ABSTRACT
The human gut microbiota is a key contributor to host metabolism and physiology, thereby impacting in various ways on host health. This complex microbial community has developed many metabolic strategies to colonize, persist and survive in the gastrointestinal environment. In this regard, intracellular glycogen accumulation has been associated with important physiological functions in several bacterial species, including gut commensals. However, the role of glycogen storage in shaping the composition and functionality of the gut microbiota offers a novel perspective in gut microbiome research. Here, we review what is known about the enzymatic machinery and regulation of glycogen metabolism in selected enteric bacteria, while we also discuss its potential impact on colonization and adaptation to the gastrointestinal tract. Furthermore, we survey the presence of such glycogen biosynthesis pathways in gut metagenomic data to highlight the relevance of this metabolic trait in enhancing survival in the highly competitive and dynamic gut ecosystem.
Introduction
The human gastrointestinal tract (GIT) contains a vast, complex and dynamic microbial community, termed the gut microbiota.Citation1,Citation2 This microbial population acts as a key contributor to host metabolism and physiology, and for this reason impacts on host health.Citation3–5 For example, elements of the gut microbiota stimulate proliferation and differentiation of intestinal epithelial cells (IECs) assuring efficient nutrient absorption, thereby positively contributing to human metabolism.Citation6 Other beneficial metabolic functions of the gut microbiota include: (i) regulation of iron, copper, magnesium and manganese availability,Citation7,Citation8 (ii) the fermentative conversion of non-digestible food components such as dietary fibers into short chain fatty acids (SCFAs), that act as an energy source for IECs and elicit immune modulatory effects on Tregs and resident macrophages, (iii) the degradation of toxic compounds, and (iv) the production of vitamins (for example vitamin K and certain B vitamins).Citation9–12 Furthermore, the presence of commensal bacteria has been shown to provide a barrier to intestinal infections.Citation13,Citation14
Gut microbiota studies are currently highly topical in the fields of microbiology, biotechnology, immunology and clinical medicine, while they have also raised awareness of the importance of intestinal health among the general public. Numerous primary research publications and reviews have covered aspects of the gut microbiota and its potential role in supporting and promoting human health, especially during early life,Citation15 a period during which the microbiota clearly contributes to the development of the infant gut and its associated immune system.Citation16–20 The gut microbiota is also known to impact on the risk of contracting certain diseases that are typically associated with adult life, such as cardiometabolic disorders, inflammatory bowel diseases, neuropsychiatric diseases and cancer.Citation3 Therefore, study of the gut microbiota and its associated host benefits holds a lot of promise in terms of promoting human health and well-being.
The human colon is one of the most densely populated microbial habitats known.Citation21,Citation22 Bacteria make up the majority of living microbes present in the human GIT and by inference are believed to be the main contributors to the metabolic activities that take place in the gut.Citation23,Citation24 Bacterial gut inhabitants mainly belong to the phyla Bacteroidota, Bacillota, Pseudomonadota, Actynomicetota, Fusobacteriota and Verrucomicrobiota.Citation23,Citation25,Citation26 It is generally accepted that microbial colonization of the GIT commences at birth; the composition of this early human gut microbiota demonstrates remarkable dynamism that gradually develops complex metabolic functions.Citation27 In contrast, in adults the microbiota is more stable and resilient, though may fluctuate in response to environmental factors, while maintaining core taxa for many years.Citation28
Determining the forces that shape our gut microbiota is fundamental to our understanding of the functioning of these intestinal communities.Citation2 Central to this goal is gaining detailed information on the ecology of our gut microbiota and the mechanisms by which the individual components of this microbiota first establish themselves and then persist in their particular niche.Citation29 The intracellular accumulation of glucose-containing polymers, such as glycogen, is an energy storage that has been associated with important physiological functions in several bacterial species. However, its role in influencing the presence and functionality of (specific components of) the gut microbiota in its habitat has not yet been thoroughly addressed.
Here, we critically review recent insights into the enzymatic machinery that certain gut bacteria use to accumulate and subsequently degrade intracellular glycogen, and discuss how this metabolic ability may facilitate colonization of the gut environment. We furthermore perform an in silico survey for the presence of genes that encode glycogen biosynthesis-associated enzymes in gut metagenomic libraries to highlight the prevalence of this metabolic trait as a possible fitness marker in the highly competitive and dynamic gut niche.
Human gut microbiota and its metabolic adaptation to its natural environment
Commensal bacteria inhabiting the gut ecosystem are believed to require specific adaptations to this particular ecological nicheCitation23. The establishment of intricate and dynamic interactions between the human host and its inhabiting microbes is necessary for these gut commensals to survive within the GIT, obtain nutrients and reproduce, and is believed to be beneficial for both partnersCitation30. The GIT is rich in macromolecules that can serve as nutrients for these gut microorganisms and therefore represent a secure habitat at a constant and optimal temperature where microbes can establish and multiplyCitation31. Although many members of the gut microbiota cooperate, there is also intense competition for space and resources within the GIT.Citation30
It is generally accepted that host diet is one of the main drivers that shape gut microbiota composition.Citation17,Citation32,Citation33 Put in simple terms, available nutrients determine whether or not a microorganism is able to establish and persist in the gut.Citation29,Citation34 Carbohydrate fermentation is a core activity of the human gut microbiota, as carbohydrates represent a major energy and carbon source in the gut.Citation35 The mammalian GIT has different compartments with diverse nutrient and environmental conditions and, consequently, different microbial communities. The small intestine houses microorganisms that utilize simple sugars, while in the colon, the bacterial inhabitants are more specialized toward complex dietary fiber fermentationCitation29 and also host glycans such as mucin-associated carbohydrates.Citation36 Accordingly, a plant-based, polysaccharide-rich diet favors expansion of those organisms that can metabolize such dietary fibers either directly or through cross-feeding activitiesCitation37. Bacterial glycan fermentation generally produces beneficial metabolites in the form of SCFAsCitation9. Acetate, propionate, and butyrate are the three main SCFAs, and each of these play different roles in human physiology.Citation9,Citation38–40
Metabolic adaptation describes the ability to metabolically switch from one substrate to another, a property that is essential for the fitness and survival of microorganisms in competitive environments such as the GIT.Citation41,Citation42 In the mammalian gut environment, nutrient availability is constantly fluctuating and saccharidic substrates that support bacterial growth are not continually available and if so, they are typically present in limiting amounts.Citation43 The model bacterium Escherichia coli, as an example of a gut commensal, exhibits metabolic adaptation through its ability to use a wide range of dietary sugars in order to ensure durable gut colonization.Citation42–44 Similarly, gut bacteria belonging to the genera Bacteroides, Bifidobacterium and Ruminococcus are capable of degrading complex carbohydrates.Citation45,Citation46 For example, in a complex nutrient environment, such as that resulting from a high fiber diet, human gut-associated Bacteroides species can switch their metabolism to suit available glycan substrates. However, a microorganism can still persist in the gut if it is able to utilize some of the available nutrients more efficiently than its competitors.Citation29
In order to cope with life in a highly competitive nutrient-limiting or nutrient-fluctuating environment, certain bacteria, including gut commensals, have developed the ability to create intracellular energy stores, such as glycogen. This polymer is accumulated under certain conditions thanks to a dedicated enzymatic machinery to serve as a major energy reserve in bacteria that may underpin successful gut colonization and survival.Citation43,Citation47,Citation48
Box 1: Colonization factors in gut commensal bacteria
To bestow beneficial activities on a human host through host-microbe mutualistic interactions, symbionts and probiotic commensals need to establish themselves, at least temporarily, in the gut environment.Citation49 Thus, investigation of the mechanisms that enable these microbes to colonize, stay alive and be metabolically active in the GIT is of key importance in order to fully understand their functions.Citation50 Apart from the ability of gut bacteria to metabolize dietary or host-derived compounds, other colonization factors have been described (). These include mechanisms such as amino acid decarboxylases or the F0F1-ATPase, which allow bacteria to tolerate fluctuating and low pH (ranging from 6.5 to 7.5 in the mouth to 1.5–6 in the stomach, and from 5.7 to 7.4 in the small intestine, cecum and colon).Citation51,Citation52 In the small intestine, bacteria need to survive exposure to toxic bile, a fluid which is predominantly composed of primary and conjugated bile acids (BAs), and which acts as a potent detergent, essential for fat digestion and absorption.Citation53 Many gut bacterial inhabitants use hydrolytic enzymes to protect themselves from BA toxicity.Citation54–58 Production of surface exopolysaccharides (EPS), biofilms and efflux pumps have also been associated with providing bile tolerance in some bacterial species.Citation59–62
Figure 1. Colonization Factors in gut commensal bacteria.
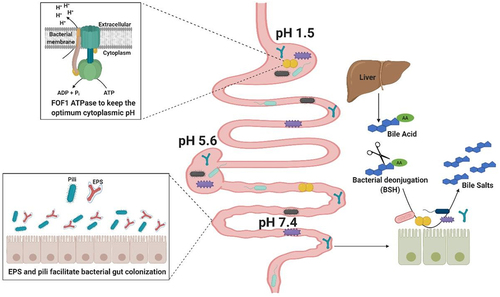
Adhesion to intestinal epithelial cells, mucus, or components of the extracellular matrix represents an additional important bacterial colonization factor.Citation63–66 Adhesion to the intestinal epithelium is considered crucial for commensal bacteria to facilitate gut establishment, thereby competing with and thus limiting colonization by intestinal pathogens.Citation67 EPS appears to play an important role in bacterial interaction with the intestinal mucosa, although the precise mechanism of this interplay is still poorly understood.Citation68 The presence of EPS surrounding the bacterial surface can reduce adherence to intestinal cells thereby negatively impacting on intestinal colonization.Citation69–72 whereas others have demonstrated that EPS-producing bacteria are better at tolerating the gastrointestinal transit and can persist longer in the GIT.Citation59,Citation73 In addition to EPS, other extracellular structures, such as pili are implicated in adhesion processes.Citation74,Citation75 For example, sortase-mediated and Tad (tight adherence) pili have been identified in several species of commensal bacteria, including lactobacilli and bifidobacteria, and shown to be important for their adherence, gut colonization and host cell proliferation.Citation76–82
Glycogen storage by members of the gut microbiota
Energy storage in the form of branched glucose polymers is clearly an ancient ability and may have been present in the last universal common ancestor of all present life.Citation83 Glycogen and other related polyglucose compounds share a common structure composed of glucose monomers that are connected by α-1,4- and α-1,6-linkages, and are among the preferred carbon sources of many gut commensals. Various bacteria in the GIT environment possess the enzymatic machinery to utilize these dietary polysaccharides as an extracellular carbon and energy source, as has been addressed in several reviews.Citation84,Citation85 Certain bacteria, employing products of the degradation of these polysaccharides, or other carbohydrates, are capable of synthesizing de novo glycogen-like polymers intracellularly (glycogen, starch or maltodextrin).Citation86 However, relatively little attention has been paid to the physiological role of these intracellular energy storage polymers, generally referred to as glycogen, or the molecular pathways and the specific enzymes required for intracellular biosynthesis and subsequent degradation. Herein, we discuss intracellular glycogen metabolism under the terms glycogen accumulation/degradation to describe such processes, unless otherwise indicated.
