal species in the formulation (Figure S3).
Figure 3. SEC-ICP-MS chromatogram of various time point of the SS samples analyzed for a) chromium, b) cobalt, c) nickel, and d) iron.

The SEC-ICP-MS chromatograms of the various time points of the SS study indicate a general increase in the peak area over time. For chromium, cobalt and nickel, there was an increase in the peak area only in the late-eluting, free metal species region, while the opposite was observed for iron. Indeed, the free iron species peak areas (RT = 5.2–7.4 min) were nearly unchanged, while the peaks indicating mAb-iron interaction increased (RT = 2.8–4.0 min), suggesting that the mAb interaction with iron selectively accumulates over time, but not for the other three metals ().
Identification of copper-histidine interactions
During our analysis, we observed an artificially high peak area at t = 0 sample in the copper chromatograms in the free copper region (~5.5 min), as depicted in . Further study suggested that the presence of histidine in the formulation buffer resulted in copper ions leaching from the HPLC system. Indeed, among the amino acids, L-histidine is one of the strongest copper-chelating ligands, as demonstrated by Deschamps and coworkers.Citation50 Prior to the addition of a wash step, the peak area of copper did not correlate with the total copper content obtained by bulk ICP-MS/MS analysis (), with the highest peak area being for t = 0 sample (). As samples were injected, the peak area decreased, indicating that less copper was stripped from the HPLC components as the system was continuously flushed with mobile phase. In an attempt to counteract this phenomenon, we added an L-histidine washing step before and between runs to remove any contribution of copper leaching from the instrument. The implementation of the histidine wash step solved this issue and generated results that agreed with the trends observed by bulk ICP-MS/MS analysis (), as illustrated in . It was then possible to observe that the copper-protein interaction peak area increased from t = 0 to 5 days in the mAb eluting range and further stabilized after 5 days. Similar to chromium, cobalt and nickel, a peak increase for copper was detected in the free metal species region at the later eluting range.
Figure 4. SEC-ICP-MS chromatogram of copper at various time point of the SS incubated samples a) without the L-histidine washing step showing artificially high peak areas in the free metal species regions and b) with the L-histidine washing step resolving the artificially high peak areas.

Calculation of peak area distribution of SS stressed mAbs
Relative semi-quantitation of the metal concentration for each species detected by SEC-ICP-MS was calculated using a relative peak area quantification approach adapted from del Castillo-Busto and coworkers,Citation51 which does not require standards. Briefly, the sum of the peak areas from the metal species in the co-formulation (Table S4) was set equal to the total concentration of metals (μg/L) obtained by bulk ICP-MS/MS analysis (). Using this approach, we assume that the mass balance of the bulk analysis is preserved when performing the SEC-ICP-MS analysis i.e., no metal is lost from the sample during the analysis, or the sample is not contaminated when introduced in the system. The concentrations of each metal species (M) and their relative proportions in the SS study samples were calculated following Equationequation 1(1)
(1) :
Table 2. Relative semi-quantitation of metal associated to mAbs in coformulated SS study by SEC-ICP-MS using the combination of metal-protein peak area distribution and total metal results by ICP-MS/MS.
The results obtained by the peak area distribution quantification approach for the SS study samples are summarized in . The results demonstrate that a small proportion of chromium, cobalt, copper, and nickel is associated with the mAbs, but that the majority of these metal-species found in the samples are of small molecular weight and free in solution. Iron exhibits the opposite behavior where the concentration of iron-associated species increased with time of SS exposure, whereas the concentration of low molecular weight iron species stayed nearly constant.
In order to confirm that the increase in the concentration of iron associated with the proteins was not specific to the samples tested, we analyzed two additional co-formulated mAbs that followed the same SS study protocol. The identical phenomenon was observed again as depicted in , indicating the tendency of iron to associate with proteins rather than accumulate in solution.
