ABSTRACT
A high-fat (HF) diet reduces resistance to the foodborne pathogen Listeria monocytogenes. We demonstrate that short-term gavage with A. muciniphila increases resistance to oral and systemic L. monocytogenes infection in mice fed a HF diet. A. muciniphila reduced inflammation in the gut and liver of mice fed a high-fat diet prior to infection and reduced inflammatory cell infiltration in the ileum to levels similar to mice fed a low-fat (LF) diet. Akkermansia administration had minimal impacts upon the microbiota and microbial metabolites and did not affect individual taxa or impact the Bacteroidetes to Firmicutes ratio. In summary, A. muciniphila increased resistance to L. monocytogenes infection in mice fed a HF diet by moderating immune/physiological effects through specific interaction between A. muciniphila and the host gut.
Introduction
A Westernized diet in which a high proportion of caloric intake is derived from animal fats is known to influence microbiota community structure and immune-inflammatory responses in the host.Citation1,Citation2 As well as being associated with the development of obesity, type 2 diabetes and inflammatory bowel disease, this diet can also increase susceptibility to foodborne pathogens including Salmonella Typhimurium and Listeria monocytogenes in animal models.Citation1,Citation3,Citation4 We previously demonstrated that a high-fat (HF) diet induces inflammatory gene expression in the gut prior to Listeria exposure and increases susceptibility to oral and systemic Listeria infection.Citation3 This may be due to an increased number of goblet cells in the colonic mucosa of these mice which are known to facilitate interaction between listerial Internalin A and host E-cadherin and MET. Other broader effects on immune/inflammatory responses were also evident.Citation5 HF diet also affected the gut microbiota in this model, causing a predictable increase in levels of Firmicutes (including Coprococcus, Butyricicoccus, Turicibacter and Clostridium XIVa) and a reduction in Verrucomicrobiota, with these alterations to the gut microbiota subsequently exaggerated by infection with Listeria.Citation3
The genus Akkermansia is a member of the phylum Verrucomicrobiota, whose type species is A. muciniphila.Citation6 This aero-tolerant, oval-shaped, Gram-negative bacterium makes up 1–4% of the human microbiome.Citation7 It uses mucin as sole source of carbon and nitrogen which allows A. muciniphila to thrive in nutrient-depleted environments.Citation6,Citation8 Metagenomic comparisons suggest that at least eight Akkermansia species can be found in the human microbiome, and concurrent colonization with different strains can occur.Citation9 A. muciniphila localizes to the mucus layer especially in the cecum, closely associates with intestinal cells,Citation10 and is predicted to produce 61 mucin-degrading proteins.Citation11 It produces the short chain fatty acids acetic acid and propionateCitation6 and facilitates the production of butyrate by other bacteria,Citation12 which can have positive impacts on gut health.Citation13 A. muciniphila has been shown to positively modulate host immune responsesCitation14 and gut barrier functionCitation10 and a reduced abundance later in life is associated with increased inflammation and reduced barrier function which can be rectified by butyrate supplementation.Citation15,Citation16 A. muciniphila is consistently reduced in humans or mice consuming a high-fat/westernized diet.Citation17–19 It has been associated with a lean phenotype and reduced body weight gain.Citation14 It inversely correlated with diseases such as ulcerative colitis and Crohn’s disease and has been suggested as an indicator of a healthy intestine.Citation20 It may also ameliorate the metabolic disorders associated with consumption of HF diets and obesity.Citation17
Based on these findings, we have investigated the effect of A. muciniphila on L. monocytogenes infection in the context of HF diet. Here we demonstrate that oral gavage with A. muciniphila enhances resistance to L. monocytogenes in mice fed a HF diet. Measured at the timepoint prior to Listeria infection, exposure to A. muciniphila ameliorated inflammatory gene expression in the distal ileum and this was coincident with a reduction in inflammatory cell infiltration. Microbiome analysis identified changes that were primarily driven by the diet, while global microbial metabolomics data failed to identify changes induced specifically by A. muciniphila. To our knowledge this is the first report of the effects of A. muciniphila against a foodborne pathogen and the results have implications for the use of microbial interventions in the prevention of foodborne infectious disease.
Results
A. muciniphila enhances resistance to Listeria monocytogenes infection in mice fed a high-fat diet
A previous study from our group identified a higher susceptibility to Listeria infection in mice fed a HF-diet.Citation3 The current study was designed to permit analysis of events occurring in mice directly before Listeria infection (day 13 sampling) and during Listeria infection (day 16 sampling) ().
Figure 1. High-fat diet increased susceptibility to oral and systemic Listeria monocytogenes infection, but this increase was absent in mice treated with A. muciniphila.
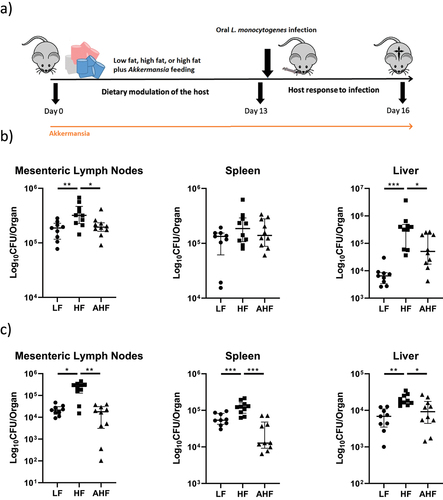
Similar to our previous study,Citation3 a HF diet significantly increased infectious load in the liver and mesenteric lymph nodes compared to LF mice following intragastric (IG) gavage with the pathogen. However, this increase was not observed in mice fed a HF diet plus A. muciniphila gavage (the Akkermansia HF (AHF) group) (). When we investigated the effect of diet and A. muciniphila treatment on systemic Listeria infection, the bacterial load was significantly increased in the spleen, liver, and mesenteric lymph nodes of HF mice compared to LF mice, and this increase was again absent in the AHF group (). Overall, the data demonstrate that A. muciniphila functionally improves resistance to L. monocytogenes infection in mice fed a HF diet.
