ABSTRACT
A diverse array of commensal microorganisms inhabits the human intestinal tract. The most abundant and most studied members of this microbial community are undoubtedly bacteria. Their important role in gut physiology, defense against pathogens, and immune system education has been well documented over the last decades. However, the gut microbiome is not restricted to bacteria. It encompasses the entire breadth of microbial life: viruses, archaea, fungi, protists, and parasitic worms can also be found in the gut. While less studied than bacteria, their divergent but important roles during health and disease have become increasingly more appreciated. This review focuses on these understudied members of the gut microbiome. We will detail the composition and development of these microbial communities and will specifically highlight their functional interactions with enteric pathogens, such as species of the family Enterobacteriaceae. The interactions can be direct through physical interactions, or indirect through secreted metabolites or modulation of the immune response. We will present general concepts and specific examples of how non-bacterial gut communities modulate bacterial pathogenesis and present an outlook for future gut microbiome research that includes these communities.
Introduction
The gut microbiota and its importance for health and disease is now widely accepted by scientists and has permeated into common knowledge within the general lay population. Over the past decades, a considerable amount of research has been performed to characterize the composition and functions of gut commensal communities in humans, but also in various other multicellular organisms. Gut microbes are critical to human health and their functions are highly diverse. They range from the production of nutrients for colonocytes to the modulation of critical brain functions and have been extensively reviewed elsewhere.Citation1–5 This review focuses on neglected microbiome members and their interactions with enteric bacterial pathogens.
Enteric bacterial pathogens
In this review, we will focus on one particular function of the gut microbiota: colonization resistance and modulation of the pathogenesis of enteric bacterial pathogens. These pathogens are often food- or water-borne and infect the gastrointestinal tract, frequently causing diarrhea and gut inflammation.Citation6 Enteric bacterial pathogens are a highly diverse group that includes Gram positive and Gram negative bacteria. Common Gram positive enteric pathogens are Clostridium difficile, an anaerobic gut microbe, and Enterococcus faecalis, a facultative anaerobic bacterium. Both can expand after antibiotic treatmentCitation7,Citation8 and cause difficult to treat gastrointestinal infections. The Gram negative bacteria Campylobacter jejuni and Vibrio cholerae are examples of important food- and water borne illnesses. However, the bacterial family that is particularly associated with infections of the gut are Enterobacteriaceae. They include species of genera such as Salmonella, Escherichia, Enterobacter, Citrobacter, Yersinia, and Shigella. Many of these pathogens cause infections that are restricted to the gut and are self-limiting, such as non-typhoidal serovars of Salmonella enterica (e.g. Typhimurium), Citrobacter rodentium, different pathotypes of E. coli, and Shigella species. Others, such as Salmonella enterica serovar Typhi can also cause systemic infections.Citation9 In order to colonize the gut environment, enteric bacterial pathogens must effectively compete with the members of the gut microbiome.Citation10–13
Bacterial microbiome and enteric pathogens
The role of the gut microbiota in the pathogenesis of enteric pathogens has been well documented in numerous research articlesCitation14–19 and summarized in many review articles.Citation20–24 However, these publications focus on only one component of the microbiome: bacteria. Actinobacteria, Bacteroidetes, Firmicutes, Proteobacteria, and VerrucomicrobiaCitation25 are important members of the human gut microbiome. These gut commensal bacteria have numerous functions in the intestines and are integral for gut metabolism and immune priming during development. Importantly, they provide colonization resistance to pathogens. Relevant to interactions discussed in this review is for example the breakdown of carbohydrates from the diet by fermentative bacteria into short-chain fatty acids (SCFAs). SCFAs are consumed by epithelial cells but also serve to inhibit bacterial virulence factors. Gut commensal bacterial metabolism thus provides protection against pathogens.Citation26 Other mechanisms of how gut bacteria inhibit enteric pathogens include competition for space and for specific nutrients.Citation27
Other microbiome members
The bacteria-centric view of the microbiome has broadened in recent years to include other organisms that inhabit the gastrointestinal tract. We now recognize that the microbiome consists of not just bacteria, but also of other prokaryotes (archaea), eukaryotes (fungi, protozoa, parasitic worms), and viruses (archaeal, bacterial and eukaryotic) (). While we have learned much about these additional microbiota members, they are still generally considered “neglected”, as research efforts are severely lacking behind the study of the bacteriome. The diverse components of the neglected microbiome not only vary in terms of their average estimated physical size but also in terms of their absolute numbers in the gut (). They contribute significantly to general gut metabolism, gut homeostasis, and provide protection from pathogenic infections.Citation28,Citation29 Additionally, many inflammatory diseases result from or induce imbalances in the composition of the microbiome.Citation27,Citation30 In the following sections we will introduce the gut virome, archaeome, mycobiome and parasitome. We will describe their composition and development, but focus specifically on how these neglected microbiome members modulate the pathogenesis of enteric pathogens.
Figure 1. Components of gut microbiota, their approximate size and abundance.
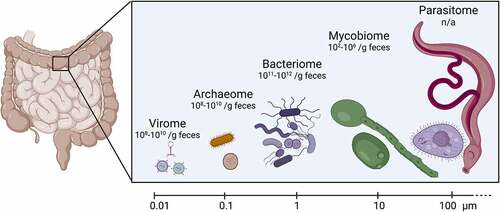
Gut virome
Composition and development
By physical size, the smallest members of the gut microbiome are viruses. However, in overall abundance, their number is close to that of bacteria (). The gut virome is defined as viruses and their genomes that are present in the gastrointestinal tract, which includes bacteriophages, eukaryotic phages, endogenous retroviruses, and archaeal viruses.Citation32,Citation33 Mycoviruses could potentially be part of the gut virome, but this has not been studied to date. Interestingly, plant viruses that are primarily derived from the environment and diet are also found in the gut.Citation34
During the development of the human gut microbiome, prophages are the pioneers of the gut virome. Analysis of the gut microbiome by shotgun metagenomics in neonates showed rapid colonization by bacteria immediately after birth. Within a month, prophages were induced, leading to the development of a gut virome dominated by bacteriophages. Eukaryotic viruses were detected later during development, at around four months of age.Citation35 Once established, the gut virome remains largely unchanged in healthy individuals. A metagenomics study showed a stable gut virome in healthy adults for up to 26 months, with a high prevalence of temperate bacteriophages (phages that integrate into the host chromosome and reside as prophages). The most abundant phages detected were crAssphages, a group of temperate phages present in Bacteroides, Faecalibacterium, Eubacterium, Prevotella, and Parabacteroides. Stable colonizers also included Caudovirales and Microviridae bacteriophages as well as eukaryotic adenoviruses and herpesviruses.Citation36,Citation37 According to the human Gut Virome Database, based on 2,697 human gut metagenomes, bacteriophages dominate 97.7% of the human gut virome, with eukaryotic viruses constituting 2.1%, and archaeal viruses making up 0.1% of the human gut virome.Citation32,Citation38
Role of the gut virome during enteric bacterial infections
Even though gut viruses are a substantial part of the gut microbiome, the functions of most viruses in the gut virome have yet to be fully established.Citation31 Nevertheless, insightful research has been performed on the role of the gut virome during enteric infections. Studies showed that bacteriophages can direct bacterial evolution and diversity by horizontal gene transfer and can influence bacterial populations, their functions in the gut, and the gut metabolome by interacting with their bacterial hosts.Citation39,Citation40 Recent research highlights the importance of phages in maintaining the colonization resistance to enteric pathogens that is mediated by the gut bacteriome. Administration of targeted phage cocktails against E. coli and E. faecalis had a lasting impact on colonization resistance against S. Typhimurium. Phage-mediated reduced abundance of E. coli and E. faecalis resulted in decreased colonization resistance to S. Typhimurium. Unexpectedly, colonization resistance remained low even after E. coli and E. faecalis abundance recovered, hinting at phage-mediated effects that are not limited to reduction of target bacteria abundance.Citation41 Similar to the gut bacteriome, the gut virome can aid in the maintenance of gut homeostasis but can also induce an immune response and modulate the host response to enteric infections ().Citation225
Figure 2. Effect of the gut virome on enteric bacterial infections.
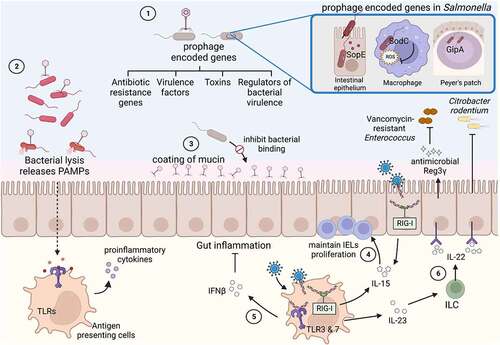
Direct inter-species interactions
The prophages present in bacterial hosts directly influence bacterial virulence by several methods (). Prophages can encode for toxins and other virulence factors, which consequently increases the coding potential of their hosts. Examples of phage-derived toxins are Shiga toxin in Shiga toxin-producing Escherichia coli (STEC),Citation42 Panton-Valentine leukocidin (PVL), a toxin with activity against phagocytes, toxic shock syndrome toxin (TSST) in Staphylococcus aureus,Citation43,Citation44 and accessory cholera exotoxin (ace) in Vibrio cholerae.Citation45,Citation46 Prophages also encode for other virulence factors. Efficient entry of S. Typhimurium into intestinal epithelium for example requires the effector SopE, which is produced by the temperate phage Sopɸ ().Citation47,Citation48 After crossing of the epithelial barrier, Salmonella preferentially localizes to Peyer’s patches, secondary lymphoid tissues predominantly found in the small intestine. Survival in Peyer’s patches requires another prophage, Gifsy-1, which encodes for the gipA gene ().Citation49 The Gifsy-1 prophage also encodes for a superoxide dismutase (SodC), which provides protection against superoxide generated by phagocytes, therefore increasing S. Typhimurium survival inside phagocytic cells ().Citation50 The platelet adherence of Streptococcus mitis is mediated by surface proteins present in a prophage, SM1,Citation51 and resistance to the neutrophil-mediated killing of S. aureus is mediated by a set of genes called chemotaxis inhibitory protein (CHIPS), which are also encoded by a prophage.Citation52 In addition to toxins and effectors, temperate phages can encode resistance to many different antibiotics. Such phages were found in many species, such as S. Typhimurium,Citation53 E. coli,Citation54 or S. aureus.Citation55 While antibiotic resistance is mainly transferred to other bacteria by conjugation or transformation, phage transduction is increasingly being recognized as an additional effective means to transfer antibiotic resistance. For this, prophages have to be induced (see below).
Prophages can not only increase coding potential, they can also regulate bacterial gene expression and modulate the mammalian immune system’s response to their bacterial host. Bacterial pathogens can be rendered more virulent through the induction of prophages. Induction of the prophage CTXɸ, encoding cholera toxin, can result in the transfer of lytic phage to an avirulent strain of V. cholerae, rendering it virulent.Citation56 In Corynebacterium diphtheriae, phage induction can increase the synthesis of diphtheria toxin.Citation57 However, even without induction, prophages can regulate virulence. The phage transcription factor Cro has been shown to activate the type III secretion system (T3SS) in enterohemorrhagic E. coli.Citation58 Similarly, production of the M protein on the surface of Streptococci is regulated by a prophage.Citation59–61
Phage-induced bacterial lysis is also an important immune response modulator and can even protect the human host. Phage-induced bacterial lysis can increase the presence of pathogen-associated molecular patterns (PAMPs) in the mammalian host. These bacterial PAMPS are recognized by antigen-presenting cells and activate an immune response (). Elevated levels of cell-free DNA in the systemic circulation were for example detected within 24 h following bacteriophage oral administration.Citation62 However, whether bacteriophages also increase gut permeability facilitating the translocation of PAMPs to the systemic circulation is currently not known. Phages also coat mucin glycoproteins in the gut by binding via IgA-like protein domains present on phage capsids.Citation63 This strategic position allows phages to lyse bacteria traversing the mucus layer, thereby protecting the underlying epithelial membrane from potentially harmful bacteria ().
Maintaining gut homeostasis and immune modulation
The gut virome plays a crucial role in maintaining the health of the gastrointestinal tract. This is highlighted by a study showing the protective role of the virome against the development of colitis. The pretreatment of mice with an antiviral cocktail led to more severe dextran sulfate sodium (DSS)-induced colitis, when compared with mice that were not pretreated. This protective effect was found to be mediated by Toll-like receptor (TLR) 3 and TLR7, which are sensors for double-stranded and single stranded RNA viruses, and the subsequent induction of Interferon β (IFN-β) secretion ().Citation64 Recently, gut viruses have also been shown to aid in maintaining the number of intestinal intraepithelial lymphocytes (IELs), which are crucial components of an effective mucosal barrier. The cytosolic viral RNA-sensing receptor in antigen-presenting cells, RIG-I, recognizes commensal viruses and maintains IELs in an Interleukin (IL)-15 axis-dependent manner (). Treatment with antiviral cocktails reduced the number of IELs in the intestine.Citation65
The composition of the gut virome is considered to be stable for years, but the expansion of certain species has been observed in the case of intestinal inflammation. Phages of Caudovirales species, including Escherichia, and Enterobacter phages, were more abundant in patients with ulcerative colitis (UC), as compared with healthy controls.Citation66 UC patients also showed an expansion of Enterobacteriaceae,Citation67 which could be the reason for the observed increase in phages that infect this bacterial family. Multiple viral functions, particularly associated with increased host bacteria fitness and pathogenicity, were also increased in UC patients.Citation66 The important role of the gut virome during inflammatory diseases was further highlighted by a study in mice, which showed that the presence of an enteric virus, murine norovirus (MNV), increased the susceptibility of Atg16L1 (an autophagy gene) hypomorphs to develop Crohn’s disease-like pathologies.Citation68
Products from commensal bacteria can induce immune signaling pathways of the host and thereby enhance barrier functions in the intestine.Citation69 Remarkably, the presence of viruses is sufficient to provide similar cues to the host. Infection by MNV reversed the intestinal abnormalities caused by depletion of bacteria. Intestinal morphology and lymphocyte differentiation, which are altered in germ-free mice due to the absence of commensal bacteria, were restored when germ-free mice were infected with MNV.Citation70 The ability of gut viruses to maintain gut homeostasis therefore prompted the question of whether they also prime the gut immune system for protection against bacterial infections. MNV indeed provided an IL-22-dependent protection against lethal infection by Citrobacter rodentium and Vancomycin-resistant Enterococcus (VRE) ().Citation71,Citation72 These beneficial effects could not only be effectively promoted by live virus but also by a resiquimod (R848), a synthetic ligand of TLR-7. Engagement of TLR-7 on dendritic cells resulted in antiviral responses, but also in the production of IL-23. The resulting immune response ultimately culminated in the production of the antimicrobial peptide Reg3γ ().Citation72 These examples of inter-kingdom interactions between viruses, bacteria and host highlight that viruses need to be considered when attempting to detangle the complex web of interactions with enteric bacterial pathogens in the gut.