Bacterially produced glycogen is a water-soluble polymer consisting of chains of α-1,4-linked glucose units that contain regular α-1,6-linked branchesCitation87 with an estimated average molecular weight between 107 and 108 Daltons.Citation48,Citation88 Glycogen branching is important for fast response to metabolic needs, because both synthesis as well as degradation of the glycogen polymer occur from the non-reducing ends of the α-1,4 chains. Thus, highly branched glycogen has a higher number of “ends” per volume, while branching also increases glycogen solubility.Citation83 Due to its large molecular mass, high solubility and highly branched structure, glycogen represents an efficient form of energy and carbon storage with little effect on the internal osmolarity of cells.Citation86,Citation88
Several early studies have described accumulation of glycogen in bacteria. This characteristic was thoroughly studied in E. coli.Citation89,Citation90 Using spectrophotometry, Levine and colleagues discovered that under certain cultivation conditions a characteristic set of absorption bands which appeared in the infrared spectrum of this enteric bacterium, were due to a glycogen-like polysaccharide.Citation91 These authors also showed marked variability in intracellular glycogen levels among different enteric strains and upon growth on different carbon sources,Citation91 with E. coli even accumulating intracellular glycogen granules.Citation89 A study carried out in Enterobacter aerogenes (previously Aerobacter aerogenes) demonstrated that utilization of degradation products of intracellular storage polymers supports bacterial survival under certain challenging conditions in vitro.Citation92 Another classic publication provides further information on the role of glycogen as a bacterial energy and carbon reserve, though being dependent on the bacterial species and nature of the carbon and energy source in the environment.Citation93 In Bacteroides fragilis, the intracellular polysaccharide was shown to be degraded rapidly when glucose was in short supply and fatty acids accumulated in the medium.Citation94 Thus, the concept of intracellular polysaccharide accumulation in (gut-associated) bacteria was established in the early 60s.Citation95,Citation96
At that time, findings suggested that certain microorganisms, e.g. Pseudomonas aeruginosa, do not synthesize any specific reserve compound.Citation97 More recently, it has been conclusively established that the ability to produce and accumulate intracellular α-glucan polysaccharides such as glycogen represents a feature of many, but not all bacteria.Citation98 Nonetheless, intracellular glycogen accumulation has been observed in more than 50 bacterial species, including Gram-positive and Gram-negative bacteria, as well as archaebacteria.Citation48,Citation87 Indeed, glycogen storage is ubiquitous among enteric bacteria, such as En. aerogenes, E. coli, Lactobacillus acidophilus, Edwardsiella tarda, Hafnia alvei, several species of Bacillus, and Streptococcus, and various ruminal bacteria (Preiss 2006) (). Furthermore, some of these glycogen-accumulating bacteria are gut pathogens, such as Clostridium botulinum, several species of Salmonella and Vibrio cholerae. As we will discuss below, glycogen accumulation in both pathogenic and nonpathogenic bacteria is associated with increased gut survival and tolerance to gut-associated stress conditions.
Table 1. Intracellular accumulation of glycogen reported in gut-related bacteria.
Biosynthesis and degradation of glycogen
Glycogen metabolism allows microorganisms to employ glucose polymers as a dedicated carbon and energy storage facility that can be mobilized for future purposes. This metabolic property relies on an enzymatic machinery responsible for glycogen biosynthesis and subsequent degradation, along with regulatory mechanisms in order to store or recruit glucose moieties depending on cellular needs.
In E. coli, Bacillus megaterium and other bacteria, glycogen is synthesized during times when carbon is abundant while other nutrients (e.g. nitrogen or phosphate) are limiting.Citation122,Citation123 However, in Fibrobacter succinogenes glycogen synthesis may take place during any growth phase.Citation111 Glycogen accumulation in L. acidophilus is carbon source dependent.Citation112 In contrast, glycogen degradation occurs when carbon sources become limiting.Citation43,Citation124 Under such circumstances, release of glucose units from glycogen by specific enzymes provide carbon resources to central metabolism.Citation125 Therefore, analysis of the enzymatic functions involved in intracellular glycogen synthesis/degradation and their mechanisms of regulation are important to understand how and when microbes implement this apparent survival strategy.
Most of the enzymes involved in glycogen metabolism in bacteria belong to glycosyl transferase or glycosyl hydrolase families, that contain specific carbohydrate-binding modulesCitation87,Citation88,Citation126 (). The classical glycogen biosynthetic pathway in bacteria, such as E. coli and L. acidophilus, involves phosphoglucomutase (Pgm), glucose-1-phosphate adenylyl transferase (GlgC), ADP-glucose-specific glycogen synthase (GlgA) and branching enzyme (GlgB). The enzymes GlgP (glycogen phosphorylase) and GlgX (debranching enzyme) are implicated in glycogen degradation ( and ).
Figure 2. Glycogen metabolism pathways in gut commensal bacteria.
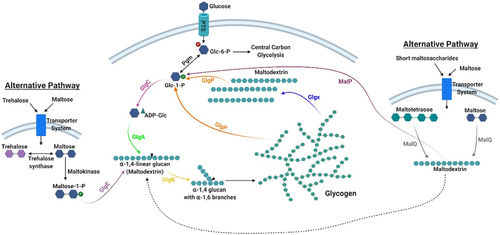
Table 2. Biosynthetic and degradative enzymes in the metabolism of glycogen.
Studies conducted on the gut bacterium E. coli were pivotal to elucidate the glycogen metabolic pathway that was described in detail by Preiss.Citation122,Citation127 Extracellular glucose is taken up and converted to glucose-6-phosphate by a bacterial phosphoenol-phosphotransferase system (PEP-PTS). Alternative sugars that can be converted to glucose-6-phosphate may also be used. Pgm converts glucose-6-phosphate into glucose-1-phosphate, which serves as a substrate for ADP-glucose synthesis catalyzed by GlgC.Citation127 GlgA catalyzes the transfer of glucosyl units from ADP-glucose to the elongating chain of linear α-1,4-glucan with the concomitant release of ADP. GlgB subsequently cleaves off portions of this α-1,4-glucan and links it to internal glucose molecules in existing chains via α-1,6 glycosidic bonds to form the glycogen branching structure.Citation86 Some bacteria possess a regulatory subunit of GlgC, called GlgD,Citation112 where GlgC itself acts as the catalytic subunit. Glycogen catabolism is mediated by glycogen phosphorylase (GlgP) which removes glucose units from the non-reducing ends of the glycogen/maltodextrin molecules liberating glucose 1-phosphate.Citation128 At the same time an isoamylase-type debranching enzyme (GlgX) cleaves the α-1,6-bonds of the limit dextrins generated by GlgP (typically 3–5 glucosyl residues in length) releasing maltodextrinsCitation86,Citation129 that can be further metabolized by GlgP (). The glucose-1-phosphate produced can be transformed into glucose-6-phosphate by Pgm and shuttled into the central carbon metabolic pathways, e.g. glycolysis or bifid shunt, the latter defined as the central and unique metabolic pathway for carbohydrate fermentation employed by bifidobacteria.Citation130 This model represents the prevalent bacterial pathway of intracellular glycogen synthesis and degradation.Citation98
It has been reported that certain microorganisms, such as species belonging to the genus Streptomyces, possess an alternative route for intracellular α-glucan biosynthesis, called the GlgE pathway. This alternative pathway employs trehalose or maltose as precursors for the disaccharide α-maltose 1-phosphate. A dedicated maltosyltransferase (GlgE) uses the latter molecule as the building block to elongate glucan chainsCitation131,Citation132 (). Furthermore, it has been described that maltose metabolic enzymes play a role in glycogen synthesis and degradation in E. coli.Citation133 Briefly, the action of an amylomaltase (MalQ) on maltose or maltooligosaccharides can lead to the formation of maltodextrinsCitation133 and a maltodextrin phosphorylase (MalP) releases glucose 1-phosphate from glycogen moleculesCitation90 (). Generally, the glycogen metabolism genes are organized in a single gene cluster in most bacteria known to accumulate glycogen (see section below).
Presence and distribution of glycogen metabolism genes in human gut commensals
Genomics approaches have been crucial to reveal the complex interactions between a host and its resident bacteria and to unravel gut colonization strategies and microbiota functionalityCitation46. A previous survey of 55 bacterial genomes from different environments, such as plant, animals and humans, revealed that about half of these bacterial species contain an apparently full set of glycogen metabolic genes.Citation125,Citation126 This finding was later substantiated by another study of 1202 deduced bacterial proteomes.Citation134 Based on recent genomic analyses, intact glycogen metabolic pathways are present in bacterial species adaptable to diverse environments and possessing flexible lifestyles.Citation98,Citation134 In contrast, a significant proportion of bacteria with parasitic behavior lack this glycogen biosynthetic ability.Citation126 Taking advantage of the increasing number of available gut metagenomic datasets, we first examined the abundance of the glycogen metabolism pathway among selected members of the human gut community, using an existing computational methodCitation135 across 1,267 gut metagenomes from American, European, and Chinese subjects. We found evidence for the presence of this pathway in all metagenomes surveyed (minimum abundance = 1 copy/1000 cells), suggesting that this appears to be a core activity of the human gut microbiota (unpublished data).
We then analyzed the occurrence of putative glycogen gene products in 70 representatives of the different genera using the Catalog of Reference Genomes From the Human (gut) MicrobiomeCitation136. We employed BLASTP analysis (cutoff values: E-value <0.00001, at least 20% identity across at least 50% of sequence length) and functional annotation profiling efforts to establish the occurrence of specific glycogen biosynthetic and degradative enzymes among gut bacteria (as outlined in ). Interestingly, this in silico analysis revealed that the majority of assessed members (~80%) of the gut microbiome, including components of the major bacterial phylogenetic divisions of the human gut microbiota, are predicted to harbor the metabolic genes involved in intracellular glycogen synthesis/breakdown, suggesting that this trait is a core function of such microbes from the gut environment. This analysis also revealed that the orthologous proteins are variably distributed across species, where certain enzymes seem to be more specific while others are present among all members. Therefore, there are organisms completely lacking glg homologs, organisms harboring partial sets of such homologs, and organisms harboring all known glg gene homologs, while some members of the latter two groups harbor more than one copy of a particular glg gene homolog.Citation98
Remarkably, all members of Bacteriodetes lack GlgC though it may be that they use other proteins such as MalQ or UDP-glucose adenylyltransferase to generate the glycogen polysaccharide. Alternatively, Bacteroidetes members may not encode an E. coli-type GlgC enzyme, yet possess an enzyme that is more similar to human glycogen enzymes as has been described for other bacteria.Citation83 Indeed, the glycogen synthesis pathway is functional in Bacteroides fragilis since accumulation of an intracellular polysaccharide formed from glucose has been demonstrated in 27 Bacteroides fragilis strains.Citation94 shows that GlgE is not common in the adult human gut microbiome since only a relatively small number of species, for example members of the genus Bifidobacterium, encode this enzyme. Transcriptome analysis has revealed that the expression of genes predicted to encode proteins participating in glycogen metabolism are up-regulated under particular circumstances in E. coli and L. acidophilus.Citation88,Citation112 Nonetheless, bifidobacterial species that are not associated with the mammalian GIT lack genes encoding glycogen metabolism.Citation137 Certain bacterial species that have been found in fecal samples, for example Pediococcus acidilactici, Weissella paramesenteroides, Gordonibacter pamelaeae, Dialister succinatiphilus, and Leuconostoc mesenteroides subsp. cremoris, among others, do not encode Glg proteins and thus do not appear to be able to synthesize intracellular glycogen. Some of these species may in fact have a food origin and therefore may not belong to the autochthonous gut microbiota population.Citation138 The glg genes also appear to be absent from the genome of Streptococcus infantarius subsp. infantarius ATCC BAA-102, which is associated with colorectal cancer, or the pathogenic strains Helicobacter pylori 83 and Enterococcus faecalis EnGen0107 (). As mentioned above, certain bacterial species contain more than one homologue of a particular glg gene, particularly in the case of glgB and glgP. Most notable examples are Blautia obeum ATCC 29,174 which possesses two homologues for glgC, glgD, glgP and glgX, and three glgB homologues, or Dorea longicatena DSM 13814 which contains two homologues for each of the glgC, glgD, glgB and glgP genes. In these species with several copies of a given glg gene, some of the copies represent homologs from different species (rather than being paralogs; data not shown). This peculiarity has been noticed previously in a study of glycogen metabolism genes in gammaproteobacterial species and may be a genetic adaptation to increase intracellular glycogen production/degradation rates or represent glycogen production under distinct circumstances by a specific set of glg genes.Citation98
Figure 3. Presence/Absence of predicted glycogen biosynthetic and degradative enzymes in gut bacteria.