A. muciniphila reduces ileal inflammation and goblet cell production but elevates expression of hepatic immune genes in mice fed a high fat diet
To investigate possible mechanisms by which A. muciniphila may enhance resistance to L. monocytogenes infection in our model, we focused upon changes apparent on day 13, the period directly prior to infection. Changes in host gene expression due to diet, with and without A. muciniphila, were measured by qPCR in the distal ileum and liver (). Expression of immune genes Tnfα, Foxp3 and Ccl2 was significantly increased in the ileum of mice fed a HF diet compared to LF, consistent with previous studies,Citation3 and this increase was absent in HF mice administered A. muciniphila (). In addition, Il1β expression was increased in the ileum of HF mice compared to AHF, while Reg3γ expression was increased in HF relative to LF ().
Figure 2. A. muciniphila reduces inflammatory expression and goblet cell numbers in the ileum while exacerbating hepatic immune expression.
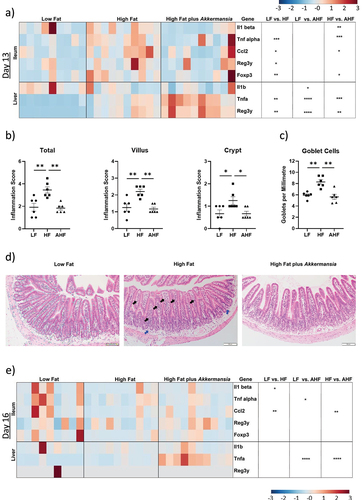
Histological analysis using H&E-stained ileal sections revealed that ileal inflammation, characterized by poly- and mononuclear cells, was increased by HF diet (as previously demonstratedCitation3). However, this infiltration was not observed in mice treated with A. muciniphila (). This suggests that A. muciniphila intervention can moderate inflammation in the small intestine of mice fed a short-term HF diet and that this is coincident with improved resistance to infection. Histological analysis also revealed an increase in goblet cell number in the HF diet group, which was again absent in the AHF group ().
In contrast to the findings in the gut, A. muciniphila enhanced diet-induced expression of Tnfα and Reg3γ genes in the liver of mice fed a HF diet (). Il1β expression was significantly lower in AHF mice compared to LF, while both Tnfα and Reg3γ were increased in HF relative to LF, and AHF relative to HF (). This indicates a suppression of high fat diet-induced expression in the ileum but exacerbation in the liver.
High fat diet suppresses ileal immune signaling in the context of L. monocytogenes infection, while A. muciniphila increases expression of Ccl2
A suppression of immune signaling in the context of L. monocytogenes infection of mice fed a HF diet has been previously reported and may provide a mechanism for the increased susceptibility to infection in these mice.Citation3 Here, we similarly observed reduced expression of Il1β and Ccl2 in the ileum of the HF group compared to LF following Listeria infection. Interestingly A. muciniphila exposure increased expression of Ccl2 in the HF group to a level similar to LF ().
A. muciniphila does not promote significant alterations to the gut microbiota during short-term HF diet feeding
The gut microbiota provides a barrier between luminal pathogens and the intestinal epithelium and also modulates the intestinal immune response; effects which mediate colonization resistance against incoming pathogens.Citation21 We investigated whether the protective effects of A. muciniphila against L. monocytogenes infection may be mediated through broader effects upon microbiota community structure. There was no significant difference in alpha diversity between groups at day 13 (), while beta diversity of each diet was significantly different from each other diet at day 13 ().
Figure 3. The protective effect of A. muciniphila does not appear to be driven by changes in the gut microbiota.
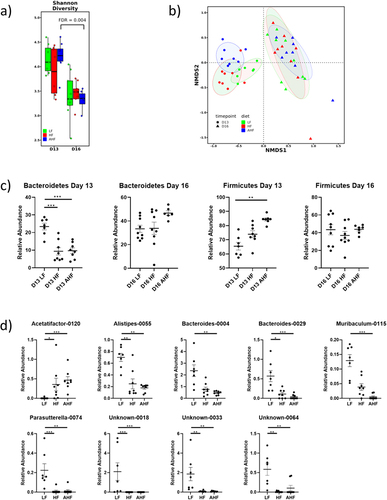
Animals fed a HF diet, with or without A. muciniphila, had decreased abundance of Bacteroidetes at day 13, while Firmicutes were increased in AHF mice relative to LF (). These changes are often associated with HF diets, low-grade inflammation, and a reduction in barrier function.Citation22 Our data demonstrated that administration of A. muciniphila did not significantly alter Bacteroidetes or Firmicutes levels in mice fed a HF diet.