Gut archaeome
Composition and development
The domain Archaea consists of anaerobic prokaryotes that share traits with bacteria, but also resemble eukaryotes in some ways. Morphologically, archaea look like bacteria; they do not have a nucleus and can be rod or round shaped (). However, cellular processes like transcription and translation are more similar to eukaryotes. Archaea display unique metabolic and biochemical pathways that separate them from the other domains of life, such as the ability to respire H2 into methane.Citation73 We normally think of archaea as extremophiles; resistant organisms that live in almost uninhabitable environments with high temperatures (45–80°C), high salt (>0.8 M), and high acidity (pH < 3) or alkalinity (pH > 9).Citation74 However, archaea are also found to reside within humans and other animals. The first discovery of archaea in the human gut was made in the 1960’s, but identification methods were not specific enough to determine the species.Citation73,Citation75 Direct culturing of archaea from intestinal samples is difficult due to their specific growth requirements. Molecular methods, such as 16S rRNA sequencing and metagenomic analysis, have improved the identification of archaea, but full genome sequence databases that form the basis for such analyses are far behind those available for bacteria. Additionally, archaea-specific primers are not routinely used in microbiota sequencing, which limits the ability to detect archaeal constituents.Citation75,Citation76
The archaeome is thought to appear shortly after birth, with transmission from the mother to the infant. However, infant microbiomes are more variable than adults and may have transient colonization by archaea.Citation77,Citation78 By adulthood, the archaeome is stable, but can be disrupted by diet, gastrointestinal stress, and changes in the host immune system.Citation76,Citation78 Studies have identified certain archaeal species (Methanobrevibacter smithii and Methanosphaera stadtmanae) that are almost universally present throughout human populations, and have even been proposed as identifiers of human fecal contamination of waste-water, as they are highly specific to humans and not found in the environment.Citation79,Citation80 The majority of these human-associated archaea are classified as methanogens. These microbes are able to take byproducts from bacterial fermentation and further metabolize them into methane, exemplifying a cross-feeding behavior between microbiome members.
Maintaining gut homeostasis and immune modulation
The most commonly identified archaeon in the human gut is the methanogen Methanobrevibacter smithii, which is found in 30–95% of individuals studied and comprises up to 10% of all anaerobes.Citation28,Citation80,Citation81 M. smithii is able to combine excess H2 with CO2 to produce methane (CH4), acting as a hydrogen sink (). High levels of hydrogen in the intestines can cause alterations in the redox potential, which inhibits bacterial metabolism rates.Citation82–84 It is hypothesized that M. smithii can activate host immune cells, although the specific microbe-associated molecular patterns (MAMPs) have not been identified. One investigation found that intestinal epithelial cells do not react to Methanobrevibacter species, but that dendritic cells are stimulated and able to phagocytose the commensal archaeal species.Citation81
Figure 3. Effect of the gut archaeome on enteric bacterial infections.
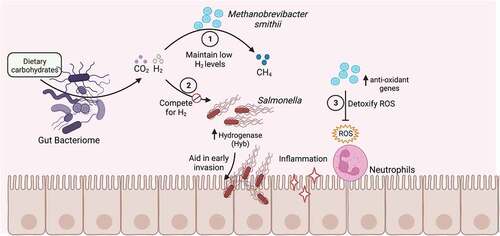
Interactions with enteric bacterial pathogens
In a human clinical study investigating the role of microbiota composition and functionality in vaccine efficacy and infection, higher abundance of Methanobrevibacter was associated with less severe symptoms following Salmonella Typhi challenge.Citation85 The researchers further observed that Methanobrevibacter antioxidant-associated genes were upregulated during S. Typhi infection. They propose this could be due to S. Typhi-induced reactive oxygen species (ROS) production by phagocytes. ROS production is an immune mechanism used to combat pathogens, but it can also lead to intestinal inflammation and an altered redox potential, potentially causing harm to the host and commensal organisms.Citation86 Salmonella Typhimurium has a number of superoxide dismutases that allow for detoxification of superoxides and enable it to survive the oxidative stress.Citation87 S. Typhimurium has been shown to take advantage of an inflammatory environment to outcompete commensal bacteria, reducing colonization resistance and enhancing infection.Citation22,Citation87–89 It is possible that antioxidative effectors produced by resident archaea could combat the ROS response, limiting inflammation and damage, thereby providing protection from S. Typhimurium infection.Citation85,Citation86 It has also been suggested that M. smithii, in addition to hydrogenotrophic commensal bacteria, can compete with S. Typhimurium for hydrogen during metabolic processes (). S. Typhimurium encodes for hydrogenases that allow the bacterium to use hydrogen as a source of energy during early infection.Citation90–92 The S. Typhimurium hyb hydrogenase has been shown to be required for the initial (first 24 h of infection) invasion of the gut epithelium in low complex microbiota (LCM) mice, presumably before high levels of inflammation in the gut are reached.Citation90 Interestingly, addition of an H2-consumer during S. Typhimurium infection reduced the colonization of Salmonella. An avirulent S. Typhimurium strain was used as the competing consumer in these experiments, but it is possible that addition of a methanogenic archaeon would result in the same phenotype.Citation90 These data suggest that initial invasion by S. Typhimurium could be inhibited by the presence of a competitor, such as M. smithiiCitation76,Citation90 ().
Archaea are an important component of the healthy gut microbiota. They participate in syntrophic interactions with fermentative bacteria and help maintain the redox potential with the gastrointestinal tract.Citation84 These organisms also aid in providing colonization resistance and metabolite competition to enteric pathogens.Citation85,Citation90 Further research into the functions of the human gut archaeome can identify their role in gut homeostasis and provide an avenue for treatment options to combat dysbiosis and enteric diseases.
Gut mycobiome
Composition and development
Not only prokaryotes and viruses but also microeukaryotes can be found in the gut environment. The gut mycobiome is the collection of fungi present in the gastrointestinal tract. In humans, fungi are detected as early as 10 days after birth.Citation93 The presence of fungi in the meconium (the first stool) raises the possibility that a gut mycobiota exists even prior to birth.Citation94 However, this notion is contested, given that contamination by environmental fungi during sample acquisition and processing cannot be excluded.Citation94,Citation95 Fungal diversity and the fungal species present in the infant gut mycobiota initially share a lot of similarities with the maternal mycobiota and seem to vary by mode of child birth. Infants that were delivered vaginally showed an enrichment in Candida spp., and infants born through emergency C-section were predominantly colonized with species of the skin commensal fungal genus, Malassezia.Citation96,Citation97 Overall, the most abundant fungal genera identified in infant gut mycobiota studies were Candida, Rhodotorula, Malassezia, Saccharomyces, and Debaryomyces.Citation93,Citation98–101 In particular, Debaryomyces hansenii was one of the most abundant fungal species present in the infant gut during breastfeeding.Citation93 It is only after weaning that fungi acquired through the diet, such as Saccharomyces cerevisiae, become highly abundant.Citation93
The adult gut is predominantly composed of Ascomycota, Basidiomycota, and Zygomycota at the phylum level and Candida, Paecilomyces, Penicillium, Aspergillus, Trichosporon, Rhodotorula, Cladosporium, Aureobasidium, Saccharomycetales, Fusarium, and Cryptococcus at the genus level.Citation102–105 To date, more than 200 species of fungi have been identified in the adult gut. This seems to indicate a diverse fungal microbiome in the human gut. It is important to note though that the composition of the gut mycobiota can vary drastically between individualsCitation106,Citation107 and a majority of species were only detected in a single sample.Citation108,Citation109 The definition of core mycobiome members that are present in most individuals has therefore been challenging. One of the mycobiome members that has been frequently found in fecal samples of healthy adults and that might constitute a core genus is Malassezia.Citation107,Citation110,Citation111 However, whether Malassezia is a true gut resident requires further investigation using more invasive sampling techniques, as the contamination of the feces by skin flora cannot be excluded.Citation108 Geographical location might additionally influence the composition of the mycobiome. Malassezia was present in volunteers recruited from Houston, Texas, but not found in healthy adults from Pennsylvania.Citation107,Citation112
Role of gut mycobiome during enteric bacterial infections
Generally, low fungal abundance is considered to be a characteristic of a healthy gut. Conversely, higher abundance of fungi, and particularly an increase in the yeast Candida albicans, have been associated with disease. An imbalance in the gut mycobiota composition has been associated with enteric diseases, such as irritable bowel syndrome (IBS), inflammatory bowel diseases (IBD), and even diseases affecting other distal organs, such as Alzheimer’s disease, cancer, and alcohol-induced liver diseases.Citation113–119 Many studies have also examined the interaction of bacteria with the gut mycobiota. For example, gut commensal bacteria, such as Bacteroidetes and Firmicutes, provided colonization resistance against C. albicans.Citation120 Herman et al. reported a positive association between the presence of Candida and species of the genus Enterobacter among 68,000 clinical fecal samples.Citation121 Similarly, recurrence of S. Typhi and S. Paratyphi infection increased when patients were colonized with Candida spp.Citation122 C. albicans also reduced the necessary infectious dose and increased the dissemination of Staphylococcus aureus, Serratia marcescens, and Streptococcus faecalis in mice.Citation123 Importantly, C. albicans can increase S. aureus toxin production during in vitro and in vivo experiments, synergistically increasing bacterial virulence.Citation126,Citation127 Although inoculations were performed intraperitoneally in this particular set of experiments, similar interactions could occur in the gut, where fungal and bacterial partners can co-occur during enteric infections. Conversely, Saccharomyces boulardii is used as a probiotic to alleviate the intestinal colonization of Clostridium difficile following broad-spectrum antibiotic therapy.Citation128–130 Thus, the effect of the gut mycobiota during enteric bacterial infections should not be neglected. In this section, we will discuss how the gut mycobiota affects enteric bacterial infections, either due to direct interkingdom interactions or indirectly by maintaining immune homeostasis.
Direct inter-species interactions
Direct interactions between the gut mycobiota and certain enteric bacterial pathogens can lead to either synergistic or antagonistic effects during disease progression.Citation131 Most of these direct interactions were elucidated in in vitro systems or at body sites, such as the oral cavity, vagina, or the lungs, but could similarly occur in the gut.Citation124,Citation125,Citation132–136 The direct binding of the probiotic yeast Saccharomyces boulardii to Gram negative bacterial pathogens E. coli and S. Typhimurium is suggested to decrease bacterial virulence. The yeast is therefore frequently studied as an additive to livestock feed.Citation137 The underlying mechanisms could be either that binding to the yeast decreases the pathogens’ binding to intestinal epithelium or that binding decreases the transit time through the gut and therefore facilitates pathogen eliminationCitation138 (). The binding is inhibited in the presence of mannose, indicating the association of these bacteria to the surface mannose residues present on S. boulardii.Citation138–140 Fungal-bacterial mixed biofilms have been observed at different body sites, including the gut. These biofilms provide protection against antimicrobial drugs and immune cell recognition ().Citation141,Citation142 Using an in vitro biofilm model, C. tropicalis, E. coli, and Serratia marcescens were observed to form more robust biofilms when all partners were present, compared to single or double species biofilms. The lipopolysaccharides produced by S. marcescens and E. coli significantly enhanced fungal biofilm maturation.Citation143 These polymicrobial biofilms are highly relevant to enteric infections, as E. coli, S. marcescens, and C. albicans are abundant in fecal samples from IBD patients.Citation144 Similarly, mixed-species biofilms of C. albicans and Citrobacter freundii were shown to form more rapidly than single-species biofilms, with fungal cells forming the main core of biofilm and bacteria associating with the periphery.Citation145
Figure 4. Direct interactions of gut fungi with enteric bacterial pathogens.
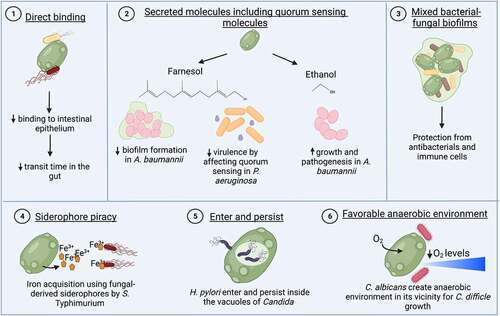
Members of the gut mycobiota can also directly interact with enteric bacterial pathogens via secreted molecules such as quorum sensing molecules. The quorum-sensing molecule farnesol is produced by C. albicans, which colonizes the gut and can cause systemic disease when breaching the intestinal barrier. Farnesol increased resistance to vancomycin in Staphylococcus aureus during systemic co-infections with both pathogens. Higher resistance was in part mediated by an increase in the expression of drug efflux pumps.Citation146 Conversely, farnesol can also have detrimental effects on S. aureus. It can synergize with antimicrobial compounds and impair S. aureus biofilm formation and membrane integrity.Citation147 Farnesol also affected quorum sensing regulation in Pseudomonas aeruginosa, decreased P. aeruginosa virulence,Citation148 and reduced biofilm formation and viability of A. baumanii ().Citation149,Citation150 Conversely, other secreted factors such as ethanol from Saccharomyces cerevisiae stimulated the growth and pathogenicity of several Acinetobacter species including Acinetobacter baumannii in a nematode model with Caenorhabditis elegans ().Citation151 A protease secreted by S. boulardii degraded toxins A and B of C. difficile and provided protection against C. difficile infection.Citation128,Citation152 In addition, S. boulardii can also detoxify cholera toxin by internalizing and therefore neutralizing its A subunit.Citation153 There are other examples of direct interactions between a specific enteric bacterial pathogen and the mycobiota. One of these interactions is “enter and persist”, as shown for Helicobacter pylori, which can enter and grow inside of the vacuole of Candida spp. as a survival strategy ().Citation154 Another interaction is based on the creation of a favorable anaerobic environment. C. albicans enhanced the growth of C. difficile, an anaerobic bacterium, by consuming oxygen and thereby reducing oxygen tension for C. difficile in the vicinity of the yeast ().Citation155,Citation156 On the other hand, C. albicans colonization changes lipid species levels in the cecal lumen, which limits C. difficile colonization.Citation157 In a recently published study, our lab has shown another example of a direct interaction mechanism: siderophore piracy. S. Typhimurium has receptors for fungal siderophores and can acquire iron in the gut using xenosiderophores produced by fungi (). This fungal siderophore mediated acquisition of iron by S. Typhimurium suggests an inter-kingdom cross-feeding between gut mycobiota and enteric bacteria.Citation158
Maintaining gut homeostasis and immune modulation
Recent studies have suggested that the gut mycobiota aids in the maintenance of gut immune homeostasis. Fungal dysbiosis is highly associated with inflammatory diseases, especially in immunocompromised patients. The host immune response can be modulated by the cell wall components (β-glucan, mannans, and chitin) present in commensal fungi, such as S. cerevisiae and C. albicans. These pathogen-associated molecular patterns (PAMPs) are recognized by pathogen recognition receptors (PRRs), such as TLR2 and Dectin-1 (recognizes β-glucans and chitin), and mannose receptor, TLR4, Dectin-2, Mincle, and DC-SIGN (recognize mannans and mannoproteins) present on the surface of dendritic cells and macrophages ().Citation159–164 The recognition of such PAMPs primes immune cells to protect against enteric infections (). This is exemplified by the finding that pre-exposure of human monocytes to S. cerevisiae chitin promotes proinflammatory cytokines production and increases intracellular killing of fungi and bacteria.Citation165
Figure 5. Indirect effects of the gut mycobiome on enteric bacterial infections.