Figure 5. Iron chromatograms depicting the increase of Fe-associated species over time in co-formulation a and co-formulation b.

Discussion
The analytical task of determining the interactions of metal and proteins, and their impact on formulated drug products, can be simplified using the right tools. To this end, we developed the novel ultra-trace multi-element SEC-ICP-MS method to gain insight on metal-protein interactions that could lead to oxidation and affect the CQAs of the drug. The combination of SEC and ICP-MS permits the separation of metal-containing species in formulated drug products according to their size and provides valuable data that cannot be obtained by conventional ICP-MS methods or other assays. Indeed, determination of the total concentration of metals in formulated drug products does not provide enough information about the distribution of ultra-trace levels of metals among proteins and small molecular weight species.
This novel SEC-ICP-MS method allows fast and efficient separation of high and low molecular weight species in co-formulated mAbs. In co-formulated samples, the injection of 5 µg of mAbs using the chromatographic conditions we developed enabled partial separation of the two mAbs. In order to detect ultra-trace levels of protein-metal interactions, the loading was increased to 400 µg, which allowed detection of metal species with the same retention time as the mAbs in the co-formulated drug product, strongly suggesting interactions between Fe and the mAbs in solution. These interactions were also observed in two other co-formulated mAbs, indicating that the phenomenon is related to the metal rather than the mAbs or the formulations. However, further investigation is needed to better understand the behavior of free iron in the sample formulation. Sensitivity to L-histidine contained in the formulation of the drug product was observed, artificially elevating the free copper peak intensity, which was remedied with a histidine washing step. Although the mechanism remains unclear, our observations suggest that protein-metal interactions may occur upon exposure of protein to stainless steel during the manufacturing process and should be closely monitored.
We believe that the technique developed here will be an invaluable tool to monitor mAb manufacturing and to support studies designed to understand metal-induced protein aggregation and modification in biologic products. Moreover, it can facilitate deeper understanding of the impact of metal exposure during drug development and manufacturing.
Materials and methods
Materials
Purified water produced using a Milli-Q system (Millipore, Bedford, MA) was used to prepare all solutions. All chemicals and reagents were trace-metal grade and used without further purification. Ammonium acetate (99.999% trace metal basis), L-histidine (BioUltra grade), type 1 equine ferritin (Catalog no. F4503-100 MG) and bovine Cu,Zn-superoxide dismutase (Cu,Zn-SOD, catalog no. S7571-15KU) were all purchased from Sigma Aldrich (St. Louis, MO). The HPLC mobile phases consisted of 200 mM ammonium acetate at a pH of 6.5. The washing mobile phase consisted of 10 µM L-histidine buffer in water.
A multi-element standard at a nominal concentration of 10 mg/L (High Purity Standard, North Charleston, USA) was used to prepare calibration standards in mobile phase and used to characterize free species. Both type 1 equine ferritin and bovine Cu,Zn-SOD were prepared by diluting the appropriate amount of standard solution in ammonium acetate buffer to achieve a final protein concentration of 140 µg/mL and used as control material for protein-associated metal species.