Next, we compared the most abundant 200 taxa for each diet at day 13 and identified significant differences in the abundances of Alistipes, Acetatifactor, Bacteroides, Muribaculum, Parasutteralla, and several unidentified taxa (). For most taxa, the abundance in LF mice was significantly different to both HF and AHF with no difference between the high fat diets, suggesting that the changes were driven by diet rather than Akkermansia administration. In the cases of Bacteroides-0004 and Muribaculum-0115, abundance in AHF alone is significantly lower than in LF. However no significant difference exists between HF and AHF, suggesting that Akkermansia does not impact resistance to Listeria through overall changes in the composition of the microbiota.
Analysis of the microbiome suggested that although infection with L. monocytogenes decreased total bacterial diversity.Citation13, there were no significant differences in alpha diversity between treatments on day 16 (), and beta diversity shows no separation between groups ().
The abundances of Bacteroidetes and Firmicutes showed no differences between treatments during infection (). HF diet is usually associated with increased Firmicutes and decreased Bacteroidetes phyla, as we saw at day 13, which suggests that the concordance of phyla may reflect a homogenizing effect of Listeria infection. Similarly, at genus level, there were no significant differences at day 16 after correction for multiple comparisons (data not shown, ANOVA and KW-test followed by Tukey’s or FDR pairwise comparisons respectively, p > 0.05). Overall, we determined a minimal effect of A. muciniphila upon the microbiota community prior to infection with the treatment group (AHF) resembling the group fed HF diet alone. During infection we demonstrated a concordance of all mouse groups reflecting a potentially dominant impact of infection on microbiota community structure.
Changes in fecal and cecal metabolites are primarily driven by diet
To investigate the changes of microbial metabolites provoked by HF diet and A. muciniphila treatment before and after Listeria infection, we performed targeted analysis of short chain fatty acids in the cecal contents, and untargeted analysis of semi-polar metabolites in the feces, from six randomly selected mice per group on day 13. Principal component analysis (PCA) found no differences between the groups in the cecum or feces (). There were no significant differences detected among SCFAs in the cecum (Supplemental ). In the feces, there were no changes detected between HF and AHF mice (), suggesting that the protective effect of A. muciniphila administration is not mediated through these metabolites.
Figure 4. The protective effect of A. muciniphila does not appear to be driven by fecal or cecal metabolomes at day 13.
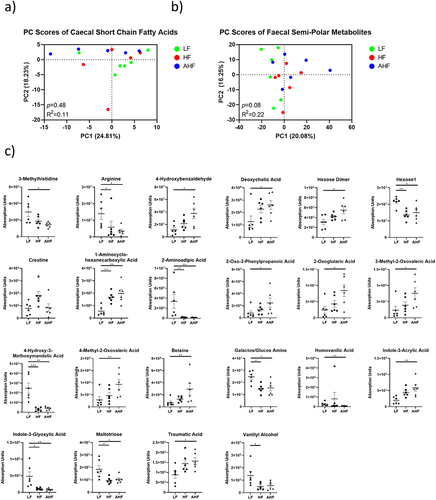
Discussion
A Westernized diet comprising a high caloric intake from fats and low consumption of fermentable fiber has been associated with diseases such as inflammatory bowel disease, obesity and type 2 diabetes.Citation1 A Westernized diet can also compromise intestinal barrier function and broadly influence immune signaling. Indeed, recent work by our group demonstrated an increased susceptibility to gastrointestinal and systemic infection by L. monocytogenes in mice fed a high fat (HF) diet over a relatively short (2 week) time period.Citation1,Citation3 We demonstrated significant alterations to intestinal histology and inflammatory status by short-term administration of a HF diet which were co-incident with predictable alterations to the microbiota (increased Firmicutes and decreased Verrucomicrobia).Citation3 However, it was unclear whether the effects on host immunity were mediated by diet or microbiota, or whether particular components of the microbiota could provide protection against infection in the appropriate context. Given that A. muciniphila is negatively impacted by HF diet and has been associated with positive effects on host barrier function, we examined the role of A. muciniphila upon host susceptibility to infection in the context of a HF diet.
A. muciniphila has been consistently associated with positive health benefits in models of metabolic disease, diabetes, and obesity.Citation23 Levels of A. muciniphila in the gut correlate negatively with bodyweight in humans, while Akkermansia supplementation in mice reversed HF diet-induced weight gain, hyperlipidemia, inflammation, and intestinal permeability.Citation18,Citation23 In our short-term (pre-obesity) model, HF diet increased expression of inflammatory markers but inflammation was reduced significantly by gavage with A. muciniphila. Previous studies have demonstrated the broadly anti-inflammatory effects of A. muciniphila intervention in long-term dietary models that reflect development of obesity or type 2 diabetes.Citation23,Citation24 Our current data suggest that such effects are demonstrable even after a shorter time period (pre-obesity) and correlate with an improvement in resistance to L. monocytogenes infection.
In contrast to the suppression of the inflammatory markers observed in the ileum, A. muciniphila enhanced inflammatory expression in the liver. Levels of Tnfα and Reg3γ expression were higher in the livers of HF mice compared to LF and were increased further in AHF mice. This was an unexpected finding which may reflect the short-term nature of our study, as A. muciniphila has been reported to decrease TNFα in a mouse model of liver injury,Citation25,Citation26 and reduced inflammation in a model of C. difficile infection.Citation27,Citation28 In our infectious model it is possible that the elevated expression of Tnfα in the liver can enhance systemic resistance to the pathogen. Indeed, it is interesting to note that these high levels of expression were maintained during Listeria infection (day 16) when a reduced Listeria load was observed (). Generally, the host response in the ileum during infection (day 16) was similar between mice fed a HF diet and those that received a HF diet plus A. muciniphila. This involved a suppression of immune signaling in mice fed a high fat diet during Listeria infection which has been previously reported and may contribute to the susceptibility to infection.Citation3 One exception to this was an increase in expression of Ccl2 in mice fed a HF diet plus Akkermansia compared to the HF group. CCL2-mediated stimulation of monocytes’ CCR2 chemokine receptor plays a vital role in the innate immune response to Listeria infection,Citation29 suggesting that modulation of innate immunity could contribute to Akkermansia-induced resistance to this pathogen.