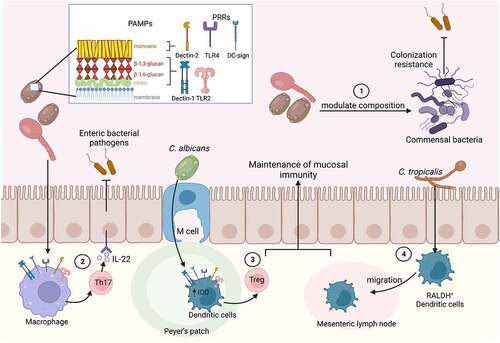
Several studies evidently showed how gut commensal fungi are involved in maintaining mucosal immunity and thus indirectly help to combat enteric infections. Colonization with intestinal fungi, for example, resulted in the accumulation of circulating Th17 CD4+ T cells and granulocytes.Citation166–168 These cells are protective against enteric infections that require the mounting of an effective Th17 response for pathogen clearance (). The maturation of murine lymph nodes involves the migration of dendritic cells expressing retinol dehydrogenase (RALDH+) from the intestinal lamina propria to the peripheral and mesenteric lymph nodes. Germ-free mice did not accumulate these RALDH+ dendritic cells in the lymph nodes, which negatively affected lymph nodes structure and cellularity. The decrease in RALDH+ dendritic cells was also observed in wild type mice treated with an antifungal cocktail, but not in antibiotic treated mice. Interestingly, oral gavage with C. tropicalis regained the migration of gut RALDH+ dendritic cells to the peripheral lymph nodes (). This effect was found to be specific to the gut commensal fungus C. tropicalis, as other commensal fungi, such as Trichosporon asahii or S. cerevisiae did not induce this migration.Citation169,Citation170 During inflammatory conditions, the presence of gut mycobiota members also provided protection to the gastrointestinal tract. Colonization with C. albicans helped to maintain immune homeostasis and to limit inflammation by promoting the expansion of regulatory T cells during DSS induced colitis. Peyer’s patch dendritic cells were shown to recognize the yeast, which induced 2,3-indoleamine dioxygenase, which in turn promoted regulatory T cell generation ().Citation171,Citation172 Pre-colonization with C. albicans also enhanced the protective immune response and survival after C. difficile challenge.Citation173 Mucosa-associated fungi, including C. albicans, were additionally shown to strengthen intestinal epithelial function and protect against enteric bacterial infections with a mechanism dependent on IL-22 production by CD4+ T helper cells.Citation166
The gut mycobiome can also indirectly affect colonization resistance provided by the gut bacteriome (). Long-term treatment with antifungal drugs altered the composition of the bacterial community with a decrease in the relative detection of Bacteroides, Allobaculum, Clostridium, Desulfovibrio, and Lactobacillus spp., and an increase in the relative detection of Anaerostipes, Coprococcus, and Streptococcus.Citation174 Presence of fungal species (C. albicans, C. glabrata, C. parapsilosis, I. orientalis, and R. mucilaginosa) were shown to induce marked changes in the composition of a defined bacterial microbiome in gnotobiotic mice and significantly reduced colonization of Bifidobacterium longum and Lactobacillus reuteri.Citation175 Furthermore, introduction of C. albicans to the gut environment altered how bacterial communities recover after antibiotic treatment. Administration of antibiotics facilitated the colonization of C. albicans, which subsequently hindered the long-term colonization by commensal Lactobacillus strains and instead facilitated the colonization of the pathobiont E. faecalis. Presence of C. albicans also changed the abundance of bacteria involved in colonization resistance to bacterial infection, such as Bacteroidetes, Lactobacillaceae, and Lachnospiraceae.Citation176,Citation177 In addition, fungal dysbiosis has been shown to reduce efficacy of fecal microbiota transplantation for C. difficile infection. A high abundance of C. albicans in donors reduced the efficacy, whereas a high abundance of Saccharomyces and Aspergillus in donors increased the efficacy of fecal microbiota transplant in controlling C. difficile infection.Citation114
The described interactions of mycobiota members with bacterial pathogens highlight that also microbiome members with low abundance can greatly influence gut homeostasis and bacterial pathogenesis.
Gut parasitome
In contrast to the obvious commensal nature of most components of the microbiome, there may be a role for parasites in the healthy gut. The term parasitism is defined as the interaction between organisms in which one (the parasite) reaps benefits to the detriment of the other (the host). Although most pathogens can be described as parasites, this term is used medically to describe protozoa and helminths.Citation178,Citation179 Protozoa are single-celled, simple eukaryotic organisms that are typically less than 50 μm in size (). Examples of protozoan parasites are Plasmodium species, which cause malaria; Entamoeba histolytica, a causative agent of intestinal amebiasis; and Giardia duodenalis, a common enteric parasite that causes the diarrheal disease giardiasis.Citation178,Citation180 These organisms go through complex life stages; cysts are shed through feces into the environment and contaminate water and food. Once ingested, the protozoa encyst and progress into the trophozoite stage, considered to be the infectious form. Many protozoa cause diarrheal diseases by directly invading and damaging the intestinal tissues, but they can also produce toxic molecules that aid in disrupting host defenses.Citation86,Citation178
Helminths are multicellular organisms with worm-like morphologies that can range from a few millimeters to several centimeters in size ().Citation178,Citation180 Common helminths are tapeworms (cestodes), flukes (schistosomes and trematodes), and roundworms (nematodes). These parasites are the main targets of deworming medications given to domestic pets and livestock and can be transmitted to humans. Helminths also cycle through different life stages, similar to protozoa, in which the eggs are shed in feces and then hatch into larvae in intermediate hosts (i.e. snails), which mature into the pathogenic adult form. However, some helminths, such as schistosomes, penetrate the skin and disseminate through the blood to target organs. These large, multicellular worms can form blockages in different organs, leading to tissue damage.Citation178,Citation181 Despite this, many enteric parasitic infections are asymptomatic and can persist for years. Parasitic infections are more common in tropical regions, developing countries, and areas with inadequate water and food sanitation, but can also affect developed countries.
Composition and development
Although the name parasite implies harm to the host, some parasitic organisms may exhibit asymptomatic colonization of the host and engage in a commensal relationship, which has led to the inclusion of the parasitome as a component of the microbiota.Citation182,Citation183 Blastocystis, Dientamoeba, and certain Entamoeba species are parasites that have been found to colonize the human gastrointestinal tract and may provide beneficial impacts on the bacterial richness and diversity.Citation77,Citation182,Citation184,Citation185 In fact, Blastocystis prevalence can range from less than 10% up to 100% in fecal samples from healthy human subjects, depending on the location of the surveyed population.Citation186–190 One retrospective metagenomic analysis found Blastocystis in 18.4% of all Danish and Spanish individuals involved in the study, with a higher prevalence in healthy versus ulcerative colitis patients (20.3% vs 14.9%, respectively).Citation191 In contrast, a study of Senegalese children identified Blastocystis in 100% of study participants, regardless of health status (defined as asymptomatic or symptomatic gastrointestinal disease).Citation192 It is important to note that although the commensal status of Blastocystis and other gut-associated protozoa is controversial, the presence of Blastocystis has been correlated with an increase in bacterial diversity and lower abundance in Enterobacteriaceae.Citation77,Citation182,Citation185 Higher bacterial diversity is commonly seen as an attribute of a healthy gut microbiota.Citation77,Citation193 The studies mentioned above are sequencing-based and do not provide any mechanism for how or why protozoa can impact the diversity of intestinal bacteria. Further research is needed to determine the commensal versus pathogenic nature of Blastocystis within the human gastrointestinal tract.
Parasitic infections have been shown to impact the makeup of the normal bacterial flora within the gut. Mice infected with Trichuris muris, the mouse whipworm, underwent significant but transitory alterations in the diversity and abundance of Bacteroidetes, as well as functional differences in metabolomic activity. However, incomplete restoration of the microbiota was seen after parasitic infection was cleared, indicating that there may be an active crosstalk between the microbiota and parasites.Citation194 Interestingly, another set of studies found that T. muris requires the presence of a bacterial microbiota in order to establish an infection. T. muris larvae were unable to develop and colonize germ-free mice but this ability was restored when mice were colonized with a single bacterial species.Citation195 These experiments illustrate the evolutionary relationship between different members of the gut microbiota.
Immune modulation
Parasitic organisms have evolved alongside humans for most of our existence and have developed strategies to evade or modulate the host immune system. Recognition begins with parasitic invasion of the mucosa and cytokine responses of the intestinal epithelial cells. Some protozoans trigger a Th1 response, while helminths tend to elicit a Th2 response.Citation181 However, immunosuppression is a common method parasites use to enhance their survival in a human host.Citation179,Citation181,Citation196 Protozoans have been shown to secrete virulence factors that can directly downregulate pro-inflammatory cytokines, inducing an anti-inflammatory environment.Citation181 Colonization with Trichuris muris has been shown to be protective against intestinal damage and to promote normal goblet cell development in mice lacking Nod2. Nod2−/− mice colonized with Bacteroides vulgatus, a common member of the gut microbiota, develop spontaneous intestinal abnormalities, such as lack of functional goblet cells, that mimic human Crohn’s disease. T. muris infection limited B. vulgatus colonization via the induction of a strong Th2 immune response.Citation197 The lack of parasitic exposure has been credited with increasing allergy and autoinflammatory conditions, commonly referred to as the Hygiene Hypothesis.Citation198 This theory posits that greater sanitation and cleanliness reduces exposure to common microbes that are important to immune system development and priming of responses. Allergies induce robust IgE antibody production against specific agents, and extreme allergic responses are due to hyperactive immune activity. Parasitic colonization can induce similar IgE expansion, although the antibodies are nonspecific.Citation199 Nonspecific IgE antibodies can suppress the production of more specific antibodies, thereby suppressing an immune response to an allergen.Citation199 Along these lines, the use of helminths or parasite-associated metabolites have begun to be investigated as anti-inflammatory therapeutics, although this idea is still controversial.Citation200–202 Regardless, commensal parasites may be important for overall immune system functioning and their role in homeostasis and infection should not be overlooked.
Interactions with enteric bacterial pathogens
Very few studies have directly investigated the role the parasitome plays in microbiota homeostasis, and even fewer have focused on the contribution intestinal parasites have on enhancing or inhibiting enteric bacterial infections. However, this neglected component of the microbiome has been shown to have clinical relevance that necessitates further investigation.
Co-infections of parasites and enteric bacterial pathogens have been reported in areas where both pathogens are endemic.Citation191,Citation203,Citation204 Entamoeba species have been found along with enteropathogenic E. coli (EPEC) or Shigella dysenteriae in children with diarrheal disease.Citation205–207 In vitro studies have found that co-infection of intestinal epithelial cells by the amoeba E. histolytica and enteropathogenic bacteria led to increased immune activation, epithelial cell damage, and virulence, compared to co-infection with the avirulent species E. dispar.Citation208 This is possibly due to enhanced E. histolytica virulence upon phagocytosis of bacteria. E. histolytica displayed increased adhesion to host cells and increased production of cysteine proteinases after phagocytosis of EPEC or Shigella, which the researchers hypothesized was responsible for increased host cell damage.Citation208
Similarly, helminth and trematode co-infections with Non-Typhoidal Salmonella are common in certain populations.Citation209,Citation210 Helminth worms, such as Heligmosomoides polygyrus, have been shown to facilitate high levels of S. Typhimurium colonization of the small intestine ().Citation209,Citation211 This could be due to alterations in metabolites that confer colonization resistance to bacterial pathogens and/or due to modulation of the immune response that prevents bacterial clearance, but the researchers note that this effect is not due to increased host cell invasion by S. Typhimurium.Citation190,Citation209 Interestingly, deworming of mice prior to S. Typhimurium infection abrogated this enhanced colonization phenotype, indicating that the presence of helminth worms is necessary to promote S. Typhimurium growth in vivo.Citation211 Prophylactic deworming in endemic regions may be a way to reduce the severity of enteric diseases.
Figure 6. Effects of the gut parasitome on enteric bacterial infections.
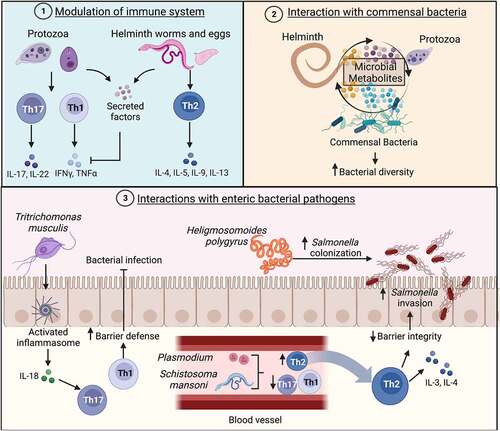
Commensal protists are likely present in many other species and are not exclusive to humans. One study has identified a rodent parasite that exists as a commensal member of the microbiota and provides protection against invasive intestinal bacterial infections. Tritrichomonas musculis, a close ortholog to Dientamoeba fragilis, can stably colonize the mouse gastrointestinal tract.Citation212 Mice colonized with T. musculis showed an expansion of Th1 and Th17 cells in the mucosa of the colon. Upon S. Typhimurium challenge, T. musculis-infected mice did not develop cecal inflammation and had significantly less dissemination of S. Typhimurium to peripheral organs, as compared to mice without protists.Citation212 This protection was dependent on inflammasome activation and IL-18 release from epithelial cells and demonstrates how parasites can modulate the immune system in a way that prevents bacterial invasion and infectionCitation212 ().