Equipment
An Agilent 8900 ICP-MS/MS (Santa Monica, CA) equipped with a Micro Mist concentric nebulizer and a Scott double-pass spray chamber was used for the study. The chromatographic system for SEC-ICP-MS experiments was an Agilent 1290 HPLC (Santa Monica, CA) with a variable loop (5–20 µL) and a diode array detector (DAD). The HPLC system was coupled to the ICP-MS/MS through an interface consisting of a 45 cm PEEK capillary tubing to connect the column outlet to the nebulizer inlet. The SEC column was an Acquity UPLC Protein BEH SEC column (200 Å, 1.7 µm, 4.6 × 150 mm, 1.7 µm, Waters, Millford, MA) Native MS experiments were carried out on a Orbitrap Eclipse mass spectrometer coupled to a Vanquish Flex UPLC with a variable wavelength detector (Thermo Scientific) using the same SEC column and mobile phase as SEC-ICP-MS experiments. A “resistor tube” was used between the ESI probe and ground to lower the electrospray current, similar to that described previously.Citation52
LC-MS methods
Separation for SEC-ICP MS experiments of 52Cr, 56Fe, 59Co and 60Ni was performed over 8 min using an isocratic flow of 200 mM ammonium acetate (pH unadjusted) at 350 µL/min. The column was first conditioned by passing at least 10 mL of mobile phase through it before injection of the standards and samples. Isocratic elution was used to separate both free and associated metal species. For the analysis of 65Cu, the separation was performed as follows: an initial isocratic flow of 200 mM ammonium acetate for 8 min at 0.35 mL/min followed by a wash step using 10 mM of L-histidine at a flow rate of 0.5 mL/min, and then equilibration with 200 mM ammonium acetate for 7 min back to starting conditions to prepare the column for the next injection.
Injection amounts were 400 µg for mAb samples and 10 µg for both ferritin, Cu,Zn-SOD and free metal standard. The ICP-MS/MS instrument settings (nebulizer gas flow, RF power and lens voltage) were checked and tuned daily using Agilent tuning solution. The isotopes monitored for mAb-metal interaction experiments included 52Cr, 56Fe, 59Co, 60Ni and 65Cu. The argon-based and other polyatomic interferencesCitation53 were successfully removed by operating the ICP-MS in MS/MS mode and with the introduction of 3 mL/min of helium gas in the collision cell.
Peak areas of the separated metal species were processed using Agilent MassHunter software. The operating conditions for SEC-ICP-MS are summarized in Table S1. Native SEC-MS experiments were performed with the same separation conditions with an additional switching valve used to divert nonvolatile salts to waste after protein elution. The MS parameters for native MS experiments can be found in Table S2.
Monoclonal antibody stainless steel study
The mAb co-formulation was prepared by mixing equal portion of mAb A, an IgG1, and mAb B, an IgG4, to achieve a final protein concentration of 20 mg/mL. The co-formulation was divided and dispensed into four 30 mL sterile polyethylene terephthalate glycol (PETG) media bottles separately. Thirty 316 L grade stainless steel (SS) coupons (Stollen Machine & Tool, Kenilworth, NJ) of 2 cm2 were divided equally and added to three of the sample bottles, while the last one acted as negative control. All three bottles containing the SS coupons were staged on stability at 25°C over time points of 5, 7, and 9 days. Once a stability time point was reached, the bottle was removed from the 25°C chamber and the sample was transferred (without coupons) to a sterile PETG media bottle. The process was repeated for each time point. All samples were stored at 5°C until prior to SEC-ICP-MS testing.
Acid digestion of SS study samples for ICP-MS/MS analysis
For sample digestion, a gentle acidic digestion protocol using a hot block was developed. In short, 250 μL of sample was mixed with 250 μL of a digestion solution containing equal parts of nitric acid and hydrogen peroxide in a metal-free polystyrene tube. This solution is then gently heated to a maximum of 70°C for up to 1 h to break down proteins that would interfere with the measurement. After compensation for volume loss, the clear solution was diluted with Milli-Q water to a dilution factor of 10, which allows for the determination of ultra-trace elements in mAb samples by ICP-MS/MS.
Supplemental Material
Download MS Word (257.4 KB)Disclosure statement
No potential conflict of interest was reported by the author(s).
Supplementary material
Supplemental data for this article can be accessed online at https://doi.org/10.1080/19420862.2023.2199466
Additional information
Funding
References
- Dupont CL, Yang S, Palenik B, Bourne PE. Modern proteomes contain putative imprints of ancient shifts in trace metal geochemistry. Proc Natl Acad Sci U S A. 2006 ;103(47):17822–9. doi:10.1073/PNAS.0605798103. PMID:17098870
- Saito MA, Sigman DM, Morel FMM. The bioinorganic chemistry of the ancient ocean: the co-evolution of cyanobacterial metal requirements and biogeochemical cycles at the Archean–Proterozoic boundary? Inorganica Chim Acta. 2003;356:308–18. doi:10.1016/S0020-1693(03)00442-0.