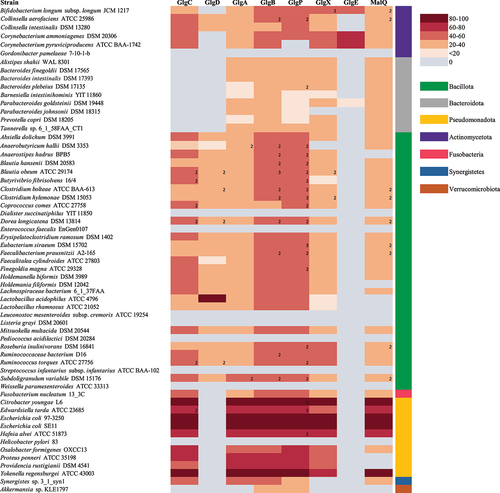
Genetic organization of glycogen genes
Bacterial genes associated with the same metabolic pathway are often clustered.Citation98,Citation139 In E. coli, this cluster includes the glgC, glgA and glgB genes, plus two genes involved in glycogen degradation, glgX and glgP.Citation127,Citation140 The gene arrangement of these glg genes is glgBXCAP which is also conserved in all species harboring glg genes in the orders Enterobacteriales and Pasteurellales.Citation98 Clustering of genes involved in glycogen metabolism has also been described for other bacteria, e.g. Bacillus stearothermophilus and B. subtilis, Lactiplantibacillus plantarum and Vibrio spp. among others.Citation86,Citation98,Citation103,Citation141 In the probiotic bacterium L. acidophilus the glycogen metabolic pathway is encoded by a 11.7-kb chromosomal region consisting of glgBCDAP-amy-pgm, while glg operons in other lactobacilli have the apparently conserved gene arrangement glgBCDAPCitation86,Citation112 (). Notably, in the gut commensals surveyed in this review there are exceptions to this single glg gene cluster. For example in Holdemanella filiformis and Erysipelatoclostridium ramosum these genes are organized in two clusters, glgCDA and glgBPX. Curiously, in the species Bacteroides intestinalis, Bacteroides finegoldii, Citrobacter youngae, Parabacteroides goldsteinii and Bifidobacterium longum subsp. longum, among others, the glg genes are scattered across the chromosome ().
Figure 4. Genetic organization of glycogen genes in gut commensal bacteria.
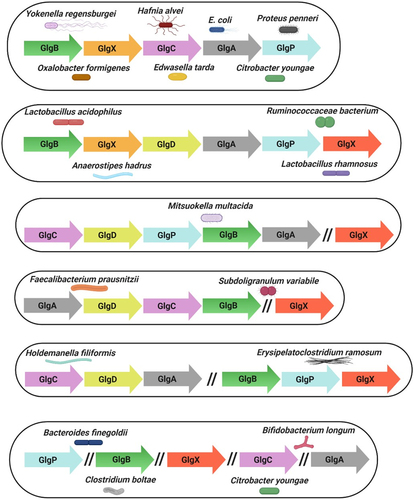
Thus, the presence of complete glycogen pathways, and their tight regulation, in human gut commensals is likely reflecting the importance of this metabolism in the gut ecosystem (see Box 2 and next section).
Box 2: Regulation of glycogen metabolism
Glycogen metabolism is closely interconnected with various metabolic processes as reviewed by Wilson and colleagues.Citation88 Thus, not surprisingly, it is subject to complex transcriptional, post-transcriptional and allosteric regulation as described below. The reader should be aware that most of the findings related to regulatory mechanisms of glycogen metabolism were generated in E. coli and will be covered as such in the following paragraphs unless otherwise indicated.
At a transcriptional level, glg genes are generally co-ordinately expressed within various regulons, and their expression is triggered by signals related to nutrient starvation. Carbon catabolite repression (CCR) is a transcriptional regulatory mechanism aimed at ensuring sequential utilization of more preferred carbon sources through repression of genes required for the metabolism of less preferred carbon compounds.Citation142 This repression has been associated with activation of glycogen metabolism upon glucose shortage in several organismsCitation142 including E. coli, Salmonella spp., Streptococcus suis, Corynebacterium glutamicum, Mycobacterium spp., or V. cholerae.Citation47 So-called catabolite responsive elements (cre) are located upstream glg genes in various organisms, which indicates CCR-mediated regulation.Citation143 Glucose and other nutrient depletion signals also trigger the stringent response, which re-programmes metabolic processes allowing bacteria to adapt and survive adverse conditions.Citation144 In E. coli the stringent and general stress responses are major determinants of intracellular glycogen content.Citation145 Under a range of stress factors and nutrient limitations, the alarmone ppGpp, a small nucleotide that acts as a global transcriptional regulator in bacteria, is a well-established positive regulator of the glycogen biosynthetic genes in E. coli.Citation146–148 However, glycogen accumulation is independent of (p)ppGpp or cre elements in various organisms, suggesting that these genes are subject to an alternative control mechanism.Citation145 Alternative sigma factors have been implicated in the transcriptional control of glg genes in E. coli, Bacillus subtilis and C. difficile.Citation103,Citation149,Citation150
At post-transcriptional level, glycogen storage is negatively regulated by the carbon storage regulator CsrA, a small-RNA binding protein that inhibits expression of targeted genesCitation151,Citation152. CsrA controls a wide variety of cellular processes including some that affect interactions of enteric species with mammalian hosts, such as central carbon metabolism, biofilm formation, virulence and motility, among othersCitation42,Citation153,Citation154. At the enzymatic level, allosteric mechanisms of regulation have been described for GlgC and GlgP, which catalyze key steps in the biosynthesis and catabolism of intracellular glycogen, respectively. Specifically, these enzymes are generally activated by metabolites that reflect signals of high carbon and energy content such as phosphoenolpyruvate, glucose-6-phosphate, fructose-1,6-bisphosphate or pyruvate, whereas they are inhibited by metabolites reminiscent of low metabolic energy levels like AMP, ADP and/or PiCitation141,Citation145,Citation155–159. While different levels of allosteric regulation for these enzymes have been described in various organismsCitation141,Citation157, allosteric regulation of GlgC is considered a main control point of glycogen accumulation in early stationary phase cellsCitation160.
Roles of glycogen storage in gut bacteria
Environmental stresses in the GIT impose physiological challenges upon bacteriaCitation161 which must adapt in response to such adverse conditionsCitation29 (see Box 1). In this regard, glycogen accumulation is a common feature across phylogenetically divergent species, and appears to represent a bacterial adaptation to nutrient (or other) limitation in the gut.
What follows below is an in-depth analysis of available evidence, obtained from in vitro studies simulating the gut environment and in vivo studies employing animal models. All of which point toward glycogen metabolism as a widespread and fitness-enhancing trait among gut commensals.
The role of glycogen accumulation in simulated gut conditions
Glycogen is a stored energy resource linked to important physiological functions in bacteria as discussed below. Transient and resident gut commensals, including those administered as adjunct cultures in fermented foods or those provided as probiotic supplements, must cope with a range of challenges during their transit along the GIT that serially enforce strong selective pressures hampering bacterial survival and establishment in the lower gut ecosystem.Citation162,Citation163 Glycogen accumulation has been linked to (long-term) survival where it can offer an energy source when the gut environment cannot.Citation88 However, the role of glycogen metabolism as a specific strategy for gut commensals to survive and thrive in their natural habitat has not received much scientific attention. Next, laboratory in vitro experiments simulating various stresses are shown to provide valuable insights to understand processes that occur in the mammalian gutCitation164.
Glycogen storage and survival upon macro- and micro-nutrient starvation
Nutrient limitation will for obvious reasons affect the gut microbiota. Nutrient availability in the GIT is affected by circadian oscillations, imposed by periods of fasting and feeding,Citation29,Citation43,Citation165,Citation166 and is in general characterized by the presence/absence of non-digestible complex carbohydrates as carbon sources.Citation167 Under such circumstances, metabolic flexibility and mixed-substrate utilization offer selective strategies for efficient gut colonization and survival.Citation29,Citation168 These circumstances include: (i) availability of complex and non-digestible carbon sources, (ii) ability or lack thereof to utilize metabolites or sub-products released by other neighboring gut microbial inhabitants, and (iii) an ability to efficiently adapt metabolic activity to available nutrients where and when appropriate.