To determine whether A. muciniphila administration affected microbiota community structure in our model we profiled the gut microbiota using 16S amplicon sequencing both before and during Listeria infection. Analysis demonstrated that HF diet increased the Firmicutes phylum and reduced the Bacteroidetes as noted in our previous short-term feeding studyCitation3 and in numerous longer term murine obesity studies.Citation30,Citation31 However, in our model A. muciniphila administration did not alter the Firmicutes or Bacteroidetes profiles over a two-week period. Similarly, whilst studies following longer-term administration of A. muciniphila have reported effects on specific bacterial interactions in the gut microbiota,Citation32 we failed to determine significant impact of A. muciniphila upon individual taxa. We suggest that greater impact of A. muciniphila upon the microbiota community structure in the context of a HF diet may be time-dependent. Surprisingly, there were no significant differences in the endogenous abundances of Akkermansia detected between LF and HF groups, yet infectious load was still increased by high fat diet. This suggests that the mechanisms by which HF diet increases susceptibility to Listeria infection involve more than the suppression of A. muciniphila, despite the protective effect provided by gavage with this species outlined in the current study.
In addition, extensive profiling of bacterial metabolites demonstrated that A. muciniphila administration did not significantly alter the metabolome relative to mice fed a HF diet alone. Overall, the findings suggest that the effects of A. muciniphila upon host immunity and anti-Listeria functions are independent of broader changes to microbiota community structure in the gut and are likely to be a direct consequence of the interaction between A. muciniphila and the host GI tract. Several studies have examined the molecular effectors of this interaction which is mediated through the A. muciniphila proteins AMUC_1100 and P9.Citation2,Citation33 Indeed, studies have demonstrated that pasteurized A. muciniphila or purified AMUC_1100 can exert similar effects on the host when compared to live bacterial cultures.Citation33,Citation34 Future work in our lab will examine the potential for pasteurized A. muciniphila or a protein extract to exert an anti-infective effect.
To our knowledge this represents the first report of the effects of A. muciniphila administration upon the outcome of a foodborne infectious pathogen. Our findings suggest a specific interaction between A. muciniphila and the host gut that modulates local inflammatory profiles and the gastrointestinal environment but does not depend upon alterations to the broader microbial community structure. The findings build upon previous work that has identified particular taxa that contribute to colonization resistance against L. monocytogenes infectionCitation35 toward the development of microbial interventions to prevent or reduce foodborne infection.
Materials and methods
Reagents
C57BL/6J mice (Envigo, UK); low fat chow (DIO series diets D12450H, Research Diets, Inc., USA); high fat chow (DIO series diets D12451, Research Diets, Inc., USA), GenElute Mammalian Total RNA kit (Sigma, USA), ReadyScript cDNA Synthesis Mix (Sigma, USA); KiCqStart SYBR Green qPCR ReadyMix (Sigma, USA); QIAamp Fast DNA Stool Kit (Qiagen, Germany); Custom oligo qPCR primers, (Eurofins Genomics, Germany). A. muciniphila was grown every day in 10 ml aliquots of anaerobic Mucin v3 media (10% inoculation) at 37°C. Nitrogen (and boiling) was used to remove the presence of oxygen from Mucin v3 media. Mucin v3 media contains peptone (Fluka), yeast extract (Roth), KH2PO4 (Fluka), NaCl (Fluka), (NH4)SO4 (Acros organics), MgSO4 (Acros organics), CaCl2 (Acros), NaHCO3 (Fluka), D-glucose (Fischer chemical), porcine mucin type II (Sigma), porcine hemin (Acros organics), L-cysteine (Sigma), and water.
Animals and study design
All the animal procedures were carried out in agreement with the guidelines of the European Commission for the handling of laboratory animals (directive 2010/63/EU), under authorizations issued by the Health Products Regulatory Authority (HPRA, Ireland) for the use of animals for scientific purposes and approved by the Animal Experimentation Ethics Committee of University College Cork.
Seven-week-old C57BL/6J mice were randomly assigned to low fat (LF) diet, high fat (HF) diet, or high fat diet plus A. muciniphila (AHF) treatments (n = 30 per group, ). Mice were housed in a specific pathogen-free facility on a 12-hour light/dark cycle at 22°C with access to water and either LF or HF chow ad libitum. A. muciniphila (1×109 CFUs in 200 μL) was delivered by daily intragastric gavage throughout the experiment. At day 13, n = 10 mice per group were sacrificed, while the remaining mice were treated with L. monocytogenes EGD-e InlAm in PBS either by intragastric gavage (5×109 CFUs, n = 10 per group) or intraperitoneal injection (5×105 CFUs, n = 10 per group), before sacrifice on day 16. Internal organs were homogenized and CFUs per organ measured on brain heart infusion (BHI) agar plates.