- Lemire JA, Harrison JJ, Turner RJ. Antimicrobial activity of metals: mechanisms, molecular targets and applications. Nat Rev Microbiol. 2013 ;11(6):371–84. doi:10.1038/nrmicro3028. PMID:23669886
- Stadtman ER. Metal ion-catalyzed oxidation of proteins: biochemical mechanism and biological consequences. Free Radic Biol Med. 1990 ;9(4):315–25. doi:10.1016/0891-5849(90)90006-5. PMID:2283087
- Narhi LO, Luo Q, Wypych J, Torosantucci R, Hawe A, Fujimori K, Nashed-Samuel Y, Jawa V, Joubert MK, Jiskoot W. Chemical and biophysical characteristics of monoclonal antibody solutions containing aggregates formed during metal catalyzed oxidation. Pharm Res. 2017;34(12):2817–28. doi:10.1007/s11095-017-2262-8.
- Jomova K, Valko M. Advances in metal-induced oxidative stress and human disease. Toxicology. 2011 ;283(2–3):65–87. doi:10.1016/J.TOX.2011.03.001. PMID:21414382
- Paik SR, Shin HJ, Lee JH. Metal-catalyzed oxidation of α-Synuclein in thE presence of copper(ii) and hydrogen peroxide. Arch Biochem Biophys. 2000 ;378(2):269–77. doi:10.1006/ABBI.2000.1822. PMID:10860544
- Castro-Acosta RM, Rodríguez-Limas WA, Valderrama B, Ramírez OT, Palomares LA. Effect of metal catalyzed oxidation in recombinant viral protein assemblies. Microb Cell Fact. 2014 ;13(1):1–12. doi:10.1186/1475-2859-13-25/FIGURES/9. PMID:24533452
- Mukherjee AG, Wanjari UR, Bradu P, Murali R, Kannampuzha S, Loganathan T, Doss GP, B P AP, Renu K, Dey A, et al. The crosstalk of the human microbiome in breast and colon cancer: a metabolomics analysis. Crit Rev Oncol Hematol. 2022;176:103757. doi:10.1016/J.CRITREVONC.2022.103757.
- Zhou H, Xu Z, Mascelli MA, Davis HM. Monoclonal antibodies and antibody-based biotherapeutics in inflammatory diseases. Pharm Biotechnol Fundam Appl Fourth Ed. 2013:393–412. doi:10.1007/978-1-4614-6486-0_20/TABLES/1.
- Zhou S, Schöneich C, Singh SK. Biologics formulation factors affecting metal leachables from stainless steel. AAPS PharmScitech. 2011 ;12(1):411–21. doi:10.1208/s12249-011-9592-3. PMID:21360314
- Hedberg Y, Karlsson M-E, Wei Z, Žnidaršič M, Odnevall Wallinder I, Hedberg J. Interaction of Albumin and Fibrinogen with Stainless Steel: influence of sequential exposure and protein aggregation on metal release and corrosion resistance. CORROSION. 2017;73(12):1423–36. doi:10.5006/2504.
- Zhou S, Evans B, Schöneich C, Singh SK. Biotherapeutic formulation factors affecting metal leachables from stainless steel studied by design of experiments. AAPS PharmScitech. 2012 ;13(1):284–94. doi:10.1208/s12249-011-9747-2. PMID:22246735
- Kocijan A, Milošev I, Pihlar B. The influence of complexing agent and proteins on the corrosion of stainless steels and their metal components. J Mater Sci Mater Med. 2003;14(1):69–77. doi:10.1023/A:1021505621388.