Several in vitro studies have investigated if the ability to accumulate glycogen affects survival of gut commensals under carbon starvation. For example, it was demonstrated that En. aerogenes cells containing glycogen survived better in an unfavorable environment in which carbon was limited.Citation169 In L. acidophilus, cells showed stable intracellular glycogen reserves during prolonged growth periods and for this reason it has been speculated that L. acidophilus maintains a higher level of intracellular glycogen to enhance its residence time when growing under conditions simulating the GIT environment.Citation112 Mutations that disrupt glycogen metabolism were also shown to negatively impact on E. coli fitness during nutrient starvation, demonstrating its role in maintenance of viability and alternative energy source acquisition during metabolic transitions.Citation42
These publications suggest that under carbon deprivation, glycogen is utilized to preserve cell viability, providing the energy and carbon source required by the bacteria for maintenance.Citation48 This bacterial durability has been linked with glycogen average chain length: glycogen with an average short chain length correlated with prolonged bacterial survival.Citation134 This effect was attributed to the slower degradation of this short chain length polymer, which can sustain bacterial viability for prolonged periods of time.Citation125
Regarding nutrient competition, the presence of specific alternative carbon sources to glucose, such as raffinose and the disaccharides trehalose and lactose, has been described to act as a signal promoting intracellular glycogen accumulation in L. acidophilus.Citation86 Raffinose is a non-digestible carbon source, known to be specifically fermented at intestinal level by several commensal species including representative strains of lactobacilli and bifidobacteria.Citation170 Specifically, growth on raffinose was associated with temporally increased expression of glg genes, which was shown to coincide with glycogen accumulation. Furthermore, glycogen accumulation was required to support L. acidophilus growth on raffinose as ΔglgA and ΔglgB mutants were shown to exhibit impaired growth on this carbohydrate.Citation86 These results suggest a close relationship between the metabolism of certain carbohydrates and glycogen metabolism, and although the specific molecular connections are currently not understood, both pathways are likely to be under the control of common central carbon regulators (see section about regulation in Box 2). Indeed, evidence in lactobacilli suggests that glg expression and glycogen accumulation are repressed by glucose as in other enteric bacteria, thus its expression might be enhanced in the presence of other carbon sources such as the ones typically encountered within the gut ecosystem. Thus, glycogen synthesis seems to be triggered by certain environmental conditions despite high energy and carbon availability, while glycogen degradation occurs during extended nutrient starvation.Citation171
The limitation of micronutrients in the gut may also affect intracellular glycogen metabolism in bacteria. For example, the bacterial response to a shortage of phosphate in the gut, which can occur under physiological stress conditions of the host, includes activation of virulence in a broad range of pathogens, as well as of glycogen accumulation.Citation172 Nitrogen is another limiting nutrient for gut microbial communities, that strongly determines gut microbial composition and their interactions with the host.Citation173,Citation174 For example, E. coli MG1655 cells grown under nitrogen-limiting conditions were shown to exhibit the highest level of glycogen accumulation compared with carbohydrate-limited conditions during stationary phase.Citation108
Remarkably, a shortage of nitrogen has been demonstrated to activate the stringent response, evidenced by increased glycogen accumulation in E. coli and Vibrio cholerae cells grown in the presence of glucose excess.Citation121,Citation175 In E. coli, limited availability of iron also connects the stringent response to glycogen metabolism as it promotes ppGpp accumulation which overall results in promotion of glycogen biosynthesis.Citation176 Accordingly, mutants defective in sensing and sequestering iron display altered glycogen accumulation phenotypes.Citation145 Consistent with this observation, it was found that the glgX gene of the commensal Bifidobacterium breve UCC2003 is important for survival under iron-limiting conditions.Citation177
Thus, glycogen accumulation as a means of intracellular carbon and energy storage may represent a common survival mechanism for commensals across different compartments of the GIT and an advantage in an environment with strong competition for available nutrients.
Glycogen storage and Survival upon acid and bile stress conditions
It is increasingly clear that many stress responses overlap and thus a stress response to one specific factor commonly results in cross-resistance to other, apparently unrelated stressors.Citation178 In this regard, it is conceivable that glycogen accumulation, when activated in response to decreased availability of nutrients and/or micronutrients, plays a role in the response and tolerance to other stress factors encountered in the GIT. As an example of this interconnection, nutrient starvation, which generally marks entry into the stationary growth phase, also represents the activation signal for glycogen accumulation.Citation179 Besides, it is well known that cells in the stationary phase are significantly more tolerant to acid stress than those in the log phase of growth.Citation180 It is also worth noting that some of the regulators controlling glycogen metabolism, control the expression of genes that contribute to the response to specific GIT stress factors, suggesting that intracellular glycogen metabolism may confer a selective advantage in order to better cope with specific GIT stress factors. The specific contribution of glycogen metabolism toward acid tolerance has not been investigated, although glycogen reserves act as intracellular energy sources, which are required for the maintenance of pH homeostasis under acidic conditions supported by the extrusion of protons via the F0F1 H+/ATPase.Citation181 Thus, under acidic conditions, cells tend to adjust their metabolism, by increasing the synthesis of energy-rich intermediates (such as ATP and NADH) and/or glycogen.Citation182 A connection between acid and bile stress responses has also been proposed as intracellular accumulation of BAs leads to proton release and thus cytoplasmic acidification. Early reports have described that bile salts promote higher intracellular accumulation of glycogen in several enterobacteria.Citation91 For example, response to and survival under acidic conditions in E. coli is highly dependent on the alternative σS factor, RpoS, also known to control glycogen accumulation.Citation150 Furthermore, the AcrAB-TolC multidrug efflux system from enterobacteria, essential for bile resistance in Salmonella typhimurium, is activated as part of the RamA-controlled regulon, which is also known to regulate glg genes in other microorganisms.Citation183,Citation184
More recently, glg gene inactivation in the commensal L. acidophilus did not appear to affect survival in simulated gastric juices, indicating the absence of a role in acid tolerance, although it was shown to negatively affect survival in simulated intestinal juices, suggesting a role in survival to bile. Indeed strains carrying a ΔglgB or ΔglgP mutation were shown to elicit growth defects, including longer lag phases during growth in the presence of bile extracts, further supporting a contribution of this metabolic pathway in the gut ecosystem.Citation86
The role of glycogen accumulation in biofilm formation
In nature bacteria are rarely encountered in planktonic lifestyles but are organized in matrix-enclosed communities known as biofilms. Recent findings suggest that bacteria within the gut environment are mainly encountered in the form of intricate biofilms that establish close association with the intestinal epithelia.Citation185 Besides, biofilm lifestyles confer resistance to harsh environments thereby rendering biofilm-associated bacteria more resilient to environmental challenges such as those encountered in the gut. In enteric bacteria, in vitro studies have demonstrated a correlation between glycogen accumulation and biofilm formation. Similarly, studies in E. coli have revealed that biofilm formation is enhanced in mutants defective for CsrA, a global carbon regulator that also represses glycogen storage during active phases of bacterial growth. Besides, it has been reported that biofilm formation phenotypes are affected in mutants with altered glycogen accumulation and subsequent catabolism, as illustrated by the finding that in E. coli glycogen accumulation and catabolism through GlgP is required to promote biofilm formation.Citation186 Consistent with this notion, in some bifidobacteria, in which biofilm production has been demonstrated to occur in response to bile exposure, a glgP insertional mutant (a mutation expected to prevent glycogen degradation) rendered the strain defective in biofilm formation when exposed to high bile concentrations.Citation187,Citation188 However, it is worth noting that contradictory observations have been reported as a glycogen-deficient ΔglgC (glycogen synthesis) mutant was shown to accumulate high levels of biofilm in E. coli.Citation189 These observations clearly indicate that, glycogen metabolism and, particularly, degradation, affect biofilm formation. Further investigation is required so as to decipher the regulatory mechanisms connecting biofilm formation and glycogen metabolism although it has been speculated that glycogen catabolism can serve as energy and/or carbon source to promote biofilm formation.Citation162 In agreement with this hypothesis, the ability to utilize glycogen was demonstrated to be important for the transition between planktonic and biofilm lifestyles and enabled increased glucose uptake during pulses of limited glucose availability in E. coli.Citation161
Role of glycogen storage for colonization and fitness in the gut ecosystem
Deciphering the functions of gut microbiota members is important to understand the factors driving colonization, resilience or survival in the gut niche.Citation29 Since glycogen accumulation plays a role in survival under in vitro conditions, this bacterial metabolism is also thought to be a crucial mechanism involved in GIT colonization and fitness. Thus, this section focuses on several in vivo studies performed to determine the impact of bacterial glycogen storage on enhancing fitness in the gut ecosystem. It is possible that glycogen storage plays an important role in GIT colonization due to the necessity to support growth and/or to maintain viable in the intestinal environment when and where there is intense competition for (micro)nutrients.
The ability of L. acidophilus to synthesize and store glycogen was shown to support long-term colonization in the murine GIT.Citation86 In this in vivo study two bacterial strains, the parent L. acidophilus (able to synthesize and store glycogen) and its glgA mutant unable to synthesize glycogen were administered in a 1:1 ratio to germ-free (GF) mice. The results showed that colonization levels of the glgA mutant are significant lower compared to the parental strain for almost one month post-delivery. These findings represent compelling evidence that the ability of L. acidophilus to synthesize intracellular glycogen provides a competitive advantage that supports gut colonization. In the same study, the authors also showed that a glgA mutant is able to colonize the gut of GF mice in the absence of competition. However, when the parental strain was administered to the mice colonized with the glgA mutant the decline in the mutant abundance coincided with an increase of the parental population level. This supports the idea that intracellular glycogen storage plays a major role in the competitive fitness of L. acidophilus in the host environment. The importance of glycogen accumulation in murine GIT colonization has also been demonstrated using the commensal E. coli K-12. It has been shown that E. coli mutants which lack genes for glycogen accumulation are not able to colonize the murine GIT. However, by providing a constant supply of a readily metabolizable sugar in the animal’s drinking water, the competitive disadvantage of E. coli glycogen metabolism mutants was rescued, supporting the notion that glycogen storage may aid to maintain fitness when there is intense competition for resources and occasional nutrient deprivation.Citation43
The trait of glycogen storage in an ecological context has also been addressed by metagenomic and metatranscriptomic approaches. Metagenomic analysis can provide host-specific insights into diversity and functional potential of the gut microbiota. Studies such as the mouse intestinal bacterial collection (miBC) identified factors required for colonization in mice. This study described the presence of glycogen genes in the strains Blautia caecimuris, Cuneatibacter caecimuris, Extibacter muris, Irregularibacter muris, Longicatena caecimuris and Pasteurella caecimuris among others.Citation190 A metatranscriptomic study in Bacteroides defined in vivo fitness determinants for these gut members in a community context under different dietary conditions. Genes involved in glycogen storage were significantly upregulated in Ba. ovatus, Ba. cellulosyliticus and Ba. thetaiotaomicron, during intestinal colonization in mice. Thus, these genes appear to be important for colonization and survival in a given environmental contextCitation191. In another study involving the ruminal microbiome, the glycogen phosphorylase-encoding gene, among other functional features, was highly transcribed by 115 bacterial genera, mainly belonging to the phyla Pseudomonadota, Bacillota, and Actinomycetota.Citation192
Much information can be gained from the distribution of genes that are functionally important in complex microbial communities by targeting specific genes.Citation193,Citation194 Using targeted metagenomic sequencing, the gene encoding the GlgB enzyme (which is crucial for glycogen storage and metabolism)Citation195 was found to be present in 69 gut microbiomes from human, pig, cow and chicken hosts.Citation196 For example, glgB sequences in human gut microbiota members included Clostridia sp., Faecalibacterium sp. and Lachnospiraceae. The activity of glgB in the gut, and their influence on microbial dynamics, are likely to be associated with diet, as carbohydrates are indeed the direct target of this enzymatic activity.Citation196 Remarkably, a metatranscriptomic analysis of the human gut microbiome revealed that specific genes involved in carbohydrate metabolism, including glgB (glucan branching enzyme), were highly expressed by Fusobacteria. These data indicate that important functional aspects of low abundance bacterial species are active contributors to the gut ecology and host physiology.Citation197 In this regard, the pathway for glycogen synthesis was shown to be active in the gut by members of Escherichia, Sutterela, Enterobacter and Enterococcus.Citation198
Another metatranscriptomic study on metabolic adaptation of the human gut microbiota during pregnancy and infancy revealed that glgC, involved in glycogen synthesis, was upregulated in the maternal microbiota prior to birth.Citation199 It has also been demonstrated that in many bacterial species synthesis of glycogen is stimulated when glucose is abundant in the mediumCitation123. High glucose concentration in the gut environment can be found during the state of late pregnancy, where the ability of microbiota to transport and degrade a variety of carbohydrates is replaced by the overexpression of a single transporter capable of internalizing glucose, and of enzymes of the glycogen synthesis pathway.Citation199
All the above indicates that there is a correlation between the presence of glg genes and their expression among members of the gut ecosystem, supporting the notion that glycogen metabolism is an important fitness property for gut bacteria.
