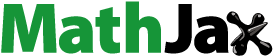
ABSTRACT
Despite tyrosine sulfation being a relatively common post-translational modification (PTM) on the secreted proteins of higher eukaryotic organisms, there have been surprisingly few reports of this modification occurring in recombinant monoclonal antibodies (mAbs) expressed by mammalian cell lines and even less information regarding its potential impact on mAb efficacy and stability. This discrepancy is likely due to the extreme lability of this modification using many of the mass spectrometry methods typically used within the biopharmaceutical industry for PTM identification, as well as the possible misidentification as phosphorylation. Here, we identified sulfation on a single tyrosine residue located within the identical variable region sequence of a 2 + 1 bispecific mAbs heavy and heavy-heavy chains using a multi-enzymatic approach in combination with mass spectrometry analysis and examined its impact on binding, efficacy, and physical stability. Unlike previous reports, we found that tyrosine sulfation modestly decreased the mAb cell binding and T cell-mediated killing, primarily by increasing the rate of antigen disassociation as determined from surface plasmon resonance-binding experiments. We also found that, while this acidic modification had no significant impact on the mAb thermal stability, sulfation did modestly increase its rate of aggregation, presumably by lowering the mAb’s colloidal stability as indicated by polyethylene glycol induced liquid–liquid phase separation experiments.
Introduction
Monoclonal antibodies (mAbs) and mAb variants have become one of the fastest growing classes of therapeutics within the biopharmaceutical industry due in part to their greater specificity and lower toxicity than small-molecule pharmaceutics.Citation1–3 Like all protein biologics, however, mAbs are susceptible to numerous post-translational modifications (PTMs) that may limit their manufacturability and shelf life by either directly impacting efficacy, half-life and immunogenicity or by indirectly promoting physical instabilities such as aggregation by altering conformational and/or colloidal stabilities.Citation4,Citation5 Examples of PTMs include common chemical modifications such as methionine oxidation, asparagine deamidation, and aspartic acid isomerization that may be modulated by environmental and solvent conditions,Citation6–8 as well as enzymatically catalyzed PTMs that may occur during cell culture such as phosphorylation, cysteinylation and aberrant glycosylation.Citation9–11 Our investigation focuses on an example from the second category of PTMs, the enzymatic addition of sulfate trioxide to the phenolic hydroxyl group of the tyrosine (Tyr) side chain. Similar to Tyr phosphorylation, this highly acid PTM results in a nominal mass increase of 80 Da.Citation12
Tyrosine sulfation (sY) is catalyzed by two trans-Golgi network membrane-bound tyrosylprotein sulfotransferases (TPSTs) that utilize 3’-phosphoadenosine-5’-phosphosulfate (PAPS) as a sulfate donorCitation13 and is thought to modulate the protein–protein interactions of certain secreted and trans-membrane spanning proteins of higher eukaryotes.Citation13,Citation14 Unlike some other PTMs, however, no clear consensus sequence has been identified that may predict which Tyr residues will be targeted for sulfation.Citation12 Instead, a set of sequence characteristics have been determined that ostensibly promote sulfation by enhancing the TPST affinity for Tyr.Citation14 Examples of these sequence features include the presence of acidic residues near the vicinity of the affected Tyr, particularly on its amino-terminal side, and the absence of nearby disulfide bonds and glycosylation.Citation14–16 It is believed that roughly 1% of all the tyrosine residues within the eukaryotic proteome are sulfated;Citation12,Citation17 yet, to our knowledge only a few Chinese hamster ovary (CHO) cell-expressed human therapeutic mAbs that contain sY have been described to date.Citation18,Citation19 Potential reasons for this dichotomy may be related to some of the challenges associated with identifying and localizing this PTM using many of the standard mass spectrometry (MS)-based techniques, described later, that are widely used within the biopharmaceutical industry for these purposes.
In this investigation, sY was identified in another CHO-derived mAb, a trivalent 2 + 1 heterodimeric bispecific mAb named mAb-X; the molecular format of similar 2 + 1 immunotherapeutic mAbs is reviewed in detail elsewhere.Citation20–23 Briefly, one arm of mAb-X is composed of a canonical antigen-binding fragment (Fab) region that binds a tumor-associated antigen (TAA) and the other arm consists of this same TAA-binding Fab linked in a “head-to-tail” fashion through its heavy chain (HC) to a second Fab that binds a T cell-specific antigen (TSA). The second constant (CH2) and third constant (CH3) domains of the HC (50240.0 Da) and longer HC or heavy–heavy chain (HHC) (75138.6 Da) dimerize in the crystallizable fragment (Fc) region as typical for other IgG mAbs. Using a multi-enzymatic approach and similar methodologies used in the previous two sY reports,Citation18,Citation19 sulfation was localized within one of the complementary-determining regions (CDRs) of the mAb-X HC and HHC that bind the TAA. In addition to the identification and localization of this novel PTM, its impact on binding, cell-killing, conformational and colloidal stabilities were explored. Unlike the previous reports where sY was also localized to the mAb variable regions, we found that sY decreased the mAb-X affinity for the TAA, increased its cell binding and killing EC50 values, and modestly increased its rate of aggregation.