There are several examples of non-gut parasitic infections that enhance S. Typhimurium infection via the modulation of the host immune response. Blood-associated parasites, like Schistosomes and Plasmodium species (causative agents of schistosomiasis and malaria, respectively), have been shown to enhance S. Typhimurium pathogenicity by impairing the host immune response.Citation210,Citation213,Citation214 Blood flukes, like Schistosoma mansoni, have complex life cycles in which different stages of development are associated with infection of various parts of the body. These organisms penetrate the skin and circulate in the blood to the lungs and heart, eventually settling in the liver and undergoing maturation into the adult worm form.Citation215 Adult worms lay eggs that progress to the lumen of the intestines, bladder, and ureter, and these eggs are shed in feces or urine back into the environment. Schistosome eggs have strong immunomodulatory effects, inducing a robust Th2 response with high IL-4 and IL-3 levels and suppressing pro-inflammatory cytokines.Citation215 Co-infection of mice with Schistosoma mansoni and Salmonella Typhimurium resulted in significantly higher Salmonella colonization of the ileum, cecum, and colon, compared to single infection controls.Citation213 Interestingly, higher levels of inflammatory cells were present in the gut lumen of co-infected mice, but there was less inflammation of the mucosa. The presence of schistosome eggs induced a robust Th2 immune response and dampened the Th1/Th17 response that is critical for clearance of S. Typhimurium.Citation213 Similarly, Plasmodium species induce IL-10 production that, during coinfection with S. Typhimurium in mice, leads to diminished phagocyte recruitment to the liver.Citation214 Liver resident macrophages were skewed toward the anti-inflammatory M2 state in response to the malaria pathogen, which leads to enhanced dissemination of S. Typhimurium.Citation214 Plasmodium-induced TNFα-signaling also resulted in reduced stomach acidity, which directly aided S. Typhimurium gastrointestinal colonization.Citation216
Although the notion of commensal parasites as members of the normal human gut microbiota may be controversial, their presence and influence should not be neglected in studies of the microbiome. Protozoa may interact with commensal bacteria to maintain gut homeostasis. Helminths may induce an anti-inflammatory environment, potentially aiding enteric bacteria during infection. These underappreciated members of the gut microbiome play important roles that should not be excluded from future research.
Summary and outlook
Microbiome research output in general and especially research focused on the gut microbiome has seen exponential growth over the last decades. While most research focuses on bacteria, the most abundant members of the gut microbiome, significant advancements have been made also on neglected members, such as viruses, archaea, fungi, and parasites. In this review, we summarized current findings on their role during infections with enteric pathogens, such as members of the Enterobacteriaceae. Despite significant research advancements, many challenges remain. Most research focuses only on one microbiome component while ignoring the others. Consequently, research on the microbiome’s influence on enteric infections is mostly restricted to studying one of its components. However, viruses, archaea, fungi, and to some extent also parasites integrate to influence mucosal immunity and colonization resistance toward enteric bacterial pathogens. Thus, to fully understand the gut microbiome’s role during enteric infections, more inclusive future studies are needed that address more than one microbiome component at a time. One important step into this direction is the now more frequent assessment of fungal communities with Internally Transcribed Spacer (ITS) sequencing alongside routine 16S-sequencing. The recent technological advancements employing whole genome shotgun sequencing for gut microbiome research instead of bacteria-centric 16S sequencing also has tremendous potential for assessing neglected microbiome members. While still more expensive and requiring more extensive bioinformatic analysis, this less biased sequencing approach will help to broaden scientist’s view of the role of different gut microbiome communities during health and disease conditions. In addition, crucial tools to determine functional roles include a) the strategic employment of defined microbial communities that include these neglected members, as well as b) application of metabolomics to assess their function in the context of gut metabolic networks.
Over the past decades, colonization of germ-free mice with defined bacterial communities has enabled the discovery of important interactions that would have been missed in more complex systems. The first widely used bacterial community, the Altered Schaedler Flora (ASF), was highly reductionist in scope and contained only eight anaerobic bacterial species.Citation217,Citation218 Nevertheless, this model has been incredibly useful to study specific host-commensal-pathogen interactions and the influence of the gut bacteriome on various other body systems and functions.Citation217,Citation219 Efforts have been undertaken since to render defined microbiomes more reminiscent of an actual gut bacterial community that is connected in an efficient metabolic network. To that extent, communities that also include facultative anaerobes and other species have been developed, such as the GM15 and the Oligo-Mouse-Microbiota 12 (OMM12) communitiesCitation220 An important improvement of the OMM12 over ASF is for example that it confers colonization resistance to Salmonella Typhimurium.Citation221 Even larger collections have also been constructed, such as the 100 strain-containing Mouse Intestinal Bacterial Collection (miBC).Citation222 However, none of these communities include non-bacterial microbiota components, such as methane-producing archaea. The only notable exception are prophages that are likely present in their bacterial genomes. Future research would greatly benefit from the establishment of defined microbial communities that include components such as archaea, fungi, and commensal protists.
Non-bacterial members of the microbiota also contribute to the overall gut metabolome. However, the extent of their contribution is currently unknown. Some metabolic functions appear straightforward to deduce, e.g. the production of methane by methanogens. Other functions are less obvious and require additional study. Metabolites produced by non-bacterial microbiome members might influence enteric bacterial infection a) directly by competitive exclusion and b) indirectly by modulating the host immune system. One study showed that the addition of yeast species to a defined bacterial community in mice only minimally affected the overall gut metabolome.Citation175 Conversely, the presence of Saccharomyces cerevisiae in humans enhanced host purine metabolism, resulting in higher levels of uric acid, which negatively influenced the pathologies associated with IBD.Citation223 Protozoa and enteric helminths also contribute to the gut metabolome. Introduction of the protist Blastocystis via fecal material transfer (FMT) increased the production of short-chain fatty acids (SCFAs) that ameliorated colitis, whereas presence of enteric helminths changed the gut metabolic profile in a way that promoted Salmonella Typhimurium intracellular invasion.Citation209,Citation224 Future studies will determine to which extent non-bacterial members of the microbiota contribute directly or indirectly to the gut metabolome.
Other key factors, such as age and diet, also influence microbiome composition and richness of the neglected microbiomes. Thus, it will be essential to consider these factors while analyzing the contribution of neglected microbiome members on enteric infections. For example, age can affect the gut virome. Overall viral richness is highest in infants and adults and decreases in elderly individuals. Bacteriophages mirror this trend, but richness in eukaryotic viruses is highest during infancy.Citation38 Similarly, diet is an important factor influencing the composition of gut microbes. Dairy consumption is positively associated with Saccharomyces abundance, and carbohydrate intake is positively associated with Candida and Methanobrevibacter abundance.Citation106,Citation112 These changes also signify the importance of individual variations in the microbiome composition. However, studies have yet to be done to determine if age and diet also affect the gut parasitome.
Neglected microbiome members have been slowly moving into the focus of researchers. This largely under-explored research field will likely greatly expand in the future and reveal more fascinating roles of viruses, archaea, fungi, and parasites for gut biology in general and for the pathogenesis of enteric bacteria in particular.
Acknowledgments
We want to thank all lab members for critical reading of the manuscript. Figures were created at Biorender.com.
Disclosure statement
No potential conflict of interest was reported by the authors.
Additional information
Funding
References
- Clarke G, Grenham S, Scully P, Fitzgerald P, Moloney RD, Shanahan F, Dinan TG, Cryan JF. The microbiome-gut-brain axis during early life regulates the hippocampal serotonergic system in a sex-dependent manner. Mol Psychiatry. 2013;18(6):666–27. doi:10.1038/mp.2012.77.
- Sharon G, Sampson TR, Geschwind DH, Mazmanian SK. The central nervous system and the gut microbiome. Cell. 2016;167(4):915–932. doi:10.1016/j.cell.2016.10.027.
- Donohoe DR, Garge N, Zhang X, Sun W, O’Connell TM, Bunger MK, Bultman SJ. The microbiome and butyrate regulate energy metabolism and autophagy in the mammalian colon. Cell Metab. 2011;13(5):517–526. doi:10.1016/j.cmet.2011.02.018.
- Vijay A, Valdes AM. Role of the gut microbiome in chronic diseases: a narrative review. Eur J Clin Nutr. 2022;76(4):489–501. doi:10.1038/s41430-021-00991-6.
- Fan Y, Pedersen O. Gut microbiota in human metabolic health and disease. Nat Rev Microbiol. 2021;19(1):55–71. doi:10.1038/s41579-020-0433-9.
- Petri WA Jr, Miller M, Binder HJ, Levine MM, Dillingham R, Guerrant RL. Enteric infections, diarrhea, and their impact on function and development. J Clin Invest. 2008;118(4):1277–1290. doi:10.1172/JCI34005.
- Vincent C, Manges AR. Antimicrobial use, human gut microbiota and clostridium difficile colonization and infection. Antibiot (Basel). 2015;4(3):230–253. doi:10.3390/antibiotics4030230.
- Dubin K, Pamer EG, Britton RA, Cani PD. Enterococci and their interactions with the intestinal microbiome. Microbiol Spectr. 2014;5(6). doi:10.1128/microbiolspec.BAD-0014-2016.
- Ryan MP, O’Dwyer J, Adley CC. Evaluation of the complex nomenclature of the clinically and veterinary significant pathogen Salmonella. Biomed Res Int. 2017;2017:3782182. doi:10.1155/2017/3782182.
- Kurtz JR, Goggins JA, McLachlan JB. Salmonella infection: interplay between the bacteria and host immune system. Immunol Lett. 2017;190:42–50. doi:10.1016/j.imlet.2017.07.006.
- Hume PJ, Singh V, Davidson AC, Koronakis V. Swiss army pathogen: the Salmonella entry toolkit. Front Cell Infect Microbiol. 2017;7:348. doi:10.3389/fcimb.2017.00348.
- Bassis CM, Theriot CM, Young VB. Alteration of the murine gastrointestinal microbiota by tigecycline leads to increased susceptibility to Clostridium difficile infection. Antimicrob Agents Chemother. 2014;58(5):2767–2774. doi:10.1128/AAC.02262-13.
- Yang J, Chen W, Xia P, Zhang W. Dynamic comparison of gut microbiota of mice infected with Shigella flexneri via two different infective routes. Exp Ther Med. 2020;19:2273–2281. doi:10.3892/etm.2020.8469.
- Bohnhoff M, Drake BL, Miller CP. Effect of streptomycin on susceptibility of intestinal tract to experimental Salmonella infection. Proc Soc Exp Biol Med. 1954;86(1):132–137. doi:10.3181/00379727-86-21030.
- Byndloss MX, Olsan EE, Rivera-Chávez F, Tiffany CR, Cevallos SA, Lokken KL, Torres TP, Byndloss AJ, Faber F, Gao Y, et al. Microbiota-activated PPAR-γ signaling inhibits dysbiotic Enterobacteriaceae expansion. Science. 2017;357(6351):570–575. doi:10.1126/science.aam9949.
- Momose Y, Hirayama K, Itoh K. Competition for proline between indigenous Escherichia coli and E. coli O157: H7 in gnotobiotic mice associated with infant intestinal microbiota and its contribution to the colonization resistance against E. coli O157: H7. Antonie Van Leeuwenhoek. 2008;94:165–171. doi:10.1007/s10482-008-9222-6.
- Ivanov II, de Llanos Frutos R, Manel N, Yoshinaga K, Rifkin DB, Sartor RB, Finlay BB, Littman DR. Specific microbiota direct the differentiation of IL-17-producing T-helper cells in the mucosa of the small intestine. Cell Host & Microbe. 2008;4:337–349. doi:10.1016/j.chom.2008.09.009.
- Isaac S, Flor-Duro A, Carruana G, Puchades-Carrasco L, Quirant A, Lopez-Nogueroles M, Pineda-Lucena A, Garcia-Garcera M, Ubeda C. Microbiome-mediated fructose depletion restricts murine gut colonization by vancomycin-resistant Enterococcus. Nat Commun. 2022;13(1):7718. doi:10.1038/s41467-022-35380-5.
- Kamada N, Kim Y-G, Sham HP, Vallance BA, Puente JL, Martens EC, Núñez G. Regulated virulence controls the ability of a pathogen to compete with the gut microbiota. Science. 2012;336(6086):1325–1329. doi:10.1126/science.1222195.
- Bäumler AJ, Sperandio V. Interactions between the microbiota and pathogenic bacteria in the gut. Nature. 2016;535(7610):85–93. doi:10.1038/nature18849.
- Sassone-Corsi M, Raffatellu M. No vacancy: how beneficial microbes cooperate with immunity to provide colonization resistance to pathogens. J Immunol. 2015;194(9):4081–4087. doi:10.4049/jimmunol.1403169.
- Rogers AWL, Tsolis RM, Bäumler AJ. Salmonella versus the Microbiome. Microbiol Mol Biol Rev. 2021;85(1). doi:10.1128/MMBR.00027-19.
- Rolhion N, Chassaing B. When pathogenic bacteria meet the intestinal microbiota. Philos Trans R Soc Lond B Biol Sci. 2016;371(1707):20150504. doi:10.1098/rstb.2015.0504.
- Ducarmon QR, Zwittink RD, Hornung BVH, van Schaik W, Young VB, Kuijper EJ. Gut microbiota and colonization resistance against bacterial enteric infection. Microbiol Mol Biol Rev. 2019;83(3). doi:10.1128/mmbr.00007-19.
- Human Microbiome Project Consortium. Structure, function and diversity of the healthy human microbiome. Nature. 2012;486(7402):207–214. doi:10.1038/nature11234.
- Sun Y, O’Riordan MXD. Regulation of bacterial pathogenesis by intestinal short-chain fatty acids. Adv Appl Microbiol. 2013;85:93–118.
- Pickard JM, Zeng MY, Caruso R, Núñez G. Gut microbiota: role in pathogen colonization, immune responses, and inflammatory disease. Immunol Rev. 2017;279(1):70–89. doi:10.1111/imr.12567.
- Matijašić M, Meštrović T, Čipčić Paljetak H, Perić M, Barešić A, Verbanac D. Gut microbiota beyond bacteria—mycobiome, virome, archaeome, and eukaryotic parasites in IBD. Int J Mol Sci. 2020;21(8):2668. doi:10.3390/ijms21082668.
- Vemuri R, Shankar EM, Chieppa M, Eri R, Kavanagh K. Beyond just bacteria: functional biomes in the gut ecosystem including virome, mycobiome, archaeome and helminths. Microorganisms. 2020;8(4):483. doi:10.3390/microorganisms8040483.