Quantitative real-time PCR
RNA was extracted from the distal ileum using the GenElute Mammalian Total RNA kit (Merck) as per manufacturer instructions and converted to cDNA using the ReadyScript cDNA Synthesis Mix (Sigma). qPCR was performed using KiCqStart SYBR Green qPCR ReadyMix (Sigma) in a LightCycler 480 for 40 PCR cycles using appropriate primers. Relative transcription was calculated using the 2-ΔΔCT method standardized to the average of the low fat group ΔCT. Primer sequences are presented in Supplemental Table 1.
Histological analysis
Tissue from the distal ileum was fixed in 4% paraformaldehyde overnight and dehydrated with 70% ethanol for 72 h at 4°C prior to paraffin embedding. 5 μm sections were mounted into slides and stained with hematoxylin and eosin using a standard procedure. Slides from six mice per group were blinded and inflammation was scored by an independent researcher (S.M.) using a protocol adapted from Drolia et al.Citation36 with some modifications.Citation3 Samples were scored on a scale of 0–3 for two parameters: infiltration of inflammatory cells (mostly mononuclear cells) to the villi and infiltration of mono- and polymorphonuclear cells to the crypt, yielding a maximum score of 6. In our model, polymorphonuclear cells were mainly located at the bottom of the crypts. The gradient of the inflammatory cell infiltration was based on 3 = highly increased, 2 = moderately increased, 1 = mildly increased and 0 = normal. Pictures were captured using an Olympus B×53 widefield microscope with an Olympus DP74 digital camera (Olympus GmbH, Germany). For goblet cell enumeration, slides from six mice per group were blinded, the lengths of all intact villi were measured and the number of goblet cells per villus was counted. This gave the number of goblet cells per millimeter. A minimum of 15 villi were measured per mouse, with a mean average of 34 villi included.
Fecal 16S rRNA gene sequencing
Fecal pellets were collected from each mouse on the day of sacrifice. DNA was extracted using the QIAamp Fast DNA Stool Kit as per the manufacturer’s instructions with the addition of a bead-beating step. The V3-V4 (341F, 805 R) variable region of the 16S rRNA gene was amplified according to the 16S metagenomic sequencing library protocol (Illumina, San Diego, CA, USA) and sequenced on an Illumina MiSeq. Raw sequences were profiled using FastQC/MultiQCCitation37,Citation38, adapter sequences removed using cutadapt (minimum length 200bp, maximum error rate of 0.2)Citation39, before optimizing filtering parameters in FIGAROCitation40, then applying optimized filtering and resolving amplicon sequence variants (ASVs) in R library DADA2 (0 mismatches, minimum overlap of 20bp, individual pools, consensus removal of bimeras).Citation41 Taxonomy was assigned to ASVs using DADA2’s assignSpecies function (100% sequence identity) with reference to the SILVA database (release 138Citation42), before removal of contaminant sequences using R library decontam.Citation43 All downstream analysis was performed in R version 3.4.3.Citation44 Alpha and beta diversities were calculated using the R package phyloseq. Differences in alpha diversity were assessed using the Mann-Whitney test. Differential abundant analysis was performed using DESeq2.Citation45 The adonis function in the vegan library was used to assess group-level differences in the microbiota.Citation46
Ultra-Performance Liquid Chromatography – Mass Spectrometry (UPLC-MS)
The fecal and cecal metabolome was measured at day 13 to investigate the production of microbial metabolites. Cecal content was examined for short chain fatty acids (SCFAs) and fecal samples were examined for semipolar metabolites, from six mice randomly selected from each treatment group.
Sample analysis was carried out by MS-Omics as follows. For SCFAs, samples were acidified using hydrochloride acid, and deuterium labeled internal standards where added. All samples were analyzed in a randomized order. Analysis was performed using a high polarity column (Zebron™ ZB-FFAP, GC Cap. Column 30 m x 0.25 mm x 0.25 µm) installed in a GC (7890B, Agilent) coupled with a quadrupole detector (5977B, Agilent). The system was controlled by ChemStation (Agilent). Raw data was converted to netCDF format using Chemstation (Agilent), before the data was imported and processed in Matlab R2014b (Mathworks, Inc.) using the PARADISe software described by Johnsen et. al.Citation47 For semi-polar metabolites, the analysis was carried out using a Thermo Scientific Vanquish LC coupled to Thermo Q Exactive HF MS. An electrospray ionization interface was used as ionization source. Analysis was performed in negative and positive ionization mode. The UPLC was performed using a slightly modified version of the protocol described by Catalin et al.Citation48 Peak areas were extracted using Compound Discoverer 3.1 (Thermo Scientific). Identification of compounds were performed at four levels; Level 1: identification by retention times (compared against in-house authentic standards), accurate mass (with an accepted deviation of 3ppm), and MS/MS spectra, Level 2a: identification by retention times (compared against in-house authentic standards), accurate mass (with an accepted deviation of 3ppm). Level 2b: identification by accurate mass (with an accepted deviation of 3ppm), and MS/MS spectra, Level 3: identification by accurate mass alone (with an accepted deviation of 3ppm).
Statistics
Statistics were performed in SPSS Version 28 (Chicago, IL, USA) and GraphPad version 9 (San Diego, CA, USA). Statistical significance was set to p < 0.05. Normality was determined by the Shapiro-Wilk test. Groups were compared with one-way ANOVA or Kruskal-Wallis (KW) test as appropriate, followed by Tukey’s or the two-stage step-up method of Benjamini, Krieger and Yekutieli pairwise comparisons respectively. Outliers were identified as values 2.2-times the interquartile range above or below the third or second quartile respectively. Permutational ANOVA (PERMANOVA) was used to compare β-diversity via Bray-Curtis dissimilarity, while principal component analysis was used to investigate UPLC data. Throughout, asterisks denote significance where * represents p < 0.05, ** p < 0.01, *** p < 0.001, and **** p < 0.0001.