Results
Sulfotyrosine identification and quantification
Liquid chromatography MS (LC/MS) analysis of the intact mAb-X revealed an approximate +80 Da mass adduct (Figure S1A of the Supporting Information) unaffected by treatment with the endoglycosidase PNGase F (Figure S1B) and consistent with the nominal mass increase expected for either Tyr phosphorylation or sulfation. Note that, although there is a 9.6 mDa mass difference between these two PTMs resulting from their difference in nuclear-binding energies,Citation24 this exceedingly small difference remains difficult to resolve using commercially available mass spectrometers.Citation12 For this reason, sY identification has historically been performed byCitation25S metabolic radiolabeling experiments and more recently by using anti-sulfotyrosine mAbs.Citation12 We distinguished Tyr sulfation from phosphorylation using a similar approach taken by Zhao et al.Citation18 by incubating mAb-X samples with lambda phosphatase () and, unique to this investigation, abalone sulfatase (). Only treatment with sulfatase resulted in the loss of the 80 Da modification, strongly suggesting the presence of sY (). The −80 Da neutral loss of this adduct observed during peptide mapping, described later, is also in agreement with this identification since phosphorylation is much more stable PTM and not nearly as susceptible to loss during ionization or fragmentation.Citation12,Citation13,Citation24
Figure 1. Deconvoluted mass spectra of intact mAb-X before (top) and after (bottom) incubation with lambda phosphatase (a) and abalone sulfatase (b). The ~ 80 Da mass adduct is only eliminated following the sulfatase treatment.

The sulfatase treated and non-treated mAb-X samples were next analyzed by cation exchange chromatography (CEX) to estimate the amount of this acidic PTM. Desulfation resulted in the loss of two CEX acidic pre-peaks and a corresponding increase of the main peak by roughly 21% (). The three CEX peaks from non-sulfatase treated material were then collected to greater than 90% purity (Figure S2A), and their identities determined by intact LC/MS (Figure S2B). The minor acidic peak eluting first was consistent in mass with mAb-X containing two sulfotyrosines (2sY) and the major acidic peak-eluting second was identified as mAb-X containing a single sulfotyrosine (1sY); the main peak was consistent in mass with the unsulfated mAb-X (0sY) (, Figure S2). The remaining material from the CEX fractions were used for peptide mapping and to determine the effect of sY on mAb-X binding, cell killing, and physical stability.
Figure 2. Cation exchange chromatography of mAb-X prior to abalone sulfatase incubation (black) and following incubation (red). Based on the integrated areas of the CEX peaks, approximately 21% of mAb-X is sulfated.

Sulfotyrosine localization
Tyrosine sulfation is an extremely labile modification that is lost in a neutral fashion due to proton-induced fragmentation of the sY sulfoester bond using even some of the gentler positive ion mode MS ionization methods commonly used within the biopharmaceutical industry, such as electrospray.Citation12 This loss is particularly pronounced for smaller peptides where fewer peptide backbone amide groups are available to capture excess protons.Citation12 Therefore, we initially narrowed down the location of sY by reduced LC/MS. Sulfation was observed on both the HC and HHC (, respectively) suggesting that it is either present in the heavy-chain portion of the Fab, termed the Fd, that binds to the TAA or in the CH2 or CH3 domains. Subsequent cleavage just below the hinge region with the endoprotease IdeS (Promega) followed by LC/MS ruled out this latter possibility (data not shown).
Figure 3. Deconvoluted mass spectrum of deglycosylated and reduced mAb-X. The ~80 Da adduct was only present on the heavy-heavy chain (a) and heavy chain (b) but not on the light chains (data not shown).

Residue-resolved localization of sY within the TAA Fd region was next attempted by peptide map analysis. Aside from the lability of this PTM during positive mode ionization, neutral loss of sY is particularly problematic using standard collision-induced disassociation (CID) fragmentation methods necessary for MS/MS sequencing since SO3 is lost at lower energies than what is required to break the peptide bond.Citation12,Citation26 To increase the likelihood of the sulfoester group retention within peptide fragment ions, ionization in the less commonly used negative ion mode has been used either with conventional CID or in combination with alternative fragmentation methods such as electron transfer and capture disassociation (ETD/ECD) described elsewhere.Citation12,Citation13,Citation24
For the level of resolution we required, sY was localized using conventional positive ion mode peptide mapping with CID fragmentation in combination with a multi-protease approach. Trypsin digestion of the CEX fractions first located sY within a 38 amino acid tryptic peptide that contains two Tyr triplet sequences (YYY) and resides within one of the TAA-binding Fab CDRs (). Digestion with chymotrypsin and AspN proteases next identified 16 and 4 amino acid length peptides, respectively, that narrowed down the sY location to the first Tyr triplet sequence of the tryptic peptide (); representative MS1 spectra of the 2sY, 1sY and 0sY CEX fractions digested with chymotrypsin are shown in , respectively. Note that the differences in relative % sulfonation between tryptic, chymotryptic and AspN peptides () are most likely due to different ionization efficiencies and lengths of the peptides. Targeted MS/MS analysis of the AspN peptide containing the YYY repeat suggested that sulfation resides on one of the flanking residues of the Tyr triplet; however, too few signature ions were present to unambiguously distinguish which of these two residues was sulfated (Figure S3 of the Supporting Information).
Figure 4. Extracted ion chromatograms of the 16 amino acid chymotryptic peptide containing the YYY sequence that resides within one of the HC and HHC CDRs of the TAA binding Fab for the 2sY (a), 1sY (b) and 0sY (c) CEX fractions. The peak areas for the unmodified peptides (top panels) and sulfated peptides (bottom panels) were used to calculate the relative percentage of Tyr sulfation (). Note that these peptides are double charged, and that RT, MA and BP represent their retention times, areas, and mass, respectively.

Table 1. Percent relative sulfonation of the CEX fractions.
The percent relative sulfonation was calculated using the extracted-ion chromatograms (XICs) of the sulfonated peptide divided by XIC of the sum of modified and unmodified peptide.