Glycogen and gut pathogenic bacteria
It has been suggested that glycogen storage increases bacterial virulence and survival at mucosal surfaces, although this view is still controversial. For example, it has been demonstrated that the ability of glycogen accumulation in Salmonella gallinarum and Salmonella pullorum does not confer a fitness increase but appeared to enhance long-term survival.Citation200 On the other hand, it has been shown that glycogen accumulation in Salmonella enterica is important for biofilm formation to increase its virulence capacity.Citation116 The pathogen V. cholerae is able to store glycogen in vivo and this mechanism plays an important role in the transition of V. cholerae between poor nutrient environment (such as an aquatic niche) and the host, because mutants that are either unable to store or degrade glycogen are attenuated for transmission.Citation121 Genome analysis of Clostridioides difficile comparing in vitro versus in vivo conditions revealed that the genes glgA, glgC and glgD (glgCDA operon) are upregulated under in vivo conditions, suggesting that C. difficile accumulates glycogen under such circumstances.Citation201 The latter work also indicated that glycogen accumulation in C. difficile is one of the strategies used by this bacterium to cope with temporary starvation conditions typical of gut colonization.Citation201 To further explore the possible link between glycogen storage and C. difficile virulence a mutant of this bacterium lacking glgC was shown to produce significantly fewer spores compared to the parental strain, indicating that glycogen storage is required for efficient sporulation in C. difficile.Citation202 While more studies are needed to address the precise role of bacterial glycogen storage in vivo under various conditions of host environmental nutrient and metabolic perturbation are needed, currently available data indicate that this mechanism is used as a strategy to survive challenges encountered in the host environment. Sporulation is a mode of long-term survival that has been described as a widespread strategy used by gut bacteria to survive under nutritional limitations or other stressful conditions, and to transit from the environment to the host.Citation203 Thus, in these bacteria which experience frequent oscillations of their environmental conditions, in particular when they transfer from a non-host environment to the gut of their host, glycogen accumulation is essential to survive outside the host.Citation121,Citation204 In conclusion, commensal or pathogenic bacteria that are able to synthesize and store glycogen survive better and longer than their isogenic derivatives that don’t possess the ability to build up such energy and carbon reserves.
Concluding remarks
Determining the functional attributes of the microbiome is essential to understand its role in host physiology, metabolism and disease and it has also prompted a wide range of biotechnological applications to improve human health. This includes the production of novel probiotics and prebiotics, among others, to modulate the gut microbiota. However, these technological developments require an in-depth knowledge of the adaptive mechanisms of these microbial communities to the human GIT. In this regard, these microorganisms must have developed metabolic strategies to efficiently colonize and survive in such a harsh, dynamic and highly competitive environment. The genetic capability for glycogen metabolism as present in a large proportion of enteric bacteria, supports the notion that this metabolic pathway enhances adaptation and performance of gut commensals in their specific ecosystem. Not surprisingly, accumulation of this polymer is interconnected to central carbon metabolism and several fundamental biological processes as demonstrated by its intricate regulatory mechanisms. Available information suggests that the ability to synthesize and store intracellular glycogen by gut bacteria is linked to their long-term survival, adaptation to periods of starvation, protection against a range of stressors relevant to the gut ecosystem while also being important, and in some cases essential, for colonization of the GIT ().
Figure 5. Role of glycogen metabolism in gut commensal bacteria.
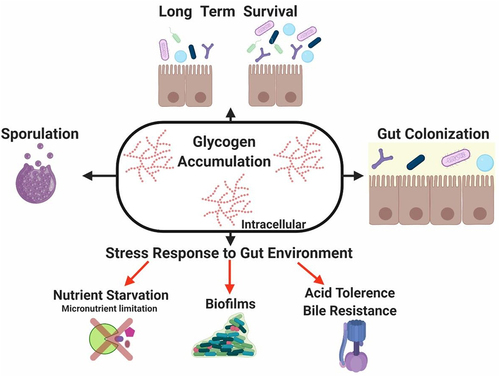
This metabolic feature shapes functionality and composition of gut microbiome from a novel perspective. While most of the findings in this field are based on in vitro studies, the role of glycogen metabolism from an ecological perspective has been addressed in this review including metagenomics and metatranscriptomics of complex microbial communities and in vivo studies. Functional understanding and delineating the diversity, extent and regulation of this metabolic pathway in the gut microbiota may provide novel clues to improve their performance within the gut ecosystem.
Disclosure statement
No potential conflict of interest was reported by the author(s).
Additional information
Funding
References
- Eckburg PB, Bik EM, Bernstein CN, Purdom E, Dethlefsen L, Sargent M, Gill SR, Nelson KE, Relman DA. Diversity of the human intestinal microbial flora. Science. 2005;308(5728):1635–27. doi:10.1126/science.1110591.
- Coyte KZ, Rakoff-Nahoum S. Understanding competition and cooperation within the mammalian gut microbiome. Curr Biol. 2019;29(11):R538–R44. doi:10.1016/j.cub.2019.04.017.
- Cani PD. Human gut microbiome: hopes, threats and promises. Gut. 2018;67(9):1716–1725. doi:10.1136/gutjnl-2018-316723.
- Lynch SV, Pedersen O, Phimister EG. The human intestinal microbiome in health and disease. N Engl J Med. 2016;375(24):2369–2379. doi:10.1056/NEJMra1600266.
- Clemente JC, Ursell LK, Parfrey LW, Knight R. The impact of the gut microbiota on human health: an integrative view. Cell. 2012;148(6):1258–1270. doi:10.1016/j.cell.2012.01.035.
- O’Hara AM, Shanahan F. The gut flora as a forgotten organ. EMBO Rep. 2006;7(7):688–693. doi:10.1038/sj.embor.7400731.
- Crowley EK, Long-Smith CM, Murphy A, Patterson E, Murphy K, O’Gorman DM, Stanton C, Nolan Y. Dietary supplementation with a magnesium-rich marine mineral blend enhances the diversity of gastrointestinal microbiota. Mar Drugs. 2018;16(6):216. doi:10.3390/md16060216.
- Bielik V, Kolisek M. Bioaccessibility and bioavailability of minerals in relation to a healthy gut microbiome. Int J Mol Sci. 2021;22(13):6803. doi:10.3390/ijms22136803.
- Rowland I, Gibson G, Heinken A, Scott K, Swann J, Thiele I, Tuohy K. Gut microbiota functions: metabolism of nutrients and other food components. Eur J Nutr. 2018;57(1):1–24. doi:10.1007/s00394-017-1445-8.
- de Vos WM, de Vos WM. Microbial biofilms and the human intestinal microbiome. NPJ Biofilms Microbiomes. 2015;1(1):15005. doi:10.1038/npjbiofilms.2015.5.
- Rossi M, Amaretti A, Raimondi S. Folate production by probiotic bacteria. Nutrients. 2011;3(1):118–134. doi:10.3390/nu3010118.
- Solopova A, Bottacini F, Venturi Degli Esposti E, Amaretti A, Raimondi S, Rossi M, van Sinderen D. Riboflavin biosynthesis and overproduction by a derivative of the human gut commensal Bifidobacterium longum subsp. infantis ATCC 15697. Front Microbiol. 2020;11:573335. doi:10.3389/fmicb.2020.573335.
- Leatham MP, Banerjee S, Autieri SM, Mercado-Lubo R, Conway T, Cohen PS. Precolonized human commensal Escherichia coli strains serve as a barrier to E. coli O157: H7 growth in the streptomycin-treated mouse intestine. Infect Immun. 2009;77(7):2876–2886. doi:10.1128/IAI.00059-09.
- Durso LM, Smith D, Hutkins RW. Measurements of fitness and competition in commensal Escherichia coli and E. coli O157: H7 strains. Appl Environ Microbiol. 2004;70(11):6466–6472. doi:10.1128/AEM.70.11.6466-6472.2004.
- Milani C, Duranti S, Bottacini F, Casey E, Turroni F, Mahony J, Belzer C, Delgado Palacio S, Arboleya Montes S, Mancabelli L, et al. The first microbial colonizers of the human gut: composition, activities, and health implications of the infant gut microbiota. Microbiol Mol Biol Rev. 2017;81(4). doi:10.1128/MMBR.00036-17.
- Mazmanian SK, Liu CH, Tzianabos AO, Kasper DL. An immunomodulatory molecule of symbiotic bacteria directs maturation of the host immune system. Cell. 2005;122(1):107–118. doi:10.1016/j.cell.2005.05.007.
- David LA, Maurice CF, Carmody RN, Gootenberg DB, Button JE, Wolfe BE, Ling AV, Devlin AS, Varma Y, Fischbach MA, et al. Diet rapidly and reproducibly alters the human gut microbiome. Nature. 2014;505(7484):559–563. doi:10.1038/nature12820.
- Gensollen T, Iyer SS, Kasper DL, Blumberg RS. How colonization by microbiota in early life shapes the immune system. Sci. 2016;352(6285):539–544. doi:10.1126/science.aad9378.
- Al Nabhani Z, Eberl G. Imprinting of the immune system by the microbiota early in life. Mucosal Immunol. 2020;13(2):183–189. doi:10.1038/s41385-020-0257-y.
- Ganal-Vonarburg SC, Hornef MW, Macpherson AJ. Microbial-host molecular exchange and its functional consequences in early mammalian life. Science. 2020;368(6491):604–607. doi:10.1126/science.aba0478.
- Ley RE, Turnbaugh PJ, Klein S, Gordon JI. Microbial ecology: human gut microbes associated with obesity. Nature. 2006;444(7122):1022–1023. doi:10.1038/4441022a.
- Sender R, Fuchs S, Milo R. Revised estimates for the number of human and bacteria cells in the body. PLoS Biol. 2016;14(8):e1002533. doi:10.1371/journal.pbio.1002533.
- Browne HP, Neville BA, Forster SC, Lawley TD. Transmission of the gut microbiota: spreading of health. Nat Rev Microbiol. 2017;15(9):531–543. doi:10.1038/nrmicro.2017.50.
- Louis P, Hold GL, Flint HJ. The gut microbiota, bacterial metabolites and colorectal cancer. Nat Rev Microbiol. 2014;12(10):661–672. doi:10.1038/nrmicro3344.
- Huttenhower C, Gevers D, Knight R, Abubucker S, Badger JH, Chinwalla AT, Creasy HH, Earl AH, FitzGerald MG, Fulton RS. Structure, function and diversity of the healthy human microbiome. Nature. 2012;486(7402):207–214.
- Arumugam M, Raes J, Pelletier E, Le Paslier D, Yamada T, Mende DR, Fernandes GR, Tap J, Bruls T, Batto J-M, et al. Enterotypes of the human gut microbiome. Nature. 2011;473(7346):174–180. doi:10.1038/nature09944.
- Iacob S, Iacob DG. Infectious threats, the intestinal barrier, and its trojan horse: dysbiosis. Front Microbiol. 2019;10:1676. doi:10.3389/fmicb.2019.01676.
- Yassour M, Vatanen T, Siljander H, Hamalainen AM, Harkonen T, Ryhanen SJ, Franzosa EA, Vlamakis H, Huttenhower C, Gevers D, et al. Natural history of the infant gut microbiome and impact of antibiotic treatment on bacterial strain diversity and stability. Sci Transl Med. 2016;8(343):343ra81. doi:10.1126/scitranslmed.aad0917.
- Pereira FC, Berry D. Microbial nutrient niches in the gut. Environ Microbiol. 2017;19(4):1366–1378. doi:10.1111/1462-2920.13659.