- Belizário JE, Faintuch J. Microbiome and gut dysbiosis. Experientia Suppl. 2018;109:459–476.
- Shkoporov AN, Hill C. Bacteriophages of the human gut: the “known unknown” of the microbiome. Cell Host Microbe. 2019;25(2):195–209. doi:10.1016/j.chom.2019.01.017.
- Spencer L, Olawuni B, Singh P. Gut virome: role and distribution in health and gastrointestinal diseases. Front Cell Infect Microbiol. 2022;12:836706. doi:10.3389/fcimb.2022.836706.
- Mukhopadhya I, Segal JP, Carding SR, Hart AL, Hold GL. The gut virome: the “missing link” between gut bacteria and host immunity? Therap Adv Gastroenterol. 2019;12:1756284819836620. doi:10.1177/1756284819836620.
- Zhang T, Breitbart M, Lee WH, Run J-Q, Wei CL, Soh SWL, Hibberd ML, Liu ET, Rohwer F, Ruan Y. RNA viral community in human feces: prevalence of plant pathogenic viruses. PLoS Biol. 2006;4(1):e3. doi:10.1371/journal.pbio.0040003.
- Liang G, Zhao C, Zhang H, Mattei L, Sherrill-Mix S, Bittinger K, Kessler LR, Wu GD, Baldassano RN, DeRusso P, et al. The stepwise assembly of the neonatal virome is modulated by breastfeeding. Nature. 2020;581(7809):470–474. doi:10.1038/s41586-020-2192-1.
- Shkoporov AN, Clooney AG, Sutton TDS, Ryan FJ, Daly KM, Nolan JA, McDonnell SA, Khokhlova EV, Draper LA, Forde A, et al. The human gut virome is highly diverse, stable, and individual specific. Cell Host Microbe. 2019;26(4):527–541.e5. doi:10.1016/j.chom.2019.09.009.
- Dutilh BE, Cassman N, McNair K, Sanchez SE, Silva GGZ, Boling L, Barr JJ, Speth DR, Seguritan V, Aziz RK, et al. A highly abundant bacteriophage discovered in the unknown sequences of human faecal metagenomes. Nat Commun. 2014;5(1):4498. doi:10.1038/ncomms5498.
- Gregory AC, Zablocki O, Zayed AA, Howell A, Bolduc B, Sullivan MB. The gut virome database reveals age-dependent patterns of virome diversity in the human gut. Cell Host & Microbe. 2020;28(5):724–740.e8. doi:10.1016/j.chom.2020.08.003.
- Hsu BB, Gibson TE, Yeliseyev V, Liu Q, Lyon L, Bry L, Silver PA, Gerber GK. Dynamic modulation of the gut microbiota and metabolome by bacteriophages in a mouse model. Cell Host Microbe. 2019;25(6):803–814.e5. doi:10.1016/j.chom.2019.05.001.
- Borodovich T, Shkoporov AN, Paul Ross R, Hill C. Phage-mediated horizontal gene transfer and its implications for the human gut microbiome. Gastroenterol Rep. 2022;10. doi:10.1093/gastro/goac012.
- von Strempel A, Weiss AS, Wittmann J, Silva MS, Ring D, Wortmann E, Clavel T, Debarbieux L, Kleigrewe K, Stecher B. Bacteriophages targeting protective commensals impair resistance against Salmonella Typhimurium infection in gnotobiotic mice. bioRxiv. 2022. https://www.biorxiv.org/content/10.1101/2022.09.28.509654v1.full.
- Gyles CL. Shiga toxin-producing Escherichia coli: an overview. J Anim Sci. 2007;85(suppl_13):E45–E62. doi:10.2527/jas.2006-508.
- Ruzin A, Lindsay J, Novick RP. Molecular genetics of SaPI1–a mobile pathogenicity island in Staphylococcus aureus. Mol Microbiol. 2001;41(2):365–377. doi:10.1046/j.1365-2958.2001.02488.x.
- Shallcross LJ, Fragaszy E, Johnson AM, Hayward AC. The role of the Panton-Valentine leucocidin toxin in staphylococcal disease: a systematic review and meta-analysis. Lancet Infect Dis. 2013;13(1):43–54. doi:10.1016/S1473-3099(12)70238-4.
- Trucksis M, Galen JE, Michalski J, Fasano A, Kaper JB. Accessory cholera enterotoxin (Ace), the third toxin of a Vibrio cholerae virulence cassette. Proc Natl Acad Sci USA. 1993;90(11):5267–5271. doi:10.1073/pnas.90.11.5267.
- Pant A, Das B, Bhadra RK. CTX phage of Vibrio cholerae: genomics and applications. Vaccine. 2020;38(Suppl 1):A7–A12. doi:10.1016/j.vaccine.2019.06.034.
- Wood MW, Rosqvist R, Mullan PB, Edwards MH, Galyov EE. SopE, a secreted protein of Salmonella dublin, is translocated into the target eukaryotic cell via a sip-dependent mechanism and promotes bacterial entry. Mol Microbiol. 1996;22(2):327–338. doi:10.1046/j.1365-2958.1996.00116.x.
- Hardt WD, Urlaub H, Galán JE. A substrate of the centisome 63 type III protein secretion system of Salmonella typhimurium is encoded by a cryptic bacteriophage. Proc Natl Acad Sci USA. 1998;95(5):2574–2579. doi:10.1073/pnas.95.5.2574.
- Stanley TL, Ellermeier CD, Slauch JM. Tissue-specific gene expression identifies a gene in the lysogenic phage Gifsy-1 that affects Salmonella enterica serovar typhimurium survival in Peyer’s patches. J Bacteriol. 2000;182(16):4406–4413. doi:10.1128/JB.182.16.4406-4413.2000.
- Figueroa-Bossi N, Bossi L. Inducible prophages contribute to Salmonella virulence in mice. Mol Microbiol. 1999;33(1):167–176. doi:10.1046/j.1365-2958.1999.01461.x.
- Bensing BA, Siboo IR, Sullam PM, Tuomanen EI. Proteins PblA and PblB of Streptococcus mitis, which promote binding to human platelets, are encoded within a lysogenic bacteriophage. Infect Immun. 2001;69(10):6186–6192. doi:10.1128/IAI.69.10.6186-6192.2001.
- van Wamel WJB, Rooijakkers SHM, Ruyken M, van Kessel KPM, van Strijp JAG. The innate immune modulators staphylococcal complement inhibitor and chemotaxis inhibitory protein of staphylococcus aureus are located on β-hemolysin-converting bacteriophages. J Bacteriol. 2006;188(4):1310–1315. doi:10.1128/JB.188.4.1310-1315.2006.
- Colavecchio A, Cadieux B, Lo A, Goodridge LD. Bacteriophages contribute to the spread of antibiotic resistance genes among foodborne pathogens of the enterobacteriaceae family - a review. Front Microbiol. 2017;8:1108. doi:10.3389/fmicb.2017.01108.
- Pfeifer E, Bonnin RA, Rocha EPC, Goldman GH. Phage-plasmids spread antibiotic resistance genes through infection and lysogenic conversion. MBio. 2022;13(5):e0185122. doi:10.1128/mbio.01851-22.
- Lee Y-D, Park J-H. Phage conversion for β-Lactam antibiotic resistance of Staphylococcus aureus from foods. J Microbiol Biotechnol. 2016;26(2):263–269. doi:10.4014/jmb.1508.08042.
- Faruque SM, Asadulghani, Alim AR, Albert MJ, Islam KM, Mekalanos JJ. Induction of the lysogenic phage encoding cholera toxin in naturally occurring strains of toxigenic Vibrio cholerae O1 and O139. Infect Immun. 1998;66(8):3752–3757. doi:10.1128/IAI.66.8.3752-3757.1998.
- Barksdale L, Garmise L, Horibata K. Virulence, toxinogeny, and lysogeny in Corynebacterium diphtheriae. Ann N Y Acad Sci. 1960;88(5):1093–1108. doi:10.1111/j.1749-6632.1960.tb20099.x.
- Hernandez-Doria JD, Sperandio V. Bacteriophage transcription factor cro regulates virulence gene expression in enterohemorrhagic Escherichia coli. Cell Host & Microbe. 2018;23(5):607–617.e6. doi:10.1016/j.chom.2018.04.007.
- Spanier JG, Cleary PP. Bacteriophage control of antiphagocytic determinants in group a streptococci. J Exp Med. 1980;152(5):1393–1406. doi:10.1084/jem.152.5.1393.
- Metzgar D, Zampolli A. The M protein of group a Streptococcus is a key virulence factor and a clinically relevant strain identification marker. Virulence. 2011;2(5):402–412. doi:10.4161/viru.2.5.16342.
- Kittang BR, Skrede S, Langeland N, Haanshuus CG, Mylvaganam H. Emm gene diversity, superantigen gene profiles and presence of SlaA among clinical isolates of group A, C and G streptococci from western Norway. Eur J Clin Microbiol Infect Dis. 2011;30(3):423–433. doi:10.1007/s10096-010-1105-x.
- Tetz G, Tetz V. Bacteriophages as new human viral pathogens. Microorganisms. 2018;6(2):54. doi:10.3390/microorganisms6020054.
- Barr JJ, Auro R, Furlan M, Whiteson KL, Erb ML, Pogliano J, Stotland A, Wolkowicz R, Cutting AS, Doran KS, et al. Bacteriophage adhering to mucus provide a non–host-derived immunity. Proc Natl Acad Sci USA. 2013;110(26):10771–10776. doi:10.1073/pnas.1305923110.
- Yang JY, Kim MS, Kim E, Cheon JH, Lee YS, Kim Y, Lee SH, Seo SU, Shin SH, Choi SS, et al. Enteric viruses ameliorate gut inflammation via Toll-like Receptor 3 and Toll-like Receptor 7-Mediated Interferon-β production. Immunity. 2016;44(4):889–900. doi:10.1016/j.immuni.2016.03.009.
- Liu L, Gong T, Tao W, Lin B, Li C, Zheng X, Zhu S, Jiang W, Zhou R. Commensal viruses maintain intestinal intraepithelial lymphocytes via noncanonical RIG-I signaling. Nat Immunol. 2019;20(12):1681–1691. doi:10.1038/s41590-019-0513-z.
- Zuo T, Lu XJ, Zhang Y, Cheung CP, Lam S, Zhang F, Tang W, Ching JYL, Zhao R, Chan PKS, et al. Gut mucosal virome alterations in ulcerative colitis. Gut. 2019;68(7):1169–1179. doi:10.1136/gutjnl-2018-318131.
- Khorsand B, Asadzadeh Aghdaei H, Nazemalhosseini-Mojarad E, Nadalian B, Nadalian B, Houri H. Overrepresentation of Enterobacteriaceae and Escherichia coli is the major gut microbiome signature in Crohn’s disease and ulcerative colitis; a comprehensive metagenomic analysis of IBDMDB datasets. Front Cell Infect Microbiol. 2022;12:1015890. doi:10.3389/fcimb.2022.1015890.
- Cadwell K, Patel KK, Maloney NS, Liu T-C, Ng ACY, Storer CE, Head RD, Xavier R, Stappenbeck TS, Virgin HW. Virus-plus-susceptibility gene interaction determines Crohn’s disease gene Atg16L1 phenotypes in intestine. Cell. 2010;141:1135–1145. doi:10.1016/j.cell.2010.05.009.
- Gasaly N, de Vos P, Hermoso MA. Impact of bacterial metabolites on gut barrier function and host immunity: a focus on bacterial metabolism and its relevance for intestinal inflammation. Front Immunol. 2021;12:658354. doi:10.3389/fimmu.2021.658354.
- Kernbauer E, Ding Y, Cadwell K. An enteric virus can replace the beneficial function of commensal bacteria. Nature. 2014;516(7529):94–98. doi:10.1038/nature13960.
- Neil JA, Matsuzawa-Ishimoto Y, Kernbauer-Hölzl E, Schuster SL, Sota S, Venzon M, Dallari S, Galvao Neto A, Hine A, Hudesman D, et al. IFN-I and IL-22 mediate protective effects of intestinal viral infection. Nat microbiol. 2019;4(10):1737–1749. doi:10.1038/s41564-019-0470-1.
- Abt MC, Buffie CG, Sušac B, Becattini S, Carter RA, Leiner I, Keith JW, Artis D, Osborne LC, Pamer EG. TLR-7 activation enhances IL-22–mediated colonization resistance against vancomycin-resistant enterococcus. Sci Transl Med. 2016;8(327):327ra25. doi:10.1126/scitranslmed.aad6663.
- Mihajlovski A, Alric M, Brugère JF. A putative new order of methanogenic Archaea inhabiting the human gut, as revealed by molecular analyses of the mcrA gene. Res Microbiol. 2008;159(7–8):516–521. doi:10.1016/j.resmic.2008.06.007.
- Valentine DL. Adaptations to energy stress dictate the ecology and evolution of the Archaea. Nat Rev Microbiol. 2007;5(4):316–323. doi:10.1038/nrmicro1619.
- Pausan MR, Csorba C, Singer G, Till H, Schöpf V, Santigli E, Klug B, Högenauer C, Blohs M, Moissl-Eichinger C. Exploring the archaeome: detection of archaeal signatures in the human body. Front Microbiol. 2019. doi:10.3389/fmicb.2019.02796.
- Nkamga VD, Henrissat B, Drancourt M. Archaea: essential inhabitants of the human digestive microbiota. Human Microbiome J. 2017;3:1–8. doi:10.1016/j.humic.2016.11.005.
- Audebert C, Even G, Cian A, Loywick A, Merlin S, Viscogliosi E, Chabé M. Colonization with the enteric protozoa Blastocystis is associated with increased diversity of human gut bacterial microbiota. Sci Rep. 2016;6(1):25255. doi:10.1038/srep25255.
- Mihajlovski A, Doré J, Levenez F, Alric M, Brugère JF. Molecular evaluation of the human gut methanogenic archaeal microbiota reveals an age-associated increase of the diversity. Environ Microbiol Rep. 2010;2(2):272–280. doi:10.1111/j.1758-2229.2009.00116.x.
- Johnston C, Ufnar JA, Griffith JF, Gooch JA, Stewart JR. A real-time qPCR assay for the detection of the nifH gene of Methanobrevibacter smithii, a potential indicator of sewage pollution. J Appl Microbiol. 2010;109(6):1946–1956. doi:10.1111/j.1365-2672.2010.04824.x.
- Dridi B, Henry M, Khéchine AE, Raoult D, Drancourt M, Dobrindt U. High prevalence of methanobrevibacter smithii and methanosphaera stadtmanae detected in the human gut using an improved DNA detection protocol. PLoS One. 2009;4(9):e7063. doi:10.1371/journal.pone.0007063.