- Brown SA, Merritt K. Electrochemical corrosion in saline and serum. J Biomed Mater Res. 1980 ;14(2):173–75. doi:10.1002/JBM.820140208. PMID:7358745
- Valko M, Morris H, Cronin M. Metals, toxicity and oxidative stress. curr Med Chem. 2005 ;12(10):1161–208. doi:10.2174/0929867053764635. PMID:15892631
- Bachi A, Dalle-Donne I, Scaloni A. Redox proteomics: chemical principles, methodological approaches and biological/biomedical promises. Chem Rev. 2013;113(1):596–698. doi:10.1021/cr300073p.
- Prabhu A, Gadgil M. Trace metals in cellular metabolism and their impact on recombinant protein production. Process Biochem. 2021;110:251–62. doi:10.1016/J.PROCBIO.2021.08.006.
- Hua Long L, Halliwell B. Oxidation and generation of hydrogen peroxide by thiol compounds in commonly used cell culture media. Biochem Biophys Res Commun. 2001 ;286(5):991–94. doi:10.1006/bbrc.2001.5514. PMID:11527398
- Hoshi T, Heinemann SH. Regulation of cell function by methionine oxidation and reduction. J Physiol. 2001 ;531(1):1–11. doi:10.1111/j.1469-7793.2001.0001j.x. PMID:11179387
- Lim JM, Kim G, Levine RL. Methionine in proteins: it’s not just for protein initiation anymore. Neurochem Res. 2019;44(1):247–57. doi:10.1007/s11064-017-2460-0.
- Daugherty AL, Mrsny RJ. Formulation and delivery issues for monoclonal antibody therapeutics. Adv Drug Deliv Rev. 2006 ;58(5–6):686–706. doi:10.1016/J.ADDR.2006.03.011. PMID:16839640
- Fevre A, Kiessig S, Bonnington L, Olaf Stracke J, Bulau P. Quantifying methionine sulfoxide in therapeutic protein formulation excipients as sensitive oxidation marker. J Chromatogr B. 2022;1189:123092. doi:10.1016/J.JCHROMB.2021.123092. PMID:35026663.
- Kumar S, Zhou S, Singh S. Metal ion leachates and the physico-chemical stability of Biotherapeutic drug products. Curr Pharm Des. 2014 ;20(8):1173–81. doi:10.2174/13816128113199990063. PMID:23713770
- Ouellette D, Alessandri L, Piparia R, Aikhoje A, Chin A, Radziejewski C, Correia I. Elevated cleavage of human immunoglobulin gamma molecules containing a lambda light chain mediated by iron and histidine. Anal Biochem. 2009 ;389(2):107–17. doi:10.1016/J.AB.2009.03.027. PMID:19318085
- Glover ZK, Basa L, Moore B, Laurence JS, Sreedhara A. Metal ion interactions with mAbs: part 1 pH and conformation modulate copper-mediated site-specific fragmentation of the IgG1 hinge region. MAbs. 2015 ;7(5):901–11. doi:10.1080/19420862.2015.1062193. PMID:26121230
- Pistacchio A, Baroni F, Cecchini I, Verani R, Stornaiuolo M, Palmese A, Pergola C. Impact of four inorganic impurities – iron, copper, nickel and zinc - on the quality attributes of a Fc-fusion protein upon incubation at different temperatures. Eur J Pharm Sci. 2022;172:106139. doi:10.1016/J.EJPS.2022.106139. PMID:35134506.
- Ackerman CM, Lee S, Chang CJ. Analytical methods for imaging metals in biology: from transition metal metabolism to transition metal signaling. Anal Chem. 2017 ;89(1):22–41. doi:10.1021/acs.analchem.6b04631. PMID:27976855
- Romano CA, Zhou M, Song Y, Wysocki VH, Dohnalkova AC, Kovarik L, Paša-Tolić L, Tebo BM. Biogenic manganese oxide nanoparticle formation by a multimeric multicopper oxidase Mnx. Nat Commun. 2017 81;8(1):1–8. doi: 10.1038/s41467-017-00896-8. PMID:28963463.