The impact of tyrosine sulfation on binding
An obvious concern with PTMs such as sulfation occurring within the variable regions of mAbs is their potential impact on binding and efficacy. To test this possibility, surface plasmon resonance (SPR) was used to monitor the association and disassociation kinetics of soluble TAA to the immobilized 0sY, 1sY and 2sY mAb-X CEX fractions. Representative sensorgrams of antigen injected at incrementally increasing concentrations to the immobilized 0sY fraction using the multi-cycle method and globally fit to a 1:1 binding modelCitation27,Citation28 are shown in Figure S4 of the supporting information. The equilibrium disassociation constants (KD) derived from the SPR association (ka), and disassociation (kd) rate constants decrease in affinity with sulfation; however, we note that the fits to this model were less than optimal judging by the spread and pattern of the residuals (Figure S4), which we believe is due to minor heterogeneity of the soluble TAA as determined by size-exclusion chromatography (SEC; data not shown).
Due to the quality of the SPR fits, sensorgrams of the sY CEX fractions were also analyzed using a model-independent sensorgram comparison method () developed by R. Karlsson et al. and described in detail elsewhere.Citation29 Briefly, six independent sensorgrams of the binding and disassociation phases of soluble TAA at five concentrations were collected for the 0sY sample and averaged. A two-standard deviation (SD) window around this average was then generated (upper and lower limits) from which a sensorgram similarity score (SSS) was calculated for the average of triplicate 1sY and 2sY sensorgrams based on their percentage of data points inside the ±2-SD window (%DPI) and the percentage of their data points outside of this window (%DPO) weighted by their distance to the 0sY average value according to EquationEq 1(1)
(1) .Citation29 Using this method, the similarity scores for mAb-X drop by roughly half with 1sY and by another 50% for 2sY.
Figure 5. Binding and disassociation kinetics of the mAb-X sY CEX fractions measured by SPR. The SPR sensorgram comparison (a) of the binding and disassociation kinetics of the TAA at incrementally increasing concentrations to the mAb-X 0sY (black), 1sY (blue) and 2sY (green) species demonstrates that sulfation predominantly impacts TAA disassociation; red traces are the ± 2-standard deviation values of the average of six independent 0sY sensorgrams. Representative SPR kinetic traces for the 40.5 nM TAA disassociation phase (b) using the same color scheme for the sY species described for panel A. The global fit of the three sY species at all five TAA concentrations to a one exponential and the sum of two exponential equations are overlaid in red and black, respectively. Note that time is plotted on a log scale for better visualization of the fits at the earlier time-points.

As shown in , sulfation decreases mAb-X TAA binding predominantly by increasing the rate of TAA disassociation. To quantify this increase, only the disassociation phases of the sensorgrams were globally fit to Eq. 2 linking the two disassociation rate constants (kd1 and kd2) across the averaged sensorgrams for the 0sY, 1sY and 2sY species at all five antigen concentrations.
In EquationEq 2(2)
(2) , Δy1 and Δy2 are the amplitudes or total change in the sensorgram response units (RU) for each disassociation phase and y∞ is the final RU signal. Note that the averaged sensorgrams of the 0sY species were adequately fit to a single exponential by fixing Δy2 in the global fit to zero. The quality of these fits was excellent by the same criteria previously stated and resulted in kd1 and kd2 values of 1.44 × 10−3 s−1 (±7×10−6) and 9.18 × 10−3 s−1 (±2×10−5) for the non-sulfated and sulfated species, respectively. For clarity, only the disassociation data for the highest soluble TAA concentration tested is shown in .
The impact of tyrosine sulfation on cell binding and killing
Target cell binding and T cell-mediated killing of a tumor cell line expressing the TAA was next investigated for the mAb-X sY fractions. For the cell-binding experiments, the mAb-X sY fractions were incubated at incrementally increasing concentrations with a Mino cell line expressing the TAA and separately with donor pan T cells expressing the TSA; binding was quantified by flow cytometry using a fluorescently labeled secondary antibody. Similar to the SPR experiments, tyrosine sulfation affected binding. The half maximal effective concentration (EC50) values increased from 1.5 nM for the 0sY fraction to 3.7 and 4.6 nM for the 1sY and 2sY fractions, respectively; the maximum cell binding of all sY species were similar (). As expected, tyrosine sulfation had no apparent impact on T-cell binding (data not shown).
Figure 6. Mino cell binding (a) and T cell-mediated Jeko tumor cell killing (b) of the 0sY (black), 1sY (blue) and 2sY (green) mAb-X CEX fractions measured by flow cytometry. EC50 values for cell binding (killing) were 1.5 (0.06), 3.7 (0.16) and 4.6 (0.19) nM for the 0sY, 1sY and 2sY species, respectively.

For the in-vitro antitumor cell activity experiments, the fractionated mAb-X sY species were similarly incubated at increasing concentrations with a co-culture of Jeko-1 cells expressing the TAA and normal healthy donor pan T cells at 2:1 effector-to-target ratio for 48 h. Following incubation, the percentage of dead Jeko-1 cells expressing the TAA was determined by flow cytometry. As shown in , all of the sY fractioned species showed potent T-cell killing of the target tumor cell line; however, the EC50 values for the 0sY species were 2.7 and 3.2-fold lower than for the 1sY and 2sY species, respectively. The overall maximum depth of kill was comparable between all the sY species.
Tyrosine sulfation and physical stability
Aside from the clear impact that mutations or PTMs occurring within the CDR regions of mAbs may have on binding, their potential effect on conformational and colloidal stability should not be overlooked. For instance, Dudgeon et al. have shown that mutations altering charge (which sulfation does) within or flanking mAb CDRs modulate colloidal stability as manifested by altered aggregation and particulation rates.Citation30 Another striking example is the cysteinylation of an unpaired sulfhydryl within the CDR3 for an IgG1 mAb.Citation31 In addition to lowering this mAb’s biological activity, this single PTM significantly reduced the Fab apparent melting temperature (Tmapp) and accelerated its rate of aggregation.Citation31 To understand if the mAb-X physical stability is impacted by Tyr sulfation, the conformational and colloidal stabilities of the 0sY, 1sY and 2sY CEX fractions were assessed and compared to their rates of aggregation during short-term static storage.