Consent for publication
All authors consent to publication of this work and have had an opportunity to review the manuscript.
Ethics approval
All animal experiments were performed following ethical review by the University College Cork Animal Experimentation Ethics Committee (AEEC) and following project authorization by the Irish Health Products Regulatory Authority (HPRA) (project number AE19130/P115).
Acknowledgments
We acknowledge funding and support from Science Foundation Ireland in the form of a centre grant (APC Microbiome Ireland grant SFI/12/RC/2273_P2). The authors wish to acknowledge funding of JK from Science Foundation Ireland grant 16/IA/4445. This research was partly funded by the European Union’s Horizon 2020 Research and Innovation Program under the Marie Skłodowska-Curie grant agreement No. 641984, through funding of the List_MAPS consortium. We wish to thank Paul O’Toole, APC Microbiome Ireland, for help and expertise with A. muciniphila culture, Niall Hyland for general discussions and advice, Juliet Barry for support with H&E staining and Suzanne Crotty for support with acquisition of histology images.
Data availability statement
All data are available on Zenodo at the following link https://doi.org/10.5281/zenodo.7590374.
Disclosure statement
No potential conflict of interest was reported by the author(s).
Additional information
Funding
References
- Statovci D, Aguilera M, MacSharry J, Melgar S. The impact of western diet and nutrients on the microbiota and immune response at mucosal interfaces. Front Immunol. 2017;8:838. doi:10.3389/fimmu.2017.00838.
- Las Heras V, Melgar S, MacSharry J, Gahan CGM. The influence of the western diet on microbiota and gastrointestinal immunity. Annu Rev Food Sci Technol. 2022;13(1):489–14. doi:10.1146/annurev-food-052720-011032.
- Las Heras V, Clooney AG, Ryan FJ, Cabrera-Rubio R, Casey PG, Hueston CM, Pinheiro J, Rudkin JK, Melgar S, Cotter PD, et al. Short-term consumption of a high-fat diet increases host susceptibility to Listeria monocytogenes infection. Microbiome. 2019;7(1):7. doi:10.1186/s40168-019-0621-x.
- Wotzka SY, Kreuzer M, Maier L, Arnoldini M, Nguyen BD, Brachmann AO, Berthold DL, Zünd M, Hausmann A, Bakkeren E, et al. Escherichia coli limits Salmonella Typhimurium infections after diet shifts and fat-mediated microbiota perturbation in mice. Nature Microbiology. 2019;4(12):2164–2174. doi:10.1038/s41564-019-0568-5.
- Nikitas G, Deschamps C, Disson O, Niault T, Cossart P, Lecuit M. Transcytosis of Listeria monocytogenes across the intestinal barrier upon specific targeting of goblet cell accessible E-cadherin. J Exp Med. 2011;208(11):2263–2277. doi:10.1084/jem.20110560.
- Derrien M, Vaughan EE, Plugge CM, de Vos WM. Akkermansia muciniphila gen. nov., sp. nov., a human intestinal mucin-degrading bacterium. Int J Syst Evol Microbiol. 2004;54(5):1469–1476. doi:10.1099/ijs.0.02873-0.
- Ouwerkerk Janneke P, van der Ark Kees CH, Davids M, Claassens Nico J, Finestra Teresa R, de Vos Willem M, van der Ark KCH, de Vos WM, Belzer C. Adaptation of akkermansia muciniphila to the oxic-anoxic interface of the mucus layer. Appl Environ Microbiol. 2016;82(23):6983–6993. doi:10.1128/AEM.01641-16.
- Sonoyama K, Fujiwara R, Takemura N, Ogasawara T, Watanabe J, Ito H, Morita T. Response of gut microbiota to fasting and hibernation in syrian hamsters. Appl Environ Microb. 2009;75(20):6451–6456. doi:10.1128/AEM.00692-09.
- van Passel MW, Kant R, Zoetendal EG, Plugge CM, Derrien M, Malfatti SA, van Passel MWJ, Chain PSG, Woyke T, Palva A, et al. The genome of Akkermansia muciniphila, a dedicated intestinal mucin degrader, and its use in exploring intestinal metagenomes. PLoS One. 2011;6(3):e16876. doi:10.1371/journal.pone.0016876.
- Derrien M, van Baarlen P, Hooiveld G, Norin E, Muller M, de Vos W. Modulation of mucosal immune response, tolerance, and proliferation in mice colonized by the mucin-degrader akkermansia muciniphila. Front Microbiol. 2011;2. doi:10.3389/fmicb.2011.00166.
- Derrien M, van Passel MW, van de Bovenkamp JH, Schipper RG, de Vos WM, Dekker J, de Vos W. Mucin-bacterial interactions in the human oral cavity and digestive tract. Gut Microbes. 2010;1(4):254–268. doi:10.4161/gmic.1.4.12778.
- Belzer C, Chia LW, Aalvink S, Chamlagain B, Piironen V, Knol J, de Vos WM. Microbial metabolic networks at the mucus layer lead to diet-independent butyrate and vitamin B 12 production by intestinal symbionts. mBio. 2017;8(5). doi:10.1128/mBio.00770-17.