- Messer JS, Liechty ER, Vogel OA, Chang EB. Evolutionary and ecological forces that shape the bacterial communities of the human gut. Mucosal Immunol. 2017;10(3):567–579. doi:10.1038/mi.2016.138.
- Sekirov I, Russell SL, Antunes LC, Finlay BB. Gut microbiota in health and disease. Physiol Rev. 2010;90(3):859–904. doi:10.1152/physrev.00045.2009.
- Rothschild D, Weissbrod O, Barkan E, Kurilshikov A, Korem T, Zeevi D, Costea PI, Godneva A, Kalka IN, Bar N, et al. Environment dominates over host genetics in shaping human gut microbiota. Nature. 2018;555(7695):210–215. doi:10.1038/nature25973.
- Ghosh TS, Rampelli S, Jeffery IB, Santoro A, Neto M, Capri M, Giampieri E, Jennings A, Candela M, Turroni S, et al. Mediterranean diet intervention alters the gut microbiome in older people reducing frailty and improving health status: the NU-AGE 1-year dietary intervention across five European countries. Gut. 2020;69(7):gutjnl-2019–319654. doi:10.1136/gutjnl-2019-319654.
- Maldonado-Gomez MX, Martinez I, Bottacini F, O’Callaghan A, Ventura M, van Sinderen D, Hillmann B, Vangay P, Knights D, Hutkins R, et al. Stable engraftment of Bifidobacterium longum AH1206 in the human gut depends on individualized features of the resident microbiome. Cell Host & Microbe. 2016;20(4):515–526. doi:10.1016/j.chom.2016.09.001.
- Marchesi JR, Adams DH, Fava F, Hermes GD, Hirschfield GM, Hold G, Quraishi MN, Kinross J, Smidt H, Tuohy KM, et al. The gut microbiota and host health: a new clinical frontier. Gut. 2016;65(2):330–339. doi:10.1136/gutjnl-2015-309990.
- Glover JS, Ticer TD, Engevik MA. Characterizing the mucin-degrading capacity of the human gut microbiota. Sci Rep. 2022;12(1):8456. doi:10.1038/s41598-022-11819-z.
- Townsend GE 2nd, Han W, Schwalm ND 3rd, Raghavan V, Barry NA, Goodman AL, Groisman EA. Dietary sugar silences a colonization factor in a mammalian gut symbiont. Proc Natl Acad Sci USA. 2019;116(1):233–238. doi:10.1073/pnas.1813780115.
- Fu X, Liu Z, Zhu C, Mou H, Kong Q. Nondigestible carbohydrates, butyrate, and butyrate-producing bacteria. Crit Rev Food Sci Nutr. 2019;59(sup1):S130–S52. doi:10.1080/10408398.2018.1542587.
- Liu H, Wang J, He T, Becker S, Zhang G, Li D, Ma X. Butyrate: a double-edged sword for health? Adv Nutr. 2018;9(1):21–29. doi:10.1093/advances/nmx009.
- McCarville JL, Chen GY, Cuevas VD, Troha K, Ayres JS. Microbiota metabolites in health and disease. Annu Rev Immunol. 2020;38(1):147–170. doi:10.1146/annurev-immunol-071219-125715.
- Miranda RL, Conway T, Leatham MP, Chang DE, Norris WE, Allen JH, Stevenson SJ, Laux DC, Cohen PS. Glycolytic and gluconeogenic growth of Escherichia coli O157: H7 (EDL933) and E. coli K-12 (MG1655) in the mouse intestine. Infect Immun. 2004;72(3):1666–1676. doi:10.1128/IAI.72.3.1666-1676.2004.
- Morin M, Ropers D, Cinquemani E, Portais JC, Enjalbert B, Cocaign-Bousquet M, Romeo T, Greenberg EP. The CSR system regulates Escherichia coli fitness by controlling glycogen accumulation and energy levels. mBio. 2017;8(5). doi:10.1128/mBio.01628-17.
- Jones SA, Jorgensen M, Chowdhury FZ, Rodgers R, Hartline J, Leatham MP, Struve C, Krogfelt KA, Cohen PS, Conway T, et al. Glycogen and maltose utilization by Escherichia coli O157: H7 in the mouse intestine. Infect Immun. 2008;76(6):2531–2540. doi:10.1128/IAI.00096-08.
- Fabich AJ, Jones SA, Chowdhury FZ, Cernosek A, Anderson A, Smalley D, McHargue JW, Hightower GA, Smith JT, Autieri SM, et al. Comparison of carbon nutrition for pathogenic and commensal Escherichia coli strains in the mouse intestine. Infect Immun. 2008;76(3):1143–1152. doi:10.1128/IAI.01386-07.
- Kamada N, Chen GY, Inohara N, Nunez G. Control of pathogens and pathobionts by the gut microbiota. Nat Immunol. 2013;14(7):685–690. doi:10.1038/ni.2608.
- Ventura M, O’Flaherty S, Claesson MJ, Turroni F, Klaenhammer TR, van Sinderen D, O’Toole PW. Genome-scale analyses of health-promoting bacteria: probiogenomics. Nat Rev Microbiol. 2009;7(1):61–71. doi:10.1038/nrmicro2047.
- Seibold G, Dempf S, Schreiner J, Eikmanns BJ. Glycogen formation in Corynebacterium glutamicum and role of ADP-glucose pyrophosphorylase. Microbiology. 2007;153(Pt 4):1275–1285. doi:10.1099/mic.0.2006/003368-0.
- Cifuente JO, Comino N, Trastoy B, D’Angelo C, Guerin ME. Structural basis of glycogen metabolism in bacteria. Biochem J. 2019;476(14):2059–2092. doi:10.1042/BCJ20170558.
- Ayres JS. Cooperative microbial tolerance behaviors in host-microbiota mutualism. Cell. 2016;165(6):1323–1331. doi:10.1016/j.cell.2016.05.049.
- Gonzalez-Rodriguez I, Ruiz L, Gueimonde M, Margolles A, Sanchez B. Factors involved in the colonization and survival of bifidobacteria in the gastrointestinal tract. FEMS Microbiol Lett. 2013;340(1):1–10. doi:10.1111/1574-6968.12056.
- Fallingborg J. Intraluminal pH of the human gastrointestinal tract. Dan Med Bull. 1999;46:183–196.
- Koziolek M, Grimm M, Becker D, Iordanov V, Zou H, Shimizu J, Wanke C, Garbacz G, Weitschies W. Investigation of pH and temperature profiles in the GI tract of fasted human subjects using the intellicap® system. J Pharm Sci. 2015;104(9):2855–2863. doi:10.1002/jps.24274.
- Cheng S, Zhu L, Faden HS. Interactions of bile acids and the gut microbiota: learning from the differences in Clostridium difficile infection between children and adults. Physiol Genomics. 2019;51(6):218–223. doi:10.1152/physiolgenomics.00034.2019.
- Drasar BS, Hill MJ, Shiner M. The deconjugation of bile salts by human intestinal bacteria. Lancet. 1966;1(7449):1237–1238. doi:10.1016/S0140-6736(66)90242-X.
- Ferrari A, Pacini N, Canzi E. A note on bile acids transformations by strains of Bifidobacterium. J Appl Bacteriol. 1980;49(2):193–197. doi:10.1111/j.1365-2672.1980.tb05117.x.
- Gilliland SE, Speck ML. Deconjugation of bile acids by intestinal lactobacilli. Appl Environ Microbiol. 1977;33(1):15–18. doi:10.1128/aem.33.1.15-18.1977.
- Moser SA, Savage DC. Bile salt hydrolase activity and resistance to toxicity of conjugated bile salts are unrelated properties in lactobacilli. Appl Environ Microbiol. 2001;67(8):3476–3480. doi:10.1128/AEM.67.8.3476-3480.2001.
- Ridlon JM, Kang DJ, Hylemon PB. Bile salt biotransformations by human intestinal bacteria. J Lipid Res. 2006;47(2):241–259. doi:10.1194/jlr.R500013-JLR200.
- Fanning S, Hall LJ, Cronin M, Zomer A, MacSharry J, Goulding D, O’Connell Motherway M, Shanahan F, Nally K, Dougan G, et al. Bifidobacterial surface-exopolysaccharide facilitates commensal-host interaction through immune modulation and pathogen protection. Proc Natl Acad Sci U S A. 2012;109(6):2108–2113. doi:10.1073/pnas.1115621109.
- Ruiz L, Zomer A, O’Connell-Motherway M, van Sinderen D, Margolles A. Discovering novel bile protection systems in Bifidobacterium breve UCC2003 through functional genomics. Appl Environ Microbiol. 2012;78(4):1123–1131. doi:10.1128/AEM.06060-11.
- Ruas-Madiedo P, Gueimonde M, Arigoni F, de Los Reyes-Gavilan CG, Margolles A, de Los Reyes-Gavilán CG. Bile affects the synthesis of exopolysaccharides by Bifidobacterium animalis. Appl Environ Microbiol. 2009;75(4):1204–1207. doi:10.1128/AEM.00908-08.
- Du D, Wang Z, James NR, Voss JE, Klimont E, Ohene-Agyei T, Venter H, Chiu W, Luisi BF. Structure of the AcrAB–TolC multidrug efflux pump. Nature. 2014;509(7501):512–515. doi:10.1038/nature13205.
- Laparra JM, Sanz Y. Comparison of in vitro models to study bacterial adhesion to the intestinal epithelium. Lett Appl Microbiol. 2009;49(6):695–701. doi:10.1111/j.1472-765X.2009.02729.x.
- Tuomola E, Crittenden R, Playne M, Isolauri E, Salminen S. Quality assurance criteria for probiotic bacteria. Am J Clin Nutr. 2001;73(2 Suppl):393S–398S. doi:10.1093/ajcn/73.2.393s.
- Saffarian A, Mulet C, Regnault B, Amiot A, Tran-Van-Nhieu J, Ravel J, Sobhani I, Sansonetti PJ, Pédron T, et al. Crypt- and mucosa-associated core microbiotas in humans and their alteration in colon cancer patients and mucosa-associated core microbiotas in humans and their alteration in colon cancer patients. mBio. 2019;10(4). doi:10.1128/mBio.01315-19.
- Pedron T, Nigro G, Sansonetti PJ. From homeostasis to pathology: decrypting microbe–host symbiotic signals in the intestinal crypt. Philos Trans R Soc Lond B Biol Sci. 2016;371(1707):20150500. doi:10.1098/rstb.2015.0500.
- Capurso L, Delle Fave G, Morelli L. Probiotics, prebiotics, and new foods. J Clin Gastroenterol. 2008;42(Suppl 3 Pt 2):S155. doi:10.1097/MCG.0b013e31817e55b2.
- Castro-Bravo N, Sánchez B, Margolles A, Ruas-Madiedo P. Chapter 10 - biological activities and applications of bifidobacterial exopolysaccharides: from the bacteria and host perspective. In: Mattarelli P, Biavati B, Holzapfel W Wood B, editors. The bifidobacteria and related. Organisms:Academic Press; 2018. pp. 177–193. doi:10.1016/B978-0-12-805060-6.00010-7.
- Lee IC, Caggianiello G, van S II, Taverne N, Meijerink M, PA B, Spano G, Kleerebezem M. Strain-specific features of extracellular polysaccharides and their impact on lactobacillus plantarum-host interactions. Appl Environ Microbiol. 2016;82(13):3959–3970. doi:10.1128/AEM.00306-16.