- Bang C, Weidenbach K, Gutsmann T, Heine H, Schmitz RA, Foligne B. The intestinal archaea methanosphaera stadtmanae and methanobrevibacter smithii activate human dendritic cells. PLoS One. 2014;9(6):e99411. doi:10.1371/journal.pone.0099411.
- Million M, Raoult D. Linking gut redox to human microbiome. Human Microbiome J. 2018;10:27–32. doi:10.1016/j.humic.2018.07.002.
- Million M, Tidjani Alou M, Khelaifia S, Bachar D, Lagier JC, Dione N, Brah S, Hugon P, Lombard V, Armougom F, et al. Increased gut redox and depletion of anaerobic and methanogenic prokaryotes in severe acute malnutrition. Sci Rep. 2016;6(1):26051. doi:10.1038/srep26051.
- Djemai K, Drancourt M, Tidjani Alou M. Bacteria and methanogens in the human microbiome: a review of syntrophic interactions. Microb Ecol. 2022;83(3):536–554. doi:10.1007/s00248-021-01796-7.
- Zhang Y, Brady A, Jones C, Song Y, Darton TC, Jones C, Blohmke CJ, Pollard AJ, Magder LS, Fasano A, et al. Compositional and functional differences in the human gut microbiome correlate with clinical outcome following infection with wild-type salmonella enterica serovar typhi. MBio. 2018;9(3):e00686–e18. doi:10.1128/mBio.00686-18.
- Rhen M. Salmonella and reactive oxygen species: a love-hate relationship. J Innate Immun. 2019;11(3):216. doi:10.1159/000496370.
- De Groote MA, Ochsner UA, Shiloh MU, Nathan C, McCord JM, Dinauer MC, Libby SJ, Vazquez-Torres A, Xu Y, Fang FC. Periplasmic superoxide dismutase protects Salmonella from products of phagocyte NADPH-oxidase and nitric oxide synthase. Proc Natl Acad Sci USA. 1997;94(25):13997–14001. doi:10.1073/pnas.94.25.13997.
- Behnsen J, Perez-Lopez A, Nuccio S-P, Raffatellu M. Exploiting host immunity: the Salmonella paradigm. Trends Immunol. 2015;36(2):112–120. doi:10.1016/j.it.2014.12.003.
- Drumo R, Pesciaroli M, Ruggeri J, Tarantino M, Chirullo B, Pistoia C, Petrucci P, Martinelli N, Moscati L, Manuali E, et al. Salmonella enterica serovar typhimurium exploits inflammation to modify swine intestinal microbiota. Front Cell Infect Microbiol. 2015;5:106. doi:10.3389/fcimb.2015.00106.
- Maier L, Vyas R, Cordova CD, Lindsay H, Schmidt TSB, Brugiroux S, Periaswamy B, Bauer R, Sturm A, Schreiber F, et al. Microbiota-derived hydrogen fuels Salmonella typhimurium invasion of the gut ecosystem. Cell Host Microbe. 2013;14(6):641–651. doi:10.1016/j.chom.2013.11.002.
- Lamichhane-Khadka R, Kwiatkowski A, Maier RJ, Brennan RG. The Hyb hydrogenase permits hydrogen-dependent respiratory growth of salmonella enterica serovar typhimurium. MBio. 2010;1(5):e00284–e10. doi:10.1128/mBio.00284-10.
- Benoit SL, Maier RJ, Sawers RG, Greening C. Molecular hydrogen metabolism: a widespread trait of pathogenic bacteria and protists. Microbiol Mol Biol Rev. 2020;84(1):e00092–e19. doi:10.1128/MMBR.00092-19.
- Schei K, Avershina E, Øien T, Rudi K, Follestad T, Salamati S, Ødegård RA. Early gut mycobiota and mother-offspring transfer. Microbiome. 2017;5(1):107. doi:10.1186/s40168-017-0319-x.
- Willis KA, Purvis JH, Myers ED, Aziz MM, Karabayir I, Gomes CK, Peters BM, Akbilgic O, Talati AJ, Pierre JF. Fungi form interkingdom microbial communities in the primordial human gut that develop with gestational age. FASEB J. 2019;33(11):12825–12837. doi:10.1096/fj.201901436RR.
- Wu X, Xia Y, He F, Zhu C, Ren W. Intestinal mycobiota in health and diseases: from a disrupted equilibrium to clinical opportunities. Microbiome. 2021;9(1):60. doi:10.1186/s40168-021-01024-x.
- Ward TL, Knights D, Gale CA. Infant fungal communities: current knowledge and research opportunities. BMC Med. 2017;15(1):30. doi:10.1186/s12916-017-0802-z.
- Henderickx JGE, de Weerd H, Groot Jebbink LJ, van Zoeren-Grobben D, Hemels MAC, van Lingen RA, Knol J, Belzer C The first fungi: mode of delivery determines early life fungal colonization in the intestine of preterm infants. Microbiome Res Reports. 2022. https://www.oaepublish.com/mrr/article/view/4576.
- Ward TL, Dominguez-Bello MG, Heisel T, Al-Ghalith G, Knights D, Gale CA, Lozupone C. Development of the human mycobiome over the first month of life and across body sites. mSystems. 2018;3(3). doi:10.1128/mSystems.00140-17.
- Arrieta MC, Arévalo A, Stiemsma L, Dimitriu P, Chico ME, Loor S, Vaca M, Boutin RCT, Morien E, Jin M, et al. Associations between infant fungal and bacterial dysbiosis and childhood atopic wheeze in a nonindustrialized setting. J Allergy Clin Immunol. 2018;142(2):424–434.e10. doi:10.1016/j.jaci.2017.08.041.
- Wampach L, Heintz-Buschart A, Hogan A, Muller EEL, Narayanasamy S, Laczny CC, Hugerth LW, Bindl L, Bottu J, Andersson AF, et al. Colonization and succession within the human gut microbiome by archaea, bacteria, and microeukaryotes during the first year of life. Front Microbiol. 2017;8:738. doi:10.3389/fmicb.2017.00738.
- Boutin RCT, Sbihi H, McLaughlin RJ, Hahn AS, Konwar KM, Loo RS, Dai D, Petersen C, Brinkman FSL, Winsor GL, et al. Composition and associations of the infant gut fungal microbiota with environmental factors and childhood allergic outcomes. MBio. 2021;12(3):e0339620. doi:10.1128/mBio.03396-20.
- Gouba N, Drancourt M. Digestive tract mycobiota: a source of infection. Med Mal Infect. 2015;45(1–2):9–16. doi:10.1016/j.medmal.2015.01.007.
- Borges FM, de Paula TO, Sarmiento MRA, de Oliveira MG, Pereira MLM, Toledo V, Nascimento C, Ferreira-Machado B, Silva L, Diniz G. Fungal diversity of human gut microbiota among eutrophic, overweight, and obese individuals based on aerobic culture-dependent approach. Curr Microbiol. 2018;75:726–735. doi:10.1007/s00284-018-1438-8.
- Dworecka-Kaszak B, Dabrowska I, Kaszak I. The mycobiome–a friendly cross-talk between fungal colonizers and their host. Ann Parasitol. 2016;62. https://yadda.icm.edu.pl/yadda/element/bwmeta1.element.agro-6a86c9bc-de56-4d1e-b07e-b04c67c54326.
- Hamad I, Raoult D, Bittar F. Repertory of eukaryotes (eukaryome) in the human gastrointestinal tract: taxonomy and detection methods. Parasite Immunol. 2016;38(1):12–36. doi:10.1111/pim.12284.
- Shuai M, Fu Y, Zhong H-L, Gou W, Jiang Z, Liang Y, Miao Z, Xu JJ, Huynh T, Wahlqvist ML, et al. Mapping the human gut mycobiome in middle-aged and elderly adults: multiomics insights and implications for host metabolic health. Gut. 2022;71(9):1812–1820. doi:10.1136/gutjnl-2021-326298.
- Nash AK, Auchtung TA, Wong MC, Smith DP, Gesell JR, Ross MC, Stewart CJ, Metcalf GA, Muzny DM, Gibbs RA, et al. The gut mycobiome of the human microbiome project healthy cohort. Microbiome. 2017;5(1):153. doi:10.1186/s40168-017-0373-4.
- Hallen-Adams HE, Suhr MJ. Fungi in the healthy human gastrointestinal tract. Virulence. 2017;8(3):352–358. doi:10.1080/21505594.2016.1247140.
- Suhr MJ, Hallen-Adams HE. The human gut mycobiome: pitfalls and potentials–a mycologist’s perspective. Mycologia. 2015;107(6):1057–1073. doi:10.3852/15-147.
- Gouba N, Raoult D, Drancourt M, Sanz Y. Plant and fungal diversity in gut microbiota as revealed by molecular and culture investigations. PLoS One. 2013;8(3):e59474. doi:10.1371/journal.pone.0059474.
- Suhr MJ, Banjara N, Hallen-Adams HE. Sequence-based methods for detecting and evaluating the human gut mycobiome. Lett Appl Microbiol. 2016;62(3):209–215. doi:10.1111/lam.12539.
- Hoffmann C, Dollive S, Grunberg S, Chen J, Li H, Wu GD, Lewis JD, Bushman FD, Pan C. Archaea and fungi of the human gut microbiome: correlations with diet and bacterial residents. PLoS One. 2013;8(6):e66019. doi:10.1371/journal.pone.0066019.
- Zhang F, Aschenbrenner D, Yoo JY, Zuo T. The gut mycobiome in health, disease, and clinical applications in association with the gut bacterial microbiome assembly. Lancet Microbe. 2022;3(12):e969–83. doi:10.1016/S2666-5247(22)00203-8.
- Zuo T, Wong SH, Cheung CP, Lam K, Lui R, Cheung K, Zhang F, Tang W, Ching JYL, Wu JCY, et al. Gut fungal dysbiosis correlates with reduced efficacy of fecal microbiota transplantation in Clostridium difficile infection. Nat Commun. 2018;9(1):3663. doi:10.1038/s41467-018-06103-6.
- Sokol H, Leducq V, Aschard H, Pham HP, Jegou S, Landman C, Cohen D, Liguori G, Bourrier A, Nion-Larmurier I, et al. Fungal microbiota dysbiosis in IBD. Gut. 2017;66(6):1039–1048. doi:10.1136/gutjnl-2015-310746.
- Aykut B, Pushalkar S, Chen R, Li Q, Abengozar R, Kim JI, Shadaloey SA, Wu D, Preiss P, Verma N, et al. The fungal mycobiome promotes pancreatic oncogenesis via activation of MBL. Nature. 2019;574(7777):264–267. doi:10.1038/s41586-019-1608-2.
- Yang AM, Inamine T, Hochrath K, Chen P, Wang L, Llorente C, Bluemel S, Hartmann P, Xu J, Koyama Y, et al. Intestinal fungi contribute to development of alcoholic liver disease. J Clin Invest. 2017;127(7):2829–2841. doi:10.1172/JCI90562.
- Nagpal R, Neth BJ, Wang S, Mishra SP, Craft S, Yadav H. Gut mycobiome and its interaction with diet, gut bacteria and alzheimer’s disease markers in subjects with mild cognitive impairment: a pilot study. EBioMedicine. 2020;59:102950. doi:10.1016/j.ebiom.2020.102950.
- Li XV, Leonardi I, Putzel GG, Semon A, Fiers WD, Kusakabe T, Lin W-Y, Gao IH, Doron I, Gutierrez-Guerrero A, et al. Immune regulation by fungal strain diversity in inflammatory bowel disease. Nature. 2022;603(7902):672–678. doi:10.1038/s41586-022-04502-w.
- Fan D, Coughlin LA, Neubauer MM, Kim J, Kim MS, Zhan X, Simms-Waldrip TR, Xie Y, Hooper LV, Koh AY. Activation of HIF-1α and LL-37 by commensal bacteria inhibits Candida albicans colonization. Nat Med. 2015;21(7):808–814. doi:10.1038/nm.3871.
- Hermann C, Hermann J, Munzel U, Rüchel R. Bacterial flora accompanying Candida yeasts in clinical specimens. Mycoses. 1999;42(11–12):619–627. doi:10.1046/j.1439-0507.1999.00519.x.
- Essoua AML, Dongmo FFD, Koanga MLM, Mouokeu RS, Assam JPA, Ngane RAN, Nintchom VP. Study of coinfection of Salmonella typhi, Salmonella paratyphi with Candida spp., in four hospitals of Douala- Cameroon. Int J Sci Res Arch. 2021;2(2):110–119. doi:10.30574/ijsra.2021.2.2.0041.
- Carlson E. Enhancement by Candida albicans of Staphylococcus aureus, Serratia marcescens, and Streptococcus faecalis in the establishment of infection in mice. Infect Immun. 1983;39(1):193–197. doi:10.1128/iai.39.1.193-197.1983.
- Niu X-X, Li T, Zhang X, Wang S-X, Liu Z-H. Lactobacillus crispatus modulates vaginal epithelial cell innate response to Candida albicans. Chin Med J. 2017;130(3):273–279. doi:10.4103/0366-6999.198927.
- Pattaroni C, Macowan M, Chatzis R, Daunt C, Custovic A, Shields MD, Power UF, Grigg J, Roberts G, Ghazal P, et al. Early life inter-kingdom interactions shape the immunological environment of the airways. Microbiome. 2022;10(1):34. doi:10.1186/s40168-021-01201-y.
- Todd OA, Noverr MC, Peters BM, Mitchell AP. Candida albicans impacts staphylococcus aureus alpha-toxin production via extracellular alkalinization. mSphere. 2019;4(6). doi:10.1128/mSphere.00780-19.
- Todd OA, PL F Jr, Harro JM, Hilliard JJ, Tkaczyk C, Sellman BR, Noverr MC, Peters BM, Lorenz M. Candida albicans augments Staphylococcus aureus virulence by engaging the Staphylococcal agr quorum sensing system. MBio. 2019;10(3). doi:10.1128/mBio.00910-19.
- Pothoulakis C, Kelly CP, Joshi MA, Gao N, O’Keane CJ, Castagliuolo I, Lamont JT. Saccharomyces boulardii inhibits Clostridium difficile toxin a binding and enterotoxicity in rat ileum. Gastroenterology. 1993;104(4):1108–1115. doi:10.1016/0016-5085(93)90280-P.
- Castagliuolo I, LaMont JT, Nikulasson ST, Pothoulakis C. Saccharomyces boulardii protease inhibits Clostridium difficile toxin a effects in the rat ileum. Infect Immun. 1996;64(12):5225–5232. doi:10.1128/iai.64.12.5225-5232.1996.