- Amiri P, Hosseini SA, Ghaffari S, Tutunchi H, Ghaffari S, Mosharkesh E, Asghari S, Roshanravan N. Role of butyrate, a gut microbiota derived metabolite, in cardiovascular diseases: a comprehensive narrative review. Front Pharmacol. 2022;12:12. doi:10.3389/fphar.2021.837509.
- Ottman N, Reunanen J, Meijerink M, Pietilä TE, Kainulainen V, Klievink J, Huuskonen L, Aalvink S, Skurnik M, Boeren S, et al. Pili-like proteins of Akkermansia muciniphila modulate host immune responses and gut barrier function. PLoS One. 2017;12(3):e0173004. doi:10.1371/journal.pone.0173004.
- Shin NR, Lee JC, Lee HY, Kim MS, Whon TW, Lee MS, Bae J-W. An increase in the Akkermansia spp. population induced by metformin treatment improves glucose homeostasis in diet-induced obese mice. Gut. 2014;63(5):727–735. doi:10.1136/gutjnl-2012-303839.
- Bodogai M, O’Connell J, Kim K, Kim Y, Moritoh K, Chen C, Gusev F, Vaughan K, Shulzhenko N, Mattison JA, et al. Commensal bacteria contribute to insulin resistance in aging by activating innate B1a cells. Sci Transl Med. 2018;10(467). doi:10.1126/scitranslmed.aat4271.
- Xu Y, Wang N, Tan H-Y, Li S, Zhang C, Feng Y. Function of Akkermansia muciniphila in obesity: interactions with lipid metabolism, immune response and gut systems. Front Microbiol. 2020;11:11. doi:10.3389/fmicb.2020.00219.
- Ashrafian F, Keshavarz Azizi Raftar S, Lari A, Shahryari A, Abdollahiyan S, Moradi HR, Masoumi M, Davari M, Khatami S, Omrani MD, et al. Extracellular vesicles and pasteurized cells derived from Akkermansia muciniphila protect against high-fat induced obesity in mice. Microb Cell Fact. 2021;20(1):219. doi:10.1186/s12934-021-01709-w.
- Schneeberger M, Everard A, Gómez-Valadés AG, Matamoros S, Ramírez S, Delzenne NM, Gomis R, Claret M, Cani PD. Akkermansia muciniphila inversely correlates with the onset of inflammation, altered adipose tissue metabolism and metabolic disorders during obesity in mice. Sci Rep. 2015;5(1):16643. doi:10.1038/srep16643.
- Png CW, Lindén SK, Gilshenan KS, Zoetendal EG, McSweeney CS, Sly LI, McGuckin MA, Florin THJ. Mucolytic bacteria with increased prevalence in IBD mucosa AugmentIn VitroUtilization of mucin by other bacteria. Official Am J Gastroentero. 2010;105(11):2420–2428. doi:10.1038/ajg.2010.281.
- Takiishi T, Fenero CIM, Câmara NOS. Intestinal barrier and gut microbiota: shaping our immune responses throughout life. Tissue Barriers. 2017;5(4):e1373208. doi:10.1080/21688370.2017.1373208.
- Magne F, Gotteland M, Gauthier L, Zazueta A, Pesoa S, Navarrete P, Balamurugan R. The firmicutes/bacteroidetes ratio: a relevant marker of gut dysbiosis in obese patients? Nutrients. 2020;12(5):1474. doi:10.3390/nu12051474.
- Xu Y, Wang N, Tan HY, Li S, Zhang C, Feng Y. Function of Akkermansia muciniphila in obesity: interactions with lipid metabolism, immune response and gut systems. Front Microbiol. 2020;11:219. doi:10.3389/fmicb.2020.00219.
- Everard A, Belzer C, Geurts L, Ouwerkerk JP, Druart C, Bindels LB, Guiot Y, Derrien M, Muccioli GG, Delzenne NM, et al. Cross-talk between Akkermansia muciniphila and intestinal epithelium controls diet-induced obesity. Proc Natl Acad Sci USA. 2013;110(22):9066–9071. doi:10.1073/pnas.1219451110.
- Wu W, Lv L, Shi D, Ye J, Fang D, Guo F, Li Y, He X, Li L. Protective effect of Akkermansia muciniphila against immune-mediated liver injury in a mouse model. Front Microbiol. 2017;8:1804. doi:10.3389/fmicb.2017.01804.
- Rao Y, Kuang Z, Li C, Guo S, Xu Y, Zhao D, Hu Y, Song B, Jiang Z, Ge Z, et al. Gut Akkermansia muciniphila ameliorates metabolic dysfunction-associated fatty liver disease by regulating the metabolism of L-aspartate via gut-liver axis. Gut Microbes. 2021;13(1):1–19. doi:10.1080/19490976.2021.1927633.
- Collado MC, Laitinen K, Salminen S, Isolauri E. Maternal weight and excessive weight gain during pregnancy modify the immunomodulatory potential of breast milk. Pediatr Res. 2012;72(1):77–85. doi:10.1038/pr.2012.42.
- Wu Z, Xu Q, Gu S, Chen Y, Lv L, Zheng B, Wang Q, Wang K, Wang S, Xia J, et al. Akkermansia muciniphila ameliorates clostridioides difficile infection in mice by modulating the intestinal microbiome and metabolites. Front Microbiol. 2022;13:841920. doi:10.3389/fmicb.2022.841920.