As typical for mAbs, thermal and chemical denaturant unfolding of mAb-X was an irreversible process that prohibited a true comparison of the conformational stabilities (the difference in free energy between the native and unfolded-states) of the sY species. Instead, differential scanning calorimetry (DSC) was used to compare the thermal unfolding transitions of the three sY species, a potentially important parameter for predicting aggregation propensity if mAb-X aggregates through a non-native pathway even at the lower temperatures used for drug product manufacturing and storage. Representative thermograms of the mAb-X sY species collected at the same heating rate are overlaid in and show no apparent difference in Tmapp values.
Figure 7. Sulfation had no apparent impact on thermal unfolding transitions of mAb-X as measured by DSC (a) but did marginally decrease its colloidal stability as assessed by PEG induced LLPS (b) and increase the rate of mAb-X aggregation during short-term static storage at 40°C (c). The 0sY, 1sY and 2sY species are shown in black, blue, and green, respectively.

In contrast to thermal stability, the native-state colloidal stabilities of numerous mAbs, assessed using a variety of techniques ranging from static and dynamic light scattering methods to simple solubility measurements, often correlate reasonably well with their solution behaviors such as opalescence, viscosity and aggregation propensity.Citation25,Citation32–35 In this investigation, polyethylene glycol (PEG) was used as an inert macromolecular crowding agent to induce liquid–liquid phase separation (LLPS) for the purpose of ranking the colloidal stabilities of the sY fractions. The mechanistic details of macromolecular crowding can be found elsewhere,Citation36 including its use to calculate protein–protein binding free energies.Citation37 For our purposes, a simple PEG-6000 titration of the mAb- sY fractions was performed and the remaining protein in the protein “poor” or lean phase following LLPS was compared. As shown in , sulfation slightly lowered the percentage of PEG-6000 required to induce LLPS indicating lower colloidal stability or increase in protein–protein interaction.
The CEX fractions of the sY species were next concentrated to 10 mg/mL and stored for 1 month at 40°C (25°C below the onset of thermal unfolding, ) during which time aliquots were withdrawn and analyzed by SEC. As shown in , sulfation increased the rate of mAb-X high molecular weight (HMW) species growth similar to its impact on colloidal stability. Although the amounts of HMW species were relatively low, the aggregation rates of the sY species would be expected to increase, and their differences magnified had they been stored at the higher concentrations (~100 mg/mL) typical for therapeutic mAb formulations. This was not possible, however, due to material constraints of the mAb-X sY species.
Discussion
Given that some 1% of all Tyr residues within the eukaryotic proteome are thought to be sulfated,Citation12 it is surprising that tyrosine sulfation of more CHO-derived protein therapeutics have not been reported. We speculate that this is largely due to the extreme lability of this PTM under the conditions of many ionization and fragmentation methods commonly used within the biopharmaceutical industry for MS-based characterization, and perhaps in some instances due to the misidentification of sY as phosphotyrosine. Since this common PTM may directly impact biological efficacy and limit shelf-life, as demonstrated here, sY identification, characterization, and quantitation using orthogonal non-MS-based methodologies amenable to high-throughput automation such as plate-based desulfation/CEX or the use of anti-sY mAbs may be attractive areas for further development. Residue resolved localization of sY could then be reserved for the more time- and resource-consuming peptide mapping using alternative MS/MS fragmentation methods such as ETD, EAD, ETciD, and EThcD. Toward this goal, a hydrophobic interaction chromatography (HIC) method was recently developed and shown to effectively separate multiple tyrosine-sulfated proteoforms of an anti-HIV-1 mAb that showed increased antigen binding with higher amounts of Fab tyrosine sulfation.Citation38
In addition to the further development of sY identification and localization methods, strategies for managing or limiting this PTM are needed, not only due to the potential risk of sY becoming a critical quality attribute, but also to ensure batch-to-batch consistency and the fulfillment of acceptance criteria that often include the use ion-exchange chromatography as an identity method prior to drug substance release. In the case of mAb-X, drug substance could be consistently manufactured with nearly identical low levels of sY that showed comparable binding and stability profiles as the 0sY fractioned species. In cases where Tyr sulfation cannot be as consistently managed, other means of eliminating this PTM are needed. Towards this end, recent studies have worked to refine the existing, and develop new in-silico, tools for predicting which Tyr residues are susceptible to sulfation so that appropriate substitutions can be made at the earliest stages of development, when cell line and culture conditions are beginning to be optimized to limit TPST activity.Citation19,Citation39–41
Materials and methods
PNGase F, phosphatase, and sulfatase treatment
The mAb-X N-linked glycans were removed by incubating 1 mg/mL of mAb-X buffered by 50 mM Tris-HCl (pH 7.5) with PNGase F (Promega, Catalog # V4831) at a final enzyme concentration of 1 U/µL. Samples were incubated overnight at 37°C prior to intact analysis by LC/MS (Figure S1). The deglycosylated mAb-X samples were also analyzed by reduced LC/MS () after buffer exchange into 7 M guanidinium hydrochloride (MP Biomedicals), 100 mM Tis-HCl (pH 8.0), and 20 mM dithiothreitol (Sigma-Aldrich) and incubation for 45 min at 37°C.