- McFarland LV, Surawicz CM, Greenberg RN, Fekety R, Elmer GW, Moyer KA, Melcher SA, Bowen KE, Cox JL, Noorani Z. A randomized placebo-controlled trial of Saccharomyces boulardii in combination with standard antibiotics for Clostridium difficile disease. JAMA. 1994;271(24):1913–1918. doi:10.1001/jama.1994.03510480037031.
- Santus W, Devlin JR, Behnsen J, Ottemann KM. Crossing kingdoms: how the mycobiota and fungal-bacterial interactions impact host health and disease. Infect Immun. 2021;89(4). doi:10.1128/IAI.00648-20.
- Li Y, Wang K, Zhang B, Tu Q, Yao Y, Cui B, Ren B, He J, Shen X, Van Nostrand JD, et al. Salivary mycobiome dysbiosis and its potential impact on bacteriome shifts and host immunity in oral lichen planus. Int J Oral Sci. 2019;11(2):13. doi:10.1038/s41368-019-0045-2.
- Grimaudo NJ, Nesbitt WE. Coaggregation of Candida albicans with oral Fusobacterium species. Oral Microbiol Immunol. 1997;12(3):168–173. doi:10.1111/j.1399-302X.1997.tb00374.x.
- Bachtiar EW, Bachtiar BM, Jarosz LM, Amir LR, Sunarto H, Ganin H, Meijler MM, Krom BP. AI-2 of Aggregatibacter actinomycetemcomitans inhibits Candida albicans biofilm formation. Front Cell Infect Microbiol. 2014;4. doi:10.3389/fcimb.2014.00094.
- Kraneveld EA, Buijs MJ, Bonder MJ, Visser M, Keijser BJF, Crielaard W, Zaura E, Sturtevant J. The relation between oral Candida load and bacterial microbiome profiles in Dutch older adults. PLoS One. 2012;7(8):e42770. doi:10.1371/journal.pone.0042770.
- Noverr MC, Huffnagle GB. Regulation of Candida albicans morphogenesis by fatty acid metabolites. Infect Immun. 2004;72(11):6206–6210. doi:10.1128/IAI.72.11.6206-6210.2004.
- Pang Y, Zhang H, Wen H, Wan H, Wu H, Chen Y, Li S, Zhang L, Sun X, Li B, et al. Yeast probiotic and yeast products in enhancing livestock feeds utilization and performance: an overview. J Fungi (Basel). 2022;8(11):1191. doi:10.3390/jof8111191.
- Pontier-Bres R, Munro P, Boyer L, Anty R, Imbert V, Terciolo C, André F, Rampal P, Lemichez E, Peyron J-F, et al. Saccharomyces boulardii modifies Salmonella typhimurium traffic and host immune responses along the intestinal tract. PLoS One. 2014;9(8):e103069. doi:10.1371/journal.pone.0103069.
- Gedek BR. original Articles. Mycoses. 1999;42(4):261–264. doi:10.1046/j.1439-0507.1999.00449.x.
- Tiago FCP, Martins FS, Souza ELS, Pimenta PFP, Araujo HRC, Castro IM, Brandão RL, Nicoli JR. Adhesion to the yeast cell surface as a mechanism for trapping pathogenic bacteria by Saccharomyces probiotics. J Med Microbiol. 2012;61(9):1194–1207. doi:10.1099/jmm.0.042283-0.
- Kernien JF, Snarr BD, Sheppard DC, Nett JE. The interface between fungal biofilms and innate immunity. Front Immunol. 2018;8. doi:10.3389/fimmu.2017.01968.
- Wall G, Lopez-Ribot JL. Current antimycotics, new prospects, and future approaches to antifungal therapy. Antibiotics. 2020;9(8):445. doi:10.3390/antibiotics9080445.
- Hager CL, Isham N, Schrom KP, Chandra J, McCormick T, Miyagi M, Ghannoum MA, Karim SA. Effects of a novel probiotic combination on pathogenic bacterial-fungal polymicrobial biofilms. mBio. 2019;10(2). doi:10.1128/mbio.00338-19.
- Hoarau G, Mukherjee PK, Gower-Rousseau C, Hager C, Chandra J, Retuerto MA, Neut C, Vermeire S, Clemente J, Colombel JF, et al. Bacteriome and mycobiome interactions underscore microbial dysbiosis in familial Crohn’s disease. mBio. 2016;7(5). doi: 10.1128/mbio.01250-16.
- Kalan L, Loesche M, Hodkinson BP, Heilmann K, Ruthel G, Gardner SE, Grice EA, Huffnagle GB. Redefining the chronic-wound microbiome: fungal communities are prevalent, dynamic, and associated with delayed healing. mBio. 2016;7(5). doi:10.1128/mbio.01058-16.
- Kong EF, Tsui C, Kucharíková S, Van Dijck P, Jabra-Rizk MA. Modulation of Staphylococcus aureus response to antimicrobials by the Candida albicans quorum sensing molecule farnesol. Antimicrob Agents Chemother. 2017;61(12):61. doi:10.1128/AAC.01573-17.
- Jabra-Rizk MA, Meiller TF, James CE, Shirtliff ME. Effect of farnesol on Staphylococcus aureus biofilm formation and antimicrobial susceptibility. Antimicrob Agents Chemother. 2006;50(4):1463–1469. doi:10.1128/AAC.50.4.1463-1469.2006.
- De Sordi L, Mühlschlegel FA. Quorum sensing and fungal-bacterial interactions in Candida albicans: a communicative network regulating microbial coexistence and virulence. FEMS Yeast Res. 2009;9(7):990–999. doi:10.1111/j.1567-1364.2009.00573.x.
- Peleg AY, Tampakakis E, Fuchs BB, Eliopoulos GM, Moellering RC, Mylonakis E Prokaryote–eukaryote interactions identified by using Caenorhabditis elegans. Proc Natl Acad Sci 2008; 105:14585–14590. doi:10.1073/pnas.0805048105
- Kostoulias X, Murray GL, Cerqueira GM, Kong JB, Bantun F, Mylonakis E, Khoo CA, Peleg AY. Impact of a cross-kingdom signaling molecule of Candida albicans on acinetobacter baumannii physiology. Antimicrob Agents Chemother. 2016;60(1):161–167. doi:10.1128/AAC.01540-15.
- Smith MG, Des Etages SG, Snyder M. Microbial synergy via an ethanol-triggered pathway. Mol Cell Biol. 2004;24(9):3874–3884. doi:10.1128/MCB.24.9.3874-3884.2004.
- Castagliuolo I, Riegler MF, Valenick L, LaMont JT, Pothoulakis C, Kozel TR. Saccharomyces boulardii protease inhibits the effects of Clostridium difficile toxins a and B in human colonic mucosa. Infect Immun. 1999;67(1):302–307. doi:10.1128/IAI.67.1.302-307.1999.
- Brandão RL, Castro IM, Bambirra EA, Amaral SC, Fietto LG, Tropia MJM, Neves MJ, Dos Santos RG, Gomes NCM, Nicoli JR. Intracellular signal triggered by cholera toxin in Saccharomyces boulardii and Saccharomyces cerevisiae. Appl Environ Microbiol. 1998;64(2):564–568. doi:10.1128/aem.64.2.564-568.1998.
- Siavoshi F, Saniee P. Vacuoles of Candida yeast as a specialized niche for Helicobacter pylori. World J Gastroenterol. 2014;20(18):5263–5273. doi:10.3748/wjg.v20.i18.5263.
- van Leeuwen PT, van der Peet JM, Bikker FJ, Hoogenkamp MA, Oliveira Paiva AM, Kostidis S, Mayboroda OA, Smits WK, Krom BP, Imperiale MJ. Interspecies Interactions between Clostridium difficile and Candida albicans. mSphere. 2016;1. doi:10.1128/mSphere.00187-16.
- Lambooij JM, Hoogenkamp MA, Brandt BW, Janus MM, Krom BP. Fungal mitochondrial oxygen consumption induces the growth of strict anaerobic bacteria. Fungal Genet Biol. 2017;109:1–6. doi:10.1016/j.fgb.2017.10.001.
- Romo JA, Markey L, Kumamoto CA. Lipid species in the GI tract are increased by the commensal fungus candida albicans and decrease the virulence of clostridioides difficile. J Fungi (Basel). 2020;6(3):100. doi:10.3390/jof6030100.
- Santus W, Rana AP, Devlin JR, Kiernan KA, Jacob CC, Tjokrosurjo J, Underhill DM, Behnsen J. Mycobiota and diet-derived fungal xenosiderophores promote Salmonella gastrointestinal colonization. Nat Microbiol. 2022;7(12):2025–2038. doi:10.1038/s41564-022-01267-w.
- Gantner BN, Simmons RM, Canavera SJ, Akira S, Underhill DM. Collaborative induction of inflammatory responses by dectin-1 and Toll-like receptor 2. J Exp Med. 2003;197(9):1107–1117. doi:10.1084/jem.20021787.
- Da Silva CA, Chalouni C, Williams A, Hartl D, Lee CG, Elias JA. Chitin is a size-dependent regulator of macrophage TNF and IL-10 production. J Immunol. 2009;182(6):3573–3582. doi:10.4049/jimmunol.0802113.
- Fuchs K, Cardona Gloria Y, Wolz OO, Herster F, Sharma L, Dillen CA, Täumer C, Dickhöfer S, Bittner Z, Dang TM, et al. The fungal ligand chitin directly binds TLR2 and triggers inflammation dependent on oligomer size. EMBO Rep. 2018;19(12):e46065. doi:10.15252/embr.201846065.
- Netea MG, Gow NAR, Munro CA, Bates S, Collins C, Ferwerda G, Hobson RP, Bertram G, Hughes HB, Jansen T, et al. Immune sensing of Candida albicans requires cooperative recognition of mannans and glucans by lectin and Toll-like receptors. J Clin Invest. 2006;116(6):1642–1650. doi:10.1172/JCI27114.
- McGreal EP, Rosas M, Brown GD, Zamze S, Wong SYC, Gordon S, Martinez-Pomares L, Taylor PR. The carbohydrate-recognition domain of Dectin-2 is a C-type lectin with specificity for high mannose. Glycobiology. 2006;16(5):422–430. doi:10.1093/glycob/cwj077.
- Yamasaki S, Matsumoto M, Takeuchi O, Matsuzawa T, Ishikawa E, Sakuma M, Tateno H, Uno J, Hirabayashi J, Mikami Y, et al. C-type lectin Mincle is an activating receptor for pathogenic fungus, Malassezia. Proc Natl Acad Sci USA. 2009;106(6):1897–1902. doi:10.1073/pnas.0805177106.
- Rizzetto L, Ifrim DC, Moretti S, Tocci N, Cheng SC, Quintin J, Renga G, Oikonomou V, De Filippo C, Weil T, et al. Fungal chitin induces trained immunity in human monocytes during cross-talk of the host with Saccharomyces cerevisiae. J Biol Chem. 2016;291(15):7961–7972. doi:10.1074/jbc.m115.699645.
- Leonardi I, Gao IH, Lin WY, Allen M, Li XV, Fiers WD, De CMB, Putzel GG, Yantiss RK, Johncilla M, et al. Mucosal fungi promote gut barrier function and social behavior via Type 17 immunity. Cell. 2022;185(5):831–846.e14. doi:10.1016/j.cell.2022.01.017.
- Shao TY, Gladys Ang WX, Jiang TT, Huang FS, Andersen H, Kinder JM, Pham G, Burg AR, Ruff B, Gonzalez T, et al. Commensal Candida albicans positively calibrates systemic Th17 immunological responses. Cell Host Microbe. 2019;25(3):404–417.e6. doi:10.1016/j.chom.2019.02.004.
- Yeung F, Chen YH, Lin JD, Leung JM, McCauley C, Devlin JC, Hansen C, Cronkite A, Stephens Z, Drake-Dunn C, et al. Altered immunity of laboratory mice in the natural environment is associated with fungal colonization. Cell Host Microbe. 2020;27(5):809–822.e6. doi:10.1016/j.chom.2020.02.015.
- Paterson MJ, Oh S, Underhill DM. Host–microbe interactions: commensal fungi in the gut. Curr Opin Microbiol. 2017;40:131–137. doi:10.1016/j.mib.2017.11.012.
- Zhang Z, Li J, Zheng W, Zhao G, Zhang H, Wang X, Guo Y, Qin C, Shi Y. Peripheral lymphoid volume expansion and maintenance are controlled by gut microbiota via RALDH+ Dendritic cells. Immunity. 2016;44(2):330–342. doi:10.1016/j.immuni.2016.01.004.
- Acovic A, Markovic BS, Gazdic M, Arsenijevic A, Jovicic N, Gajovic N, Jovanovic M, Zdravkovic N, Kanjevac T, Randall Harrell C, et al. Indoleamine 2,3-dioxygenase-dependent expansion of T-regulatory cells maintains mucosal healing in ulcerative colitis. Therap Adv Gastroenterol. 2018;11:175628481879355. doi:10.1177/1756284818793558.
- Bonifazi P, Zelante T, D’Angelo C, De Luca A, Moretti S, Bozza S, Perruccio K, Iannitti RG, Giovannini G, Volpi C, et al. Balancing inflammation and tolerance in vivo through dendritic cells by the commensal Candida albicans. Mucosal Immunol. 2009;2(4):362–374. doi:10.1038/mi.2009.17.
- Markey L, Shaban L, Green ER, Lemon KP, Mecsas J, Kumamoto CA. Pre-colonization with the commensal fungus Candida albicansreduces murine susceptibility to Clostridium difficile infection. Gut Microbes. 2018;1–13. doi:10.1080/19490976.2018.1465158.
- Wheeler ML, Limon JJ, Bar AS, Leal CA, Gargus M, Tang J, Brown J, Funari VA, Wang HL, Crother TR, et al. Immunological consequences of intestinal fungal dysbiosis. Cell Host Microbe. 2016;19(6):865–873. doi:10.1016/j.chom.2016.05.003.
- van Tvan Bernardes ET, van Tilburg Bernardes E, Pettersen VK, Gutierrez MW, Laforest-Lapointe I, Jendzjowsky NG, Cavin J-B, Vicentini FA, Keenan CM, Ramay HR, et al. Intestinal fungi are causally implicated in microbiome assembly and immune development in mice. Nat Commun. 2020;11(1). doi: 10.1038/s41467-020-16431-1.
- Mason KL, Erb Downward JR, Falkowski NR, Young VB, Kao JY, Huffnagle GB, Deepe GS. Interplay between the Gastric Bacterial Microbiota and Candida albicans during Postantibiotic Recolonization and Gastritis. Infect Immun. 2012;80(1):150–158. doi:10.1128/iai.05162-11.