- Serbina NV, Shi C, Pamer EG. Monocyte-mediated immune defense against murine Listeria monocytogenes infection. Adv Immunol. 2012;113:119–134.
- Singh RP, Halaka DA, Hayouka Z, Tirosh O. High-fat diet induced alteration of mice microbiota and the functional ability to utilize fructooligosaccharide for ethanol production. Front Cell Infect Microbiol. 2020;10:376. doi:10.3389/fcimb.2020.00376.
- Hildebrandt MA, Hoffmann C, Sherrill-Mix SA, Keilbaugh SA, Hamady M, Chen YY, Knight R, Ahima RS, Bushman F, Wu GD, et al. High-fat diet determines the composition of the murine gut microbiome independently of obesity. Gastroenterology. 2009;137(5):1716-24.e1–2. doi:10.1053/j.gastro.2009.08.042.
- Hänninen A, Toivonen R, Pöysti S, Belzer C, Plovier H, Ouwerkerk JP, Emani R, Cani PD, De Vos WM. Akkermansia muciniphila induces gut microbiota remodelling and controls islet autoimmunity in NOD mice. Gut. 2018;67(8):1445–1453. doi:10.1136/gutjnl-2017-314508.
- Plovier H, Everard A, Druart C, Depommier C, Van Hul M, Geurts L, Chilloux J, Ottman N, Duparc T, Lichtenstein L, et al. A purified membrane protein from Akkermansia muciniphila or the pasteurized bacterium improves metabolism in obese and diabetic mice. Nat Med. 2017;23(1):107–113. doi:10.1038/nm.4236.
- Depommier C, Everard A, Druart C, Plovier H, Van Hul M, Vieira-Silva S, Falony G, Raes J, Maiter D, Delzenne NM, et al. Supplementation with Akkermansia muciniphila in overweight and obese human volunteers: a proof-of-concept exploratory study. Nat Med. 2019;25(7):1096–1103. doi:10.1038/s41591-019-0495-2.
- Becattini S, Littmann ER, Carter RA, Kim SG, Morjaria SM, Ling L, Gyaltshen Y, Fontana E, Taur Y, Leiner IM, et al. Commensal microbes provide first line defense against Listeria monocytogenes infection. J Exp Med. 2017;214(7):1973–1989. doi:10.1084/jem.20170495.
- Drolia R, Tenguria S, Durkes AC, Turner JR, Bhunia AK. Listeria adhesion protein induces intestinal epithelial barrier dysfunction for bacterial translocation. Cell Host & Microbe. 2018;23(4):470–84.e7. doi:10.1016/j.chom.2018.03.004.
- Andrews S, Krueger F, Segonds-Pichon A, Biggins L, Krueger C, Wingett S. FastQC: a quality control tool for high throughput sequence data. Cambridge, UK: Babraham Institute; 2010.
- Ewels P, Magnusson M, Lundin S, Käller M. MultiQC: summarize analysis results for multiple tools and samples in a single report. Bioinformatics. 2016;32(19):3047–3048. doi:10.1093/bioinformatics/btw354.
- Marcel M. Cutadapt removes adapter sequences from high-throughput sequencing reads, EMB net. EMBnet J. 2011;17(1):10–12. doi:10.14806/ej.17.1.200.
- Weinstein MM, Prem A, Jin M, Tang S, Bhasin JM. FIGARO: an efficient and objective tool for optimizing microbiome rRNA gene trimming parameters. bioRxiv. 2019;610394. doi:10.1101/610394.
- Callahan BJ, McMurdie PJ, Rosen MJ, Han AW, Johnson AJA, Holmes SP. DADA2: high-resolution sample inference from Illumina amplicon data. Nat Methods. 2016;13(7):581–583. doi:10.1038/nmeth.3869.
- Quast C, Pruesse E, Yilmaz P, Gerken J, Schweer T, Yarza P, Peplies J, Glöckner FO. The SILVA ribosomal RNA gene database project: improved data processing and web-based tools. Nucleic Acids Res. 2013;41(D1):D590–D6. doi:10.1093/nar/gks1219.
- Davis NM, Proctor DM, Holmes SP, Relman DA, Callahan BJ. Simple statistical identification and removal of contaminant sequences in marker-gene and metagenomics data. Microbiome. 2018;6(1):226. doi:10.1186/s40168-018-0605-2.
- McMurdie PJ, Holmes S, Watson M. Phyloseq: an R package for reproducible interactive analysis and graphics of microbiome census data. PLoS One. 2013;8(4):e61217. doi:10.1371/journal.pone.0061217.
- Love MI, Huber W, Anders S. Moderated estimation of fold change and dispersion for RNA-seq data with DESeq2. Genome Biol. 2014;15(12):550. doi:10.1186/s13059-014-0550-8.
- Oksanen J, Blanchet FG, Kindt R, Legendre P, Minchin P, O’hara R, Simpson GL, Solymos P, Stevens MH, Wagner H. Community ecology package. R Package Version. 2013;2:321–326.
- Johnsen LG, Skou PB, Khakimov B, Bro R. Gas chromatography - mass spectrometry data processing made easy. J Chromatogr A. 2017;1503:57–64. doi:10.1016/j.chroma.2017.04.052.
- Doneanu CE, Chen W, Mazzeo JR. Uplc/Ms monitoring of water-soluble vitamin bs in cell culture media in minutes. Waters Corporation Application Note. 2011;1–7. https://www.waters.com/webassets/cms/library/docs/720004042en.pdf