Distinction between Try phosphorylation and sulfation was made by treating mAb-X with lambda protein phosphatase (New England Biolabs, Catalog # P0753S) and abalone sulfatase (Millipore Sigma, Catalog # S9754). For the phosphatase treatment, 8 µL of lambda protein phosphatase (400 U/µL) was added to a 50 µL mixture consisting of 20 µg of mAb-X, 50 mM Tris-HCl (pH 7.5), 100 mM NaCl, 0.01% (w/v) polysorbate-80, and 1 mM MnCl2 and incubated overnight at 30°C. Desulfation experiments were performed by diluting mAb-X to 1 mg/mL in a 100 mM sodium acetate (pH 5.0), 100 mM NaCl buffer solution to which abalone sulfatase was added to a final concentration of 15 U/mL. Samples were incubated for 2 h at 37°C.
Intact and reduced LC/MS
Reversed-phase separation of all mAb-X samples was performed on an Agilent (Palo Alto, CA) 1200 UltraPerformance liquid chromatography (UPLC) system equipped with a Waters (Milford, MA) BioResolve RP mAb Polyphenyl, 420 Å, 2.6 μm, 2.1 × 150 mm column. Twenty micrograms of the mAb-X samples were injected and eluted using a gradient consisting of 100% solvent A (20% acetonitrile, 5% butanol) ramped to 80% solvent B (50% acetonitrile, 5% butanol) over a 12-min time period using a 0.5 mL/min flow rate. Prior to returning the column to 100% solvent A the column was washed for 1 min with 94% solvent C (95% acetonitrile, 5% butanol) and 6% solvent A. The column temperature was maintained at 80°C and detection was monitored at 215 nm.
Mass spectrometric analysis was performed on an Agilent (Palo Alto, CA) 6230B time-of-flight (TOF) mass spectrometer using an electrospray ionization source in positive ion mode. The desolvation and source temperatures were set at 450°C and 80°C, respectively, while the capillary and cone voltages were set at 3200 and 30 V, respectively. All the other voltages were optimized to provide maximal signal intensity. All raw data were processed using the Protein Metrics (Cupertino, CA) software to obtain the deconvoluted average mass (±40 ppm).
Peptide mapping
The mAb-X sY CEX fractions were diluted to 1.0 mg/mL with 100 mM Tis-HCl (pH 7.5), denatured in the presence of 0.5% Rapigest surfactant (Waters), reduced with dithiothreitol (Sigma-Aldrich), and alkylated with iodoacetamide (Sigma-Aldrich). Samples were then divided into thirds and digested with either trypsin (Promega, Catalog # VA9000), chymotrypsin (Promega, Catalog # V1061) and AspN (Promega, Catalog # V1621) in protease to mAb-X molar ratios of 1:10, 1:20 and 1:50, respectively. Following overnight 37°C incubation the digests were quenched by addition of trifluoroacetic acid. Reversed-phase separation was performed in on a Waters Acquity UPLC (Waters, Milford, MA); 0.8 µg of each sample was injected onto a Waters BEH C18, 2.1 × 150 mm, 130 Å, 1.7 μm particle size column (Waters, Milford, MA) and eluted using a linear gradient from 0.2% to 30% (Mobile phase B) in 47 min. Mobile phase A consisted of 0.1% formic acid in water and mobile phase B was 0.1% formic acid, 99.9% acetonitrile. The column temperature was maintained at 50°C and the flow rate was kept at 0.2 mL/min. Data were acquired in positive mode on QExactive Plus mass spectrometer (Thermo Fisher Scientific) with a m/z range was set to 320–1800, with maximum injection time of 100 ms for full scan, the S lens was kept at 50. High-resolution data were acquired in data-dependent acquisition mode using high-energy collisional dissociation (HCD) for fragmentation of the 10 most abundant ions; resolution for full scan was set to 70,000, while resolution for MS2 was kept at 17,500. Acquired data was searched using Byologic software (Protein Metrics, Cupertino, CA) and manually verified. Targeted analysis was run separately focusing on m/z of peptides of interest.
CEX chromatography
CEX chromatography was performed on an Agilent Infinity 1260 LC system (Agilent, Palo Alto, CA) equipped with an analytical fraction collector. Twenty micrograms of mAb-X was injected neat onto a Thermo MAbPac SCX-10, 4 × 250 mm analytical column (Thermo Fisher Scientific) maintained at 25°C and equilibrated with the Thermo CX-1 pH gradient buffer A (pH 5.6) and eluted over 30 min using a linear gradient of the Thermo CX-1 buffer B (pH 10.2). In the first 2 min of the gradient the percentage of buffer B was increased to 30%, over the next 25 min the gradient was ramped to 40% to elute all peaks. Note that for fraction collection purposes up to 130 µg of mAb-X was injected and a slightly shallower pH gradient was used. Absorbance was monitored at 215 and 280 nm. Following fraction collection the mAb-X sY fractions were buffer exchanged into 20 mM L-Histidine/L-Histidine-HCl (pH 6.0) buffer.
Surface plasmon resonance
The binding and disassociation kinetics of the mAb-X sY CEX fraction were analyzed on a Biacore T200 instrument (Cytiva, Marlborough, MA). The sensor surface of a CM5 series s chip was activated with 750 mg of 1-ethyl-3-(3-dimethylaminopropyl)carbodiimide hydrochloride (EDC) and 115 mg of N-hydroxysuccinimide (NHS). Following activation, 20 µg/ml of anti-human IgG Fc (Invitrogen, Catalog # 12-4998-82) was then diluted in running buffer (0.01 M HEPES, 0.15 NaCl, 3 mM EDTA, 0.005% Tween-20) and injected at a 10 µl/min flow rate for 420 s to reach an immobilization level of about 10,000 RU on all four channels; an inactivation of the surface step was then included by injecting ethanolamine-HCl pH 8.5. Binding of the soluble TAA (CD33: CD33 antigen, Arco Biosystems, Catalog # CDD-82W6; FcRH5: Anti-human IgG (Fc) antibody, GE, Catalog# BR-1008-39) to the mAb-X sY CEX fractions was performed using a multi-cycle method. mAb-X fractions were diluted in the same running buffer to 0.1 µg/ml and 0.5 µg/mL and injected on the surface at flow rate of 10 µl/min for 60 s. The soluble TAA was diluted to six concentrations (40.5, 13.5, 4.5, 1.5, 0.5 and 0 nM) and was then injected on all 4 channels (channel 1 as reference) at a flow rate of 30 µl/min for an association phase of 300 s, followed by 600 s of dissociation. Six cycles of running analyte were repeated according to analyte concentrations in ascending order. After each cycle of interaction analysis, the sensor chip surface is regenerated completely with 3 M MgCl2 at a flow rate of 30 μL/min for 30 s.