- Dertli E, Mayer MJ, Narbad A. Impact of the exopolysaccharide layer on biofilms, adhesion and resistance to stress in Lactobacillus johnsonii FI9785. BMC Microbiol. 2015;15(1):8. doi:10.1186/s12866-015-0347-2.
- Denou E, Pridmore RD, Berger B, Panoff JM, Arigoni F, Brussow H. Identification of genes associated with the long-gut-persistence phenotype of the probiotic Lactobacillus johnsonii strain NCC533 using a combination of genomics and transcriptome analysis. J Bacteriol. 2008;190(9):3161–3168. doi:10.1128/JB.01637-07.
- Tahoun A, Masutani H, El-Sharkawy H, Gillespie T, Honda RP, Kuwata K, Inagaki M, Yabe T, Nomura I, Suzuki T, et al. Capsular polysaccharide inhibits adhesion of Bifidobacterium longum 105-A to enterocyte-like Caco-2 cells and phagocytosis by macrophages. Gut Pathog. 2017;9(1):27. doi:10.1186/s13099-017-0177-x.
- Lebeer S, Verhoeven TL, Francius G, Schoofs G, Lambrichts I, Dufrene Y, Vanderleyden J, De Keersmaecker SCJ. Identification of a gene cluster for the biosynthesis of a long, galactose-rich exopolysaccharide in lactobacillus rhamnosus gg and functional analysis of the priming glycosyltransferase. Appl Environ Microbiol. 2009;75(11):3554–3563. doi:10.1128/AEM.02919-08.
- Craig L, Li J. Type IV pili: paradoxes in form and function. Curr Opin Struct Biol. 2008;18(2):267–277. doi:10.1016/j.sbi.2007.12.009.
- Hendrickx AP, Budzik JM, Oh SY, Schneewind O. Architects at the bacterial surface - sortases and the assembly of pili with isopeptide bonds. Nat Rev Microbiol. 2011;9(3):166–176. doi:10.1038/nrmicro2520.
- Reunanen J, von Ossowski I, Hendrickx AP, Palva A, de Vos WM. Characterization of the SpaCBA pilus fibers in the probiotic Lactobacillus rhamnosus GG. Appl Environ Microbiol. 2012;78(7):2337–2344. doi:10.1128/AEM.07047-11.
- Turroni F, Foroni E, Serafini F, Viappiani A, Montanini B, Bottacini F, Ferrarini A, Bacchini PL, Rota C, Delledonne M, et al. Ability of Bifidobacterium breve to grow on different types of milk: exploring the metabolism of milk through genome analysis. Appl Environ Microbiol. 2011;77(20):7408–7417. doi:10.1128/AEM.05336-11.
- Oxaran V, Ledue-Clier F, Dieye Y, Herry JM, Pechoux C, Meylheuc T, Briandet R, Juillard V, Piard JC. Pilus biogenesis in Lactococcus lactis: molecular characterization and role in aggregation and biofilm formation. PLoS One. 2012;7(12):e50989. doi:10.1371/journal.pone.0050989.
- Yu X, Jaatinen A, Rintahaka J, Hynonen U, Lyytinen O, Kant R, Åvall-Jääskeläinen S, von Ossowski I, Palva A. Human gut-commensalic lactobacillus ruminis ATCC 25644 displays sortase-assembled surface piliation: phenotypic characterization of its fimbrial operon through in silico predictive analysis and recombinant expression in Lactococcus lactis. PLoS One. 2015;10(12):e0145718. doi:10.1371/journal.pone.0145718.
- Proft T, Baker EN. Pili in gram-negative and gram-positive bacteria - structure, assembly and their role in disease. Cell Mol Life Sci. 2009;66(4):613–635. doi:10.1007/s00018-008-8477-4.
- O’Connell Motherway M, Houston A, O’Callaghan G, Reunanen J, O’Brien F, O’Driscoll T, Casey PG, de Vos WM, van Sinderen D, Shanahan F. A Bifidobacterial pilus-associated protein promotes colonic epithelial proliferation. Mol Microbiol. 2019;111(1):287–301. doi:10.1111/mmi.14155.
- O’Connell Motherway M, Zomer A, Leahy SC, Reunanen J, Bottacini F, Claesson MJ, O'Brien F, Flynn K, Casey PG, Moreno Munoz JA, et al. Functional genome analysis of Bifidobacterium breve UCC2003 reveals type IVb tight adherence (Tad) pili as an essential and conserved host-colonization factor. Proc Natl Acad Sci U S A. 2011;108(27):11217–11222. doi:10.1073/pnas.1105380108.
- Zmasek CM, Godzik A. Phylogenomic analysis of glycogen branching and debranching enzymatic duo. BMC Evol Biol. 2014;14(1):183. doi:10.1186/s12862-014-0183-2.
- Flint HJ, Scott KP, Duncan SH, Louis P, Forano E. Microbial degradation of complex carbohydrates in the gut. Gut Microbes. 2012;3(4):289–306. doi:10.4161/gmic.19897.
- Porter NT, Martens EC. The critical roles of polysaccharides in gut microbial ecology and physiology. Annu Rev Microbiol. 2017;71(1):349–369. doi:10.1146/annurev-micro-102215-095316.
- Goh YJ, Klaenhammer TR. Insights into glycogen metabolism in Lactobacillus acidophilus: impact on carbohydrate metabolism, stress tolerance and gut retention. Microb Cell Fact. 2014;13(1):94. doi:10.1186/s12934-014-0094-3.
- Park K-H. Roles of enzymes in glycogen metabolism and degradation in Escherichia coli. J Appl Glycosci. 2015;62(2):37–45. doi:10.5458/jag.jag.JAG-2015_005.
- Wilson WA, Roach PJ, Montero M, Baroja-Fernandez E, Munoz FJ, Eydallin G, Viale AM, Pozueta-Romero J. Regulation of glycogen metabolism in yeast and bacteria. FEMS Microbiol Rev. 2010;34(6):952–985. doi:10.1111/j.1574-6976.2010.00220.x.
- Cedergren B, Holme T. On the glycogen in Escherichia coli B; electron microscopy of ultrathin sections of cells. J Ultrastruct Res. 1959;3(1):70–73. doi:10.1016/S0022-5320(59)80016-2.
- Strydom L, Jewell J, Meier MA, George GM, Pfister B, Zeeman S, Kossmann J, Lloyd JR. Analysis of genes involved in glycogen degradation in Escherichia coli. FEMS Microbiol Lett. 2017;364(3):fnx016. doi:10.1093/femsle/fnx016.
- Levine S, Stevenson HJ, Tabor EC, Bordner RH, Chambers LA. Glycogen of enteric bacteria. J Bacteriol. 1953;66(6):664–670. doi:10.1128/jb.66.6.664-670.1953.
- Strange RE, Dark FA, Ness AG. The survival of stationary phase aerobacter aerogenes stored in aqueous suspension. J Gen Microbiol. 1961;25(1):61–76. doi:10.1099/00221287-25-1-61.
- Wilkinson JF. Carbon and energy storage in bacteria. J Gen Microbiol. 1963;32(2):171–176. doi:10.1099/00221287-32-2-171.
- Lindner JG, Marcelis JH, de Vos NM, Hoogkamp-Korstanje JA, De Vos NM. Intracellular polysaccharide of Bacteroides fragilis. J Gen Microbiol. 1979;111(1):93–99. doi:10.1099/00221287-111-1-93.
- Marr AG, Nilson EH, Clark DJ. The maintenance requirement of Escherichia coli. Ann N Y Acad Sci. 1963;102(3):536–548. doi:10.1111/j.1749-6632.1963.tb13659.x.
- Mallette MF. Validity of the concept of energy of maintenance. Ann N Y Acad Sci. 1963;102(3):521–535. doi:10.1111/j.1749-6632.1963.tb13658.x.
- Gronlund AF, Campbell JJ. Nitrogenous substrates of endogenous respiration in Pseudomonas Aeruginosa. J Bacteriol. 1963;86(1):58–66. doi:10.1128/jb.86.1.58-66.1963.
- Almagro G, Viale AM, Montero M, Rahimpour M, Munoz FJ, Baroja-Fernandez E, Bahaji A, Zúñiga M, González-Candelas F, Pozueta-Romero J, et al. Comparative genomic and phylogenetic analyses of Gammaproteobacterial glg genes traced the origin of the Escherichia coli glycogen glgBXCAP operon to the last common ancestor of the sister orders Enterobacteriales and Pasteurellales. PLoS One. 2015;10(1):e0115516. doi:10.1371/journal.pone.0115516.
- Patel GB, Breuil C. Accumulation of an iodophilic polysaccharide during growth of Acetivibrio cellulolyticus on cellobiose. Arch Microbiol. 1981;129(4):265–267. doi:10.1007/BF00414695.
- Shaw DH, Squires MJ. Optional production and utilization of polysaccharide by Aeromonas hydrophila. Arch Microbiol. 1980;125(1–2):83–87. doi:10.1007/BF00403202.
- Strange RE. Induced enzyme synthesis in aqueous suspensions of starved stationary phase aerobacter aerogenes. Nature. 1961;191(4795):1272–1273. doi:10.1038/1911272a0.
- Barry C, Gavard R, Milhaud G, Aubert JP. The glycogen of Bacillus megatherium. C R Hebd Seances Acad Sci. 1952;235:1062–1064.
- Kiel JA, Boels JM, Beldman G, Venema G. Glycogen in Bacillus subtilis: molecular characterization of an operon encoding enzymes involved in glycogen biosynthesis and degradation. Mol Microbiol. 1994;11(1):203–218. doi:10.1111/j.1365-2958.1994.tb00301.x.
- Whyte JN, Strasdine GA. An intracellular alpha-D-glucan from Clostridium botulinum, type E. Carbohydr Res. 1972;25(2):435–441. doi:10.1016/S0008-6215(00)81655-9.
- Seibold GM, Eikmanns BJ. Inactivation of the phosphoglucomutase gene pgm in Corynebacterium glutamicum affects cell shape and glycogen metabolism. Biosci Rep. 2013;33(4). doi:10.1042/BSR20130076.
- Seibold GM, Breitinger KJ, Kempkes R, Both L, Kramer M, Dempf S, Eikmanns BJ. The glgB-encoded glycogen branching enzyme is essential for glycogen accumulation in Corynebacterium glutamicum. Microbiology. 2011;157(11):3243–3251. doi:10.1099/mic.0.051565-0.
- Holme T, Palmstierna H. Changes in glycogen and nitrogen-containing compounds in Escherichia coli b during growth in deficient media. I. Nitrogen and Carbon Starvation. Acta Chem Scan.1956;10:578–586.
- Fung T, Kwong N, van der Zwan T, Wu M. Residual glycogen metabolism in Escherichia coli is specific to the limiting macronutrient and varies during stationary phase. J Exp Microbiol Immunol. 2013;17:83–87.
- Wang L, Liu Q, Tan X, Wang Z, Wang M, Wise MJ, Li C, Ma C, Li E, Deng B, et al. Molecular structure of glycogen in Escherichia coli. Biomacromolecules. 2019;20(7):2821–2829. doi:10.1021/acs.biomac.9b00586.