- Wongkongkathep P, Han JY, Choi TS, Yin S, Kim HI, Loo JA. Native top-down mass spectrometry and ion mobility MS for characterizing the Cobalt and Manganese Metal binding of α-Synuclein protein. J Am Soc Mass Spectrom. 2018 ;29(9):1870–80. doi:10.1007/s13361-018-2002-2. PMID:29951842
- Lin CW, McCabe JW, Russell DH, Barondeau DP. Molecular mechanism of ISC Iron–Sulfur cluster biogenesis revealed by high-resolution native mass Spectrometry. J Am Chem Soc. 2020 ;142(13):6018–29. doi:10.1021/jacs.9b11454. PMID:32131593
- Aron AT, Petras D, Schmid R, Gauglitz JM, Büttel I, Antelo L, Zhi H, Nuccio SP, Saak CC, Malarney KP, et al. Native mass spectrometry-based metabolomics identifies metal-binding compounds. Nat Chem. 2021;14(1):100–09. 10.1038/s41557-021-00803-1. PMID:34795435.
- Luo Y, Ronk M, Joubert MK, Semin D, Nashed-Samuel Y. Determination of interactions between antibody biotherapeutics and copper by size exclusion chromatography (SEC) coupled with inductively coupled plasma mass spectrometry (ICP/MS). Anal Chim Acta. 2019;1079:252–59. doi:10.1016/J.ACA.2019.06.047. PMID:31387718.
- Wojcieszek J, Ruzik L. Operationally defined species characterization and bioaccessibility evaluation of cobalt, copper and selenium in Cape gooseberry (Physalis Peruviana L.) by SEC-ICP MS. J Trace Elem Med Biol. 2016;34:15–21. doi:10.1016/j.jtemb.2015.12.001. PMID:26854240.
- Manley SA, Byrns S, Lyon AW, Brown P, Gailer J. Simultaneous Cu-, Fe-, and Zn-specific detection of metalloproteins contained in rabbit plasma by size-exclusion chromatography–inductively coupled plasma atomic emission spectroscopy. J Biol Inorg Chem. 2009 ;14(1):61–74. doi:10.1007/s00775-008-0424-1. PMID:18781345
- Wang M, Feng W-Y, Zhao Y-L, Chai Z-F. ICP-MS-based strategies for protein quantification. Mass Spectrom Rev. 2009;29(2):326–48. doi:10.1002/mas.20241.
- Cid-Barrio L, Calderón-Celis F, Abásolo-Linares P, Fernández-Sánchez ML, Costa-Fernández JM, Encinar JR, Sanz-Medel A. Advances in absolute protein quantification and quantitative protein mapping using ICP-MS. TrAc Trends Anal Chem. 2018;104:148–59. doi:10.1016/J.TRAC.2017.09.024.
- Theiner S, Grabarics M, Galvez L, Varbanov HP, Sommerfeld NS, Galanski M, Keppler BK, Koellensperger G. The impact of whole human blood on the kinetic inertness of platinum(iv) prodrugs – an HPLC-ICP-MS study. Dalt Trans. 2018;47(15):5252–58. doi:10.1039/c7dt04537a.
- Pechancová R, Gallo J, Milde D, Pluháček T. Ion-exchange HPLC-ICP-MS: a new window to chromium speciation in biological tissues. Talanta. 2020;218:121150. doi:10.1016/J.TALANTA.2020.121150. PMID:32797905.
- Nong Q, Chen X, Hu L, Huang Y, Luan T, Liu H, Chen B. Identification and characterization of Gd-binding proteins in NIH-3T3 cells. Talanta. 2020;219:121281. doi:10.1016/J.TALANTA.2020.121281. PMID:32887171.