In-vitro tumor cell binding
Cell binding of the mAb-X was investigated by incubating each of the CEX sY fractions at incrementally increasing concentrations with a Mino cell line expressing the TAA at a concentration of 50,000 cells per well. Following a 45-min incubation with the sY fractions, cells were incubated with a fluorescently labeled anti-human AF647 secondary antibody (Jackson ImmunoResearch, West Grove, PA, Catalog # 109-545-003) for 30 min at room temperature prior to quantification by flow cytometry using a Becton Dickson (Franklin Lakes, NJ) FACS Canto flow cytometer.
In-vitro antitumor activity
T cell-dependent antitumor activity was assessed by co-incubating Jeko-1 tumor cells expressing the TAA (10,000/well) with donor pan T cells (STEMCELL Technologies, Vancouver, Canada) (20,000/well) in the presence of incrementally increasing concentrations of the mAb-X sY CEX fractions for 48 h at 37°C in media supplemented with 5% CO2. Following incubation, the percentage of dead tumor cells was calculated using a live/dead fixable stain (Thermo Fisher Scientific, Waltham, MA) and a Becton Dickson (Franklin Lakes, NJ) FACS Canto flow cytometer.
Differential scanning calorimetry
DSC was performed on a TA Instruments NanoDSC (New Castle, DE) using the NanoAnalyze software. The mAb-X sY CEX fractions were buffer exchanged into 20 mM L-histidine/L-histidine-HCl (pH 6.0) and adjusted to 1 mg/mL concentrations. Prior to analysis, the samples and buffer without protein were degassed for 30 min. Samples and buffer were heated from 10°C to 100°C at a rate of 1°C⋅min−1. Following acquisition, the buffer was subtracted from each of the samples and the data were normalized to convert to kJ⋅mol−1⋅°C−1. For each thermal unfolding transition, a Gaussian peak was fit, and the mode was reported as the Tmapp.
PEG precipitation
PEG precipitation experiments were performed by preparing 200 µL solutions consisting of 1.0 mg/mL of each of the mAb-X sY CEX fractions, 20 mM L-histidine/L-histidine-HCl (pH 6.0) and incrementally increasing concentrations of PEG-6000. The solutions were placed at 4°C overnight and then centrifuged for 60 min at 25,000 RCF. The amount of mAb-X remaining in the supernatant was then measured by absorbance at 280 nm using an Agilent Cary UV-8454 (Agilent, Palo Alto, CA). Data were fit to the empirical four-parameter sigmoidal equation to guide the eye.
Abbreviations
CDR | = | complementary determining region |
CEX | = | cation exchange chromatography |
CH2 | = | second constant heavy chain domain |
CH3 | = | third constant heavy chain domain |
CHO | = | Chinese Hamster Ovary |
CID | = | collision-induced disassociation |
DSC | = | differential scanning calorimetry |
EC50 | = | half maximal effective concentration |
ETD/ECD | = | electron transfer/capture disassociation |
Fab | = | fragment antigen binding |
Fd | = | N-terminal half of the heavy chain that comprises the Fab |
Fc | = | fragment crystallizable |
HC | = | heavy chain |
HHC | = | heavy-heavy chain |
HMW | = | high molecular weight species |
ka | = | association rate constant |
kd | = | disassociation rate constant |
KD | = | equilibrium disassociation constant |
LC/MS | = | liquid chromatography mass spectrometry |
LLPS | = | liquid-liquid phase separation |
mAb | = | monoclonal antibody |
MS | = | mass spectrometry |
PEG | = | polyethylene glycol |
PTM | = | post-translational modification |
SD | = | standard deviation |
SEC | = | size exclusion chromatography |
SPR | = | surface plasmon resonance |
SSS | = | sensogram similarity score |
TAA | = | tumor associated antigen |
TPSTs | = | tyrosylprotein sulfotransferases |
Tmapp | = | apparent melting temperature |
TSA | = | T-cell specific antigen |
Tyr | = | tyrosine |
sY | = | tyrosine sulfation |
Supplemental Material
Download MS Word (897.8 KB)Acknowledgments
The authors report there are no competing interests to declare.
Disclosure statement
No potential conflict of interest was reported by the author(s).
Supplementary material
Supplemental data for this article can be accessed online at https://doi.org/10.1080/19420862.2023.2259289
Additional information
Funding
References
- Maggon K. Monoclonal antibody “gold rush”. Curr Med Chem. 2007;14:1978–11. doi:10.2174/092986707781368504.
- Projan SJ, Gill D, Lu Z, Herrmann SH. Small molecules for small minds? The case for biologic pharmaceuticals. Expert Opin Biol Ther. 2004;4(8):1345–50. doi:10.1517/14712598.4.8.1345. PMID: 15268667.
- Carter PJ, Lazar GA. Next generation antibody drugs: pursuit of the ‘high-hanging fruit’. Nat Rev Drug Discov. 2017;17:197–223. doi:10.1038/nrd.2017.227.