- Downward JRE, Erb Downward JR, Falkowski NR, Mason KL, Muraglia R, Huffnagle GB. Modulation of post-antibiotic bacterial community reassembly and host response by Candida albicans. Sci Rep. 2013;3(1). doi:10.1038/srep02191.
- Baron S, editor. Medical microbiology. 4th ed. Galveston (TX): University of Texas Medical Branch at Galveston; 1996.
- Cummings RD, Hokke CH, Haslam SM. Parasitic Infections. In: Varki A, Cummings RD, Esko JD, Stanley P, Hart GW, Aebi M, Mohnen D, Kinoshita T, Packer NH, Prestegard J, editors. Essentials of glycobiology. Cold Spring Harbor (NY): Cold Spring Harbor Laboratory Press; 2015.
- Haque R. Human intestinal parasites. J Health Popul Nutr. 2007;25:387–391.
- Ianiro G, Iorio A, Porcari S, Masucci L, Sanguinetti M, Perno CF, Gasbarrini A, Putignani L, Cammarota G. How the gut parasitome affects human health. Therap Adv Gastroenterol. 2022;15:17562848221091524. doi:10.1177/17562848221091524.
- Marzano V, Mancinelli L, Bracaglia G, Del Chierico F, Vernocchi P, Di Girolamo F, Garrone S, Tchidjou Kuekou H, D’Argenio P, Dallapiccola B, et al. “Omic” investigations of protozoa and worms for a deeper understanding of the human gut “parasitome”. PLoS Negl Trop Dis. 2017;11(11):e0005916. doi:10.1371/journal.pntd.0005916.
- Scanlan PD, Marchesi JR. Micro-eukaryotic diversity of the human distal gut microbiota: qualitative assessment using culture-dependent and -independent analysis of faeces. ISME J. 2008;2(12):1183–1193. doi:10.1038/ismej.2008.76.
- Morton ER, Lynch J, Froment A, Lafosse S, Heyer E, Przeworski M, Blekhman R, Ségurel L, Benson A. Variation in Rural African gut microbiota is strongly correlated with colonization by entamoeba and subsistence. PLoS Genet. 2015;11(11):e1005658. doi:10.1371/journal.pgen.1005658.
- Chabé M, Lokmer A, Ségurel L. Gut Protozoa: friends or Foes of the human gut microbiota? Trends Parasitol. 2017;33(12):925–934. doi:10.1016/j.pt.2017.08.005.
- Li LH, Zhang XP, Lv S, Zhang L, Yoshikawa H, Wu Z, Steinmann P, Utzinger J, Tong XM, Chen SH, et al. Cross-sectional surveys and subtype classification of human Blastocystis isolates from four epidemiological settings in China. Parasitol Res. 2007;102(1):83–90. doi:10.1007/s00436-007-0727-0.
- Hirata T, Nakamura H, Kinjo N, Hokama A, Kinjo F, Yamane N, Fujita J. Prevalence of Blastocystis hominis and Strongyloides stercoralis infection in Okinawa, Japan. Parasitol Res. 2007;101(6):1717–1719. doi:10.1007/s00436-007-0712-7.
- Wong KHS, Ng GC, Lin RTP, Yoshikawa H, Taylor MB, Tan KSW. Predominance of subtype 3 among Blastocystis isolates from a major hospital in Singapore. Parasitol Res. 2008;102(4):663–670. doi:10.1007/s00436-007-0808-0.
- Bart A, Wentink-Bonnema EMS, Gilis H, Verhaar N, Wassenaar CJA, van Vugt M, Goorhuis A, van Gool T. Diagnosis and subtype analysis of Blastocystis sp. in 442 patients in a hospital setting in the Netherlands. BMC Infect Dis. 2013;13(1):389. doi:10.1186/1471-2334-13-389.
- Lukeš J, Stensvold CR, Jirků-Pomajbíková K, Wegener Parfrey L, Knoll LJ. Are human intestinal eukaryotes beneficial or commensals? PLoS Pathog. 2015;11(8):e1005039. doi:10.1371/journal.ppat.1005039.
- Jokelainen P, Hebbelstrup Jensen B, Andreassen BU, Petersen AM, Röser D, Krogfelt KA, Nielsen HV, Stensvold CR, Gilligan P. Dientamoeba fragilis, a commensal in children in Danish day care centers. J Clin Microbiol. 2017;55(6):1707–1713. doi:10.1128/JCM.00037-17.
- El Safadi D, Gaayeb L, Meloni D, Cian A, Poirier P, Wawrzyniak I, Delbac F, Dabboussi F, Delhaes L, Seck M, et al. Children of Senegal River Basin show the highest prevalence of Blastocystis sp. ever observed worldwide. BMC Infect Dis. 2014;14(1):164. doi:10.1186/1471-2334-14-164.
- Eckburg PB, Bik EM, Bernstein CN, Purdom E, Dethlefsen L, Sargent M, Gill SR, Nelson KE, Relman DA. Diversity of the human intestinal microbial flora. Science. 2005;308(5728):1635–1638. doi:10.1126/science.1110591.
- Houlden A, Hayes KS, Bancroft AJ, Worthington JJ, Wang P, Grencis RK, Roberts IS, Kim CH. Chronic trichuris muris infection in C57BL/6 mice causes significant changes in host microbiota and metabolome: effects reversed by pathogen clearance. PLoS One. 2015;10(5):e0125945. doi:10.1371/journal.pone.0125945.
- White EC, Houlden A, Bancroft AJ, Hayes KS, Goldrick M, Grencis RK, Roberts IS. Manipulation of host and parasite microbiotas: survival strategies during chronic nematode infection. Sci Adv. 2018;4(3):eaap7399. doi:10.1126/sciadv.aap7399.
- Giacomin P, Croese J, Krause L, Loukas A, Cantacessi C. Suppression of inflammation by helminths: a role for the gut microbiota? Philos Trans R Soc Lond B Biol Sci. 2015;370:20140296. doi:10.1098/rstb.2014.0296.
- Ramanan D, Bowcutt R, Lee SC, Tang MS, Kurtz ZD, Ding Y, Honda K, Gause WC, Blaser MJ, Bonneau RA, et al. Helminth infection promotes colonization resistance via type 2 immunity. Science. 2016;352(6285):608–612. doi:10.1126/science.aaf3229.
- Garn H, Potaczek DP, Pfefferle PI. The hygiene hypothesis and new perspectives-current challenges meeting an old postulate. Front Immunol. 2021;12:637087. doi:10.3389/fimmu.2021.637087.
- Lynch NR, Hagel IA, Palenque ME, Di Prisco MC, Escudero JE, Corao LA, Sandia JA, Ferreira LJ, Botto C, Perez M, et al. Relationship between helminthic infection and IgE response in atopic and nonatopic children in a tropical environment. J Allergy Clin Immunol. 1998;101(2):217–221. doi:10.1016/S0091-6749(98)70386-0.
- Berrilli F, Di Cave D, Cavallero S, D’Amelio S. Interactions between parasites and microbial communities in the human gut. Front Cell Infect Microbiol. 2012. doi:10.3389/fcimb.2012.00141.
- Ryan SM, Eichenberger RM, Ruscher R, Giacomin PR, Loukas A, Lok JB. Harnessing helminth-driven immunoregulation in the search for novel therapeutic modalities. PLoS Pathog. 2020;16(5):e1008508. doi:10.1371/journal.ppat.1008508.
- Shi W, Xu N, Wang X, Vallée I, Liu M, Liu X. Helminth therapy for immune-mediated inflammatory diseases: current and future perspectives. J Inflamm Res. 2022;15:475–491. doi:10.2147/JIR.S348079.
- Mbuyi-Kalonji L, Barbé B, Nkoji G, Madinga J, Roucher C, Linsuke S, Hermy M, Heroes A-S, Mattheus W, Polman K, et al. Non-typhoidal Salmonella intestinal carriage in a Schistosoma mansoni endemic community in a rural area of the Democratic Republic of Congo. PLoS Negl Trop Dis. 2020;14(2):e0007875. doi:10.1371/journal.pntd.0007875.
- Fletcher SM, Stark D, Harkness J, Ellis J. Enteric protozoa in the developed world: a public health perspective. Clin Microbiol Rev. 2012;25(3):420–449. doi:10.1128/CMR.05038-11.
- Beyene G, Tasew H. Prevalence of intestinal parasite, Shigella and Salmonella species among diarrheal children in Jimma health center, Jimma southwest Ethiopia: a cross sectional study. Ann Clin Microbiol Antimicrob. 2014;13(1):10. doi:10.1186/1476-0711-13-10.
- Desenclos JC, Zergabachew A, Desmoulins B, Chouteau L, Desve G, Admassu M. Clinical, microbiological and antibiotic susceptibility patterns of diarrhoea in Korem, Ethiopia. J Trop Med Hyg. 1988;91:296–301.
- Huruy K, Kassu A, Mulu A, Worku N, Fetene T, Gebretsadik S, Biadglegne F, Belyhun Y, Muche A, Gelaw A, et al. Intestinal parasitosis and shigellosis among diarrheal patients in Gondar teaching hospital, northwest Ethiopia. BMC Res Notes. 2011;4(1):472. doi:10.1186/1756-0500-4-472.
- Galván-Moroyoqui JM, Domínguez-Robles MDC, Franco E, Meza I, Sinnis P. The interplay between entamoeba and enteropathogenic bacteria modulates epithelial cell damage. PLoS Negl Trop Dis. 2008;2(7):e266. doi:10.1371/journal.pntd.0000266.
- Reynolds LA, Redpath SA, Yurist-Doutsch S, Gill N, Brown EM, van der Heijden J, Brosschot TP, Han J, Marshall NC, Woodward SE, et al. Enteric helminths promote Salmonella coinfection by altering the intestinal metabolome. J Infect Dis. 2017;215(8):1245–1254. doi:10.1093/infdis/jix141.
- Bajinka O, Qi M, Barrow A, Touray AO, Yang L, Tan Y. Pathogenicity of Salmonella during Schistosoma-Salmonella Co-infections and the importance of the gut microbiota. Curr Microbiol. 2021;79(1):26. doi:10.1007/s00284-021-02718-z.
- Brosschot TP, Lawrence KM, Moeller BE, Kennedy MHE, FitzPatrick RD, Gauthier CM, Shin D, Gatti DM, Conway KME, Reynolds LA, et al. Impaired host resistance to Salmonella during helminth co-infection is restored by anthelmintic treatment prior to bacterial challenge. PLoS Negl Trop Dis. 2021;15(1):e0009052. doi:10.1371/journal.pntd.0009052.
- Chudnovskiy A, Mortha A, Kana V, Kennard A, Ramirez JD, Rahman A, Remark R, Mogno I, Ng R, Gnjatic S, et al. Host-protozoan interactions protect from mucosal infections through activation of the inflammasome. Cell. 2016;167(2):444–456.e14. doi:10.1016/j.cell.2016.08.076.
- Schramm G, Suwandi A, Galeev A, Sharma S, Braun J, Claes A-K, Braubach P, Grassl GA. Schistosome eggs impair protective Th1/Th17 immune responses against salmonella infection. Front Immunol. 2018;9:2614. doi:10.3389/fimmu.2018.02614.
- Lokken KL, Stull-Lane AR, Poels K, Tsolis RM, Raffatellu M. Malaria parasite-mediated alteration of macrophage function and increased iron availability predispose to disseminated nontyphoidal Salmonella infection. Infect Immun. 2018;86(9). doi:10.1128/IAI.00301-18.
- Walker AJ. Insights into the functional biology of schistosomes. Parasit Vectors. 2011;4(1):203. doi:10.1186/1756-3305-4-203.
- Walker GT, Yang G, Tsai JY, Rodriguez JL, English BC, Faber F, Souvannaseng L, Butler BP, Tsolis RM. Malaria parasite infection compromises colonization resistance to an enteric pathogen by reducing gastric acidity. Sci Adv. 2021;7(27). doi:10.1126/sciadv.abd6232.
- Dewhirst FE, Chien C-C, Paster BJ, Ericson RL, Orcutt RP, Schauer DB, Fox JG. Phylogeny of the defined murine microbiota: altered schaedler flora. Appl Environ Microbiol. 1999;65(8):3287–3292. doi:10.1128/AEM.65.8.3287-3292.1999.
- Schaedler RW, Dubs R, Costello R. Association of germfree mice with bacteria isolated from normal mice. J Exp Med. 1965;122(1):77–82. doi:10.1084/jem.122.1.77.
- Wymore Brand M, Wannemuehler MJ, Phillips GJ, Proctor A, Overstreet A-M, Jergens AE, Orcutt RP, Fox JG. The altered schaedler flora: continued applications of a defined murine microbial community. ILAR J. 2015;56(2):169–178. doi:10.1093/ilar/ilv012.
- Bolsega S, Bleich A, Basic M. Synthetic microbiomes on the rise—application in deciphering the role of microbes in host health and disease. Nutrients. 2021;13(11):4173. doi:10.3390/nu13114173.
- Stecher B. Establishing causality in Salmonella-microbiota-host interaction: the use of gnotobiotic mouse models and synthetic microbial communities. Int J Med Microbiol. 2021;311(3):151484. doi:10.1016/j.ijmm.2021.151484.
- Lagkouvardos I, Pukall R, Abt B, Foesel BU, Meier-Kolthoff JP, Kumar N, Bresciani A, Martínez I, Just S, Ziegler C, et al. The Mouse Intestinal Bacterial Collection (miBC) provides host-specific insight into cultured diversity and functional potential of the gut microbiota. Nat microbiol. 2016;1(10):16131. doi:10.1038/nmicrobiol.2016.131.
- Chiaro TR, Soto R, Zac Stephens W, Kubinak JL, Petersen C, Gogokhia L, Bell R, Delgado JC, Cox J, Voth W, et al. A member of the gut mycobiota modulates host purine metabolism exacerbating colitis in mice. Sci Transl Med. 2017;9(380). doi: 10.1126/scitranslmed.aaf9044.
- Deng L, Wojciech L, Png CW, Koh EY, Aung TT, Kioh DYQ, Chan ECY, Malleret B, Zhang Y, Peng G, et al. Experimental colonization with Blastocystis ST4 is associated with protective immune responses and modulation of gut microbiome in a DSS-induced colitis mouse model. Cell Mol Life Sci. 2022;79(5):245. doi:10.1007/s00018-022-04271-9.
- Iliev ID, Cadwell K. Effects of intestinal fungi and viruses on immune responses and inflammatory bowel diseases. Gastroenterology. 2021;160(4):1050–1066. doi:10.1053/j.gastro.2020.06.100.