- Bishop DP, Hare DJ, Clases D, Doble PA. Applications of liquid chromatography-inductively coupled plasma-mass spectrometry in the biosciences: a tutorial review and recent developments. TrAc Trends Anal Chem. 2018;104:11–21. doi:10.1016/J.TRAC.2017.09.017.
- Richarz AN, Brätter P. Speciation analysis of trace elements in the brains of individuals with Alzheimer’s disease with special emphasis on metallothioneins. Anal Bioanal Chem. 2002 ;372(3):412–17. doi:10.1007/S00216-001-1187-5. PMID:11939525
- Richarz A-N, Wolf C, Brätter P. Determination of protein-bound trace elements in human cell cytosols of different organs and different pathological states. Analyst (Lond). 2003;128(6):640–45. doi:10.1039/b300299n.
- Lopez-Avila V, Sharpe O, Robinson WH. Determination of ceruloplasmin in human serum by SEC-ICPMS. Anal Bioanal Chem. 2006 ;386(1):180–87. doi:10.1007/s00216-006-0528-9. PMID:16794815
- Kumar K. Iron Assay and size exclusion high performance liquid Chromatography of Ferritin and Magnetoferritin. J Liq Chromatogr Relat Technol. 1997;20(20):3351–64. doi:10.1080/10826079708005836.
- Nischwitz V, Michalke B, Kettrup A. Extraction and characterisation of trace element species from porcine liver samples using online HPLC-ICP-MS and offline HPLC-ESI-MS. J Anal at Spectrom. 2003;18(5):444–51. doi:10.1039/B212526A.
- Jahromi EZ, White W, Wu Q, Yamdagni R, Gailer J. Remarkable effect of mobile phase buffer on the SEC-ICP-AES derived Cu, Fe and Zn-metalloproteome pattern of rabbit blood plasma. Metallomics. 2010 ;2(7):460–68. doi:10.1039/C003321A. PMID:21072345
- Mohammad Soltani H, Tayebi M. Comparative study of AISI 304L to AISI 316L stainless steels joints by TIG and Nd: yAG laser welding. J Alloys Compd. 2018;767:112–21. doi:10.1016/J.JALLCOM.2018.06.302.
- Yan Y, Xing T, Wang S, Daly TJ, Li N. Coupling mixed-mode size exclusion chromatography with native mass spectrometry for sensitive detection and quantitation of Homodimer impurities in Bispecific IgG. Anal Chem. 2019;91(17):11417–24. doi:10.1021/acs.analchem.9b02793.
- Deschamps P, Kulkarni PP, Gautam-Basak M, Sarkar B. The saga of copper(ii)–l-histidine. Coord Chem Rev. 2005;249(9–10):895–909. doi:10.1016/J.CCR.2004.09.013.
- Del Castillo Busto ME, Cuello-Nunez S, Ward-Deitrich C, Morley T, Goenaga-Infante H. A fit-for-purpose copper speciation method for the determination of exchangeable copper relevant to Wilson’s disease. Anal Bioanal Chem. 2022 ;414(1):561–73. doi:10.1007/s00216-021-03517-y. PMID:34272592
- Vanaernum ZL, Busch F, Jones BJ, Jia M, Chen Z, Boyken SE, Sahasrabuddhe A, Baker D, Wysocki VH. Rapid online buffer exchange for screening of proteins, protein complexes and cell lysates by native mass spectrometry. Nat Protoc. 2020;15(3):1132–57. doi:10.1038/s41596-019-0281-0.
- Harrington CF, Elahi S, Merson SA, Ponnampalavanar P. Quantitative analysis of Iron-containing protein Myoglobin in different foodstuffs by liquid chromatography coupled to high-resolution inductively coupled Plasma mass spectrometry. J AOAC Int. 2004 ;87(1):253–58. doi:10.1093/JAOAC/87.1.253. PMID:15084108