- Hermeling S, Crommelin DJA, Schellekens H, Jiskoot W. Structure-immunogenicity relationships of therapeutic proteins. Pharm Res. 2004;21(6):897–903. doi:10.1023/B:PHAM.0000029275.41323.a6.
- Maas C, Hermeling S, Bouma B, Jiskoot W, Gebbink MFBG. A role for protein misfolding in immunogenicity of biopharmaceuticals. J Biol Chem. 2007;282(4):2229–36. doi:10.1074/jbc.M605984200.
- Vlasak J, Ionescu R. Fragmentation of monoclonal antibodies. MAbs. 2011;3(3):253–63. doi:10.4161/mabs.3.3.15608. PMID: 21487244.
- Wang W, Singh S, Zeng DL, King K, Nema S. Antibody structure, instability, and formulation. J Pharm Sci. 2007;96(1):1–26. doi:10.1002/jps.20727. PMID: 16998873.
- Gupta S, Jiskoot W, Schöneich C, Rathore AS. Oxidation and deamidation of monoclonal antibody products: potential impact on stability, biological activity, and efficacy. J Pharm Sci. 2022;111(4):903–18. doi:10.1016/j.xphs.2021.11.024. PMID: 34890632.
- Liu H, Nowak C, Andrien B, Shao M, Ponniah G, Neill A. Impact of IgG Fc-oligosaccharides on recombinant monoclonal antibody structure, stability, safety, and efficacy. Biotechnol Prog. 2017;33(5):1173–81. doi:10.1002/btpr.2498. PMID: 28547754.
- Gadgil HS, Bondarenko PV, Pipes GD, Dillon TM, Banks D, Abel J, Kleemann GR, Treuheit MJ. Identification of cysteinylation of a free cysteine in the fab region of a recombinant monoclonal IgG1 antibody using lys-C limited proteolysis coupled with LC/MS analysis. Anal Biochem. 2006;355(2):165–74. doi:10.1016/j.ab.2006.05.037. PMID: 16828048.
- McSherry T, McSherry J, Ozaeta P, Longenecker K, Ramsay C, Fishpaugh J, Allen S. Cysteinylation of a monoclonal antibody leads to its inactivation. MAbs. 2016;8(4):718–25. doi:10.1080/19420862.2016.1160179. PMID: 27050640.
- Seibert C, Sakmar TP. Toward a framework for sulfoproteomics: synthesis and characterization of sulfotyrosine-containing peptides. Peptide Science. 2008;90(3):459–77. doi:10.1002/bip.20821.
- Monigatti F, Hekking B, Steen H. Protein sulfation analysis—a primer. Biochimica Et Biophysica Acta (BBA) - Prot Proteom. 2006;1764(12):1904–13. doi:10.1016/j.bbapap.2006.07.002.
- Stone MJ, Chuang S, Hou X, Shoham M, Zhu JZ. Tyrosine sulfation: an increasingly recognised post-translational modification of secreted proteins. N Biotechnol. 2009;25(5):299–317. doi:10.1016/j.nbt.2009.03.011.
- Beisswanger R, Corbeil D, Vannier C, Thiele C, Dohrmann U, Kellner R, Ashman K, Niehrs C, Huttner WB. Existence of distinct tyrosylprotein sulfotransferase genes: molecular characterization of tyrosylprotein sulfotransferase-2. Proc Natl Acad Sci USA. 1998;95(19):11134–39. doi:10.1073/pnas.95.19.11134.
- Huttner WB. Tyrosine sulfation and the secretory pathway. Annu Rev Physiol. 1988;50(1):363–76. doi:10.1146/annurev.ph.50.030188.002051. PMID: 3288098.
- Baeuerle PA, Huttner WB. Tyrosine sulfation of yolk proteins 1, 2, and 3 in drosophila melanogaster. J Biol Chem. 1985;260(10):6434–39. doi:10.1016/S0021-9258(18)88991-8. PMID: 3922974.
- Zhao J, Saunders J, Schussler SD, Rios S, Insaidoo FK, Fridman AL, Li H, Liu YH. Characterization of a novel modification of a CHO-produced mAb: evidence for the presence of tyrosine sulfation. MAbs. 2017;9(6):985–95. doi:10.1080/19420862.2017.1332552. PMID: 28590151.
- Tyshchuk O, Gstöttner C, Funk D, Nicolardi S, Frost S, Klostermann S, Becker T, Jolkver E, Schumacher F, Koller CF, et al. Characterization and prediction of positional 4-hydroxyproline and sulfotyrosine, two post-translational modifications that can occur at substantial levels in CHO cells-expressed biotherapeutics. MAbs. 2019;11(7):1219–32. doi:10.1080/19420862.2019.1635865.
- Klein C, Schaefer W, Regula JT, Dumontet C, Brinkmann U, Bacac M, Umaña P. Engineering therapeutic bispecific antibodies using CrossMab technology. Methods. 2019;154:21–31. doi:10.1016/j.ymeth.2018.11.008. PMID: 30453028.
- Surowka M, Schaefer W, Klein C. Ten years in the making: application of CrossMab technology for the development of therapeutic bispecific antibodies and antibody fusion proteins. MAbs. 2021;13(1):1967714. doi:10.1080/19420862.2021.1967714. PMID: 34491877.
- Klein C, Schaefer W, Regula JT. The use of CrossMAb technology for the generation of bi- and multispecific antibodies. MAbs. 2016;8(6):1010–20. doi:10.1080/19420862.2016.1197457. PMID: 27285945.
- Bacac M, Klein C, Umana P. CEA TCB: a novel head-to-tail 2: 1 T cell bispecific antibody for treatment of CEA-positive solid tumors. Oncoimmunol. 2016;5(8):e1203498. doi:10.1080/2162402x.2016.1203498. PMID: 27622073.
- Chen G, Zhang Y, Trinidad JC, Dann C. Distinguishing sulfotyrosine containing peptides from their phosphotyrosine counterparts using mass spectrometry. J Am Soc Mass Spectrom. 2018;29(3):455–62. doi:10.1007/s13361-017-1854-1.
- Valente JJ, Payne RW, Manning MC, Wilson WW, Henry CS. Colloidal behavior of proteins: effects of the second virial coefficient on solubility, crystallization and aggregation of proteins in aqueous solution. Curr Pharm Biotechnol. 2005;6(6):427–36. doi:10.2174/138920105775159313. PMID: 16375727.
- Yagami T, Kitagawa K, Aida C, Fujiwara H, Futaki S. Stabilization of a tyrosine O-sulfate residue by a cationic functional group: formation of a conjugate acid-base pair. J Pept Res. 2000;56(4):239–49. doi:10.1034/j.1399-3011.2000.00746.x. PMID: 11083063.
- Oshannessy DJ, Brighamburke M, Soneson KK, Hensley P, Brooks I. Determination of rate and equilibrium binding constants for macromolecular interactions using surface plasmon resonance: use of nonlinear least squares analysis methods. Anal Biochem. 1993;212(2):457–68. doi:10.1006/abio.1993.1355.
- O’Shannessy DJ, Brigham-Burke M, Karl Soneson K, Hensley P, Brooks I. Determination of rate and equilibrium binding constants for macromolecular interactions by surface plasmon resonance. In: Part B: numerical computer methods. Vol. 240. Elsevier; 1994. pp. 323–49. doi:10.1016/S0076-6879(94)40054-7.
- Karlsson R, Pol E, Frostell Å. Comparison of surface plasmon resonance binding curves for characterization of protein interactions and analysis of screening data. Anal Biochem. 2016;502:53–63. doi:10.1016/j.ab.2016.03.007. PMID: 27019155.
- Dudgeon K, Rouet R, Kokmeijer I, Schofield P, Stolp J, Langley D, Stock D, Christ D. General strategy for the generation of human antibody variable domains with increased aggregation resistance. Proc Natl Acad Sci USA. 2012;109(27):10879–84. doi:10.1073/pnas.1202866109.
- Banks DD, Gadgil HS, Pipes GD, Bondarenko PV, Hobbs V, Scavezze JL, Kim J, Jiang XR, Mukku V, Dillon TM. Removal of cysteinylation from an unpaired sulfhydryl in the variable region of a recombinant monoclonal IgG1 antibody improves homogeneity, stability, and biological activity. J Pharm Sci. 2008;97(2):775–90. doi:10.1002/jps.21014. PMID: 17786988.
- Banks DD, Latypov RF, Ketchem RR, Woodard J, Scavezze JL, Siska CC, Razinkov VI. Native-state solubility and transfer free energy as predictive tools for selecting excipients to include in protein formulation development studies. J Pharm Sci. 2012;101(8):2720–32. doi:10.1002/jps.23219.
- Banks DD, Cordia JF, Spasojevic V, Sun J, Franc S, Cho Y. Isotonic concentrations of excipients control the dimerization rate of a therapeutic immunoglobulin G1 antibody during refrigerated storage based on their rank order of native-state interaction. Protein Sci. 2018;27(12):2073–83. doi:10.1002/pro.3518.
- Kingsbury JS, Saini A, Auclair SM, Fu L, Lantz MM, Halloran KT, Calero-Rubio C, Schwenger W, Airiau CY, Zhang J, et al. A single molecular descriptor to predict solution behavior of therapeutic antibodies. Sci Adv. 2020;6(32):eabb0372. doi:10.1126/sciadv.abb0372.
- Quigley A, Williams DR. The second virial coefficient as a predictor of protein aggregation propensity: a self-interaction chromatography study. Eur J Pharm Biopharm. 2015;96:282–90. doi:10.1016/j.ejpb.2015.07.025. PMID: 26259782.
- Minton AP. Influence of macromolecular crowding upon the stability and state of association of proteins: predictions and observations. J Pharm Sci. 2005;94(8):1668–75. doi:10.1002/jps.20417.
- Wang Y, Latypov RF, Lomakin A, Meyer JA, Kerwin BA, Vunnum S, Benedek GB. Quantitative evaluation of colloidal stability of antibody solutions using PEG-induced liquid–liquid phase separation. Mol Pharmaceut. 2014;11(5):1391–402. doi:10.1021/mp400521b.
- Cai CX, Doria-Rose NA, Schneck NA, Ivleva VB, Tippett B, Shadrick WR, O’Connell S, Coooper JW, Schneiderman Z, Zhang B, et al. Tyrosine O-sulfation proteoforms affect HIV-1 monoclonal antibody potency. Sci Rep. 2022;12(1):8433–44. doi:10.1038/s41598-022-12423-x.
- Yang ZR. Predicting sulfotyrosine sites using the random forest algorithm with significantly improved prediction accuracy. BMC Bioinform. 2009;10(1):361. doi:10.1186/1471-2105-10-361. PMID: 19874585.
- Chang WC, Lee TY, Shien DM, Hsu JB, Horng JT, Hsu PC, Wang TY, Huang HD, Pan RL. Incorporating support vector machine for identifying protein tyrosine sulfation sites. J Comput Chem. 2009;30(15):2526–37. doi:10.1002/jcc.21258. PMID: 19373826.
- Liu R, Zhang Y, Kumar A, Huhn S, Hullinger L, Du Z. Modulating tyrosine sulfation of recombinant antibodies in CHO cell culture by host selection and sodium chlorate supplementation. Biotechnol J. 2021;16(9):2100142. doi:10.1002/biot.202100142.