- Stewart CS, Paniagua C, Dinsdale D, Cheng KJ, Garrow SH. Selective isolation and characteristics of Bacteriodes succinogenes from the rumen of a cow. Appl Environ Microb. 1981;41(2):504–510. doi:10.1128/aem.41.2.504-510.1981.
- Gaudet G, Forano E, Dauphin G, Delort AM. Futile cycling of glycogen in Fibrobacter succinogenes as shown by in situ 1H-NMR and 13C-NMR investigation. Eur J Biochem. 1992;207(1):155–162. doi:10.1111/j.1432-1033.1992.tb17032.x.
- Goh YJ, Klaenhammer TR. A functional glycogen biosynthesis pathway in Lactobacillus acidophilus: expression and analysis of the glg operon. Mol Microbiol. 2013;89(6):1187–1200. doi:10.1111/mmi.12338.
- Cheng KJ, Hironaka R, Roberts DWA, Costerton JW. Cytoplasmic glycogen inclusions in cells of anaerobic gram-negative rumen bacteria. Can J Microbiol. 1973;19(12):1501–1506. doi:10.1139/m73-244.
- Lou J, Dawson KA, Strobel HJ. Glycogen formation by the ruminal bacterium prevotella ruminicola. Appl Environ Microb. 1997;63(4):1483–1488. doi:10.1128/aem.63.4.1483-1488.1997.
- Cheng KJ, Brown RG, Costerton JW. Characterization of a cytoplasmic reserve glucan from ruminococcus albus. Appl Environ Microbiol. 1977;33(3):718–724. doi:10.1128/aem.33.3.718-724.1977.
- Bonafonte MA, Solano C, Sesma B, Alvarez M, Montuenga L, García-Ros D, Gamazo C. The relationship between glycogen synthesis, biofilm formation and virulence in Salmonella enteritidis. FEMS Microbiol Lett. 2000;191(1):31–36. doi:10.1111/j.1574-6968.2000.tb09315.x.
- Kamio Y, Terawaki Y, Nakajima T, Matsuda K. Structure of glycogen produced by selenomonas ruminantium. Agric Biol Chem. 1981;45(1):209–216. doi:10.1271/bbb1961.45.209.
- Wallace RJ. Cytoplasmic reserve polysaccharide of Selenomonas ruminantium. Appl Environ Microb. 1980;39(3):630. doi:10.1128/aem.39.3.630-634.1980.
- McFarland CR, Snyder TL, McKenzie R. Polysaccharide storage in different streptococci. Microbios. 1984;40:7–14.
- van Houte J, Jansen HM. Role of glycogen in survival of Streptococcus mitis. J Bacteriol. 1970;101(3):1083–1085. doi:10.1128/jb.101.3.1083-1085.1970.
- Bourassa L, Camilli A. Glycogen contributes to the environmental persistence and transmission of Vibrio cholerae. Mol Microbiol. 2009;72(1):124–138. doi:10.1111/j.1365-2958.2009.06629.x.
- Preiss J. Bacterial glycogen synthesis and its regulation. Annu Rev Microbiol. 1984;38(1):419–458. doi:10.1146/annurev.mi.38.100184.002223.
- Preiss J, Romeo T. Physiology, biochemistry and genetics of bacterial glycogen synthesis. Adv Microb Physiol. 1989;30:183–238.
- Dawes EA, Ribbons DW. Studies on the endogenous metabolism of Escherichia coli. Biochem J. 1965;95(2):332–343. doi:10.1042/bj0950332.
- Klotz A, Forchhammer K. Glycogen, a major player for bacterial survival and awakening from dormancy. Future Microbiol. 2017;12(2):101–104. doi:10.2217/fmb-2016-0218.
- Henrissat B, Deleury E, Coutinho PM. Glycogen metabolism loss: a common marker of parasitic behaviour in bacteria? Trends Genet. 2002;18(9):437–440. doi:10.1016/S0168-9525(02)02734-8.
- Preiss J. Bacterial glycogen inclusions: enzymology and regulation of synthesis. In: Shively J, editor. Inclusions in prokaryotes. Berlin, HeidelbergBerlin Heidelberg:Springer; 2006. pp. 71–108. doi:10.1007/3-540-33774-1_4.
- Alonso-Casajus N, Dauvillee D, Viale AM, Munoz FJ, Baroja-Fernandez E, Moran-Zorzano MT, Eydallin G, Ball S, Pozueta-Romero J. Glycogen phosphorylase, the product of the glgP Gene, catalyzes glycogen breakdown by removing glucose units from the nonreducing ends in Escherichia coli. J Bacteriol. 2006;188(14):5266–5272. doi:10.1128/JB.01566-05.
- Dauvillee D, Kinderf IS, Li Z, Kosar-Hashemi B, Samuel MS, Rampling L, Ball S, Morell MK. Role of the Escherichia coli glgX gene in glycogen metabolism. J Bacteriol. 2005;187(4):1465–1473. doi:10.1128/JB.187.4.1465-1473.2005.
- O’Callaghan A, van Sinderen D. Bifidobacteria and their role as members of the human gut microbiota. Front Microbiol. 2016;7:925.
- Schneider D, Bruton CJ, Chater KF. Duplicated gene clusters suggest an interplay of glycogen and trehalose metabolism during sequential stages of aerial mycelium development in Streptomyces coelicolor A3(2). Mol Gen Genet. 2000;263(3):543–553. doi:10.1007/s004380051200.
- Chandra G, Chater KF, Bornemann S. Unexpected and widespread connections between bacterial glycogen and trehalose metabolism. Microbiology. 2011;157(6):1565–1572. doi:10.1099/mic.0.044263-0.
- Park JT, Shim JH, Tran PL, Hong IH, Yong HU, Oktavina EF, Nguyen HD, Kim J-W, Lee TS, Park S-H, et al. Role of maltose enzymes in glycogen synthesis by Escherichia coli. J Bacteriol. 2011;193(10):2517–2526. doi:10.1128/JB.01238-10.
- Wang L, Wise MJ. Glycogen with short average chain length enhances bacterial durability. Naturwissenschaften. 2011;98(9):719–729. doi:10.1007/s00114-011-0832-x.
- Nayfach S, Pollard KS. Average genome size estimation improves comparative metagenomics and sheds light on the functional ecology of the human microbiome. Genome Biol. 2015;16(1):51. doi:10.1186/s13059-015-0611-7.
- Nelson KE, Weinstock GM, Highlander SK, Worley KC, Creasy HH, Wortman JR, Rusch DB, Mitreva M, Sodergren E, Chinwalla AT, et al. A catalog of reference genomes from the human microbiome. Science. 2010;328(5981):994–999. doi:10.1126/science.1183605.
- Milani C, Lugli GA, Duranti S, Turroni F, Mancabelli L, Ferrario C, Mangifesta M, Hevia A, Viappiani A, Scholz M, et al. Bifidobacteria exhibit social behavior through carbohydrate resource sharing in the gut. Sci Rep. 2015;5(1):15782. doi:10.1038/srep15782.
- Pasolli E, De Filippis F, Mauriello IE, Cumbo F, Walsh AM, Leech J, Cotter PD, Segata N, Ercolini D. Large-scale genome-wide analysis links lactic acid bacteria from food with the gut microbiome. Nat Commun. 2020;11(1):2610. doi:10.1038/s41467-020-16438-8.
- Kaznadzey A, Shelyakin P, Belousova E, Eremina A, Shvyreva U, Bykova D, Emelianenko V, Korosteleva A, Tutukina M, Gelfand MS, et al. The genes of the sulphoquinovose catabolism in Escherichia coli are also associated with a previously unknown pathway of lactose degradation. Sci Rep. 2018;8(1):3177. doi:10.1038/s41598-018-21534-3.
- Romeo T, Black J, Preiss J. Genetic regulation of glycogen biosynthesis inEscherichia coli: In vivo effects of the catabolite repression and stringent response systems inglg gene expression. Curr Microbiol. 1990;21(2):131–137. doi:10.1007/BF02091831.
- Takata H, Takaha T, Okada S, Takagi M, Imanaka T. Characterization of a gene cluster for glycogen biosynthesis and a heterotetrameric ADP-glucose pyrophosphorylase from Bacillus stearothermophilus. J Bacteriol. 1997;179(15):4689–4698. doi:10.1128/jb.179.15.4689-4698.1997.
- Görke B, Stülke J. Carbon catabolite repression in bacteria: many ways to make the most out of nutrients. Nat Rev Microbiol. 2008;6(8):613–624. doi:10.1038/nrmicro1932.
- Barrangou R, Azcarate-Peril MA, Duong T, Conners SB, Kelly RM, Klaenhammer TR. Global analysis of carbohydrate utilization by Lactobacillus acidophilus using cDNA microarrays. Proc Natl Acad Sci USA. 2006;103(10):3816. doi:10.1073/pnas.0511287103.
- Handtke S, Albrecht D, Otto A, Becher D, Hecker M, Voigt B. The proteomic response of bacillus pumilus cells to glucose starvation. Proteomics. 2018;18(1):1700109. doi:10.1002/pmic.201700109.
- Montero M, Eydallin G, Viale Alejandro A, Almagro G, Muñoz Francisco F, Rahimpour M, Sesma M, Baroja-Fernández E, Pozueta-Romero J. Escherichia coli glycogen metabolism is controlled by the PhoP-PhoQ regulatory system at submillimolar environmental Mg2+ concentrations, and is highly interconnected with a wide variety of cellular processes. Biochem J. 2009;424(1):129–141. doi:10.1042/BJ20090980.
- Ronneau S, Hallez R. Make and break the alarmone: regulation of (p)ppGpp synthetase/hydrolase enzymes in bacteria. FEMS Microbiol Rev. 2019;43(4):389–400. doi:10.1093/femsre/fuz009.
- Durfee T, Hansen A-M, Zhi H, Blattner FR, Jin DJ. Transcription profiling of the stringent response in Escherichia coli. J Bacteriol. 2008;190(3):1084. doi:10.1128/JB.01092-07.
- Magnusson LU, Farewell A, Nyström T. ppGpp: a global regulator in Escherichia coli. Trends Microbiol. 2005;13(5):236–242. doi:10.1016/j.tim.2005.03.008.
- Britton RA, Eichenberger P, Gonzalez-Pastor JE, Fawcett P, Monson R, Losick R, Grossman AD. Genome-wide analysis of the stationary-phase sigma factor (Sigma-H) Regulon of Bacillus subtilis. J Bacteriol. 2002;184(17):4881. doi:10.1128/JB.184.17.4881-4890.2002.
- Slauch J, Taylor R, Maloy S. Survival in a cruel world: how Vibrio cholerae and Salmonella respond to an unwilling host. Genes Dev. 1997;11(14):1761–1774. doi:10.1101/gad.11.14.1761.
- Liu MY, Gui G, Wei B, Preston JF, Oakford L, Yüksel Ü, Giedroc DP, Romeo T. The RNA molecule CsrB binds to the global regulatory protein CsrA and antagonizes its activity in Escherichia coli. J Biol Chem. 1997;272(28):17502–17510. doi:10.1074/jbc.272.28.17502.
- Berndt V, Beckstette M, Volk M, Dersch P, Bronstrup M. Metabolome and transcriptome-wide effects of the carbon storage regulator a in enteropathogenic Escherichia coli. Sci Rep. 2019;9(1):138. doi:10.1038/s41598-018-36932-w.
- Romeo T. Global regulation by the small RNA-binding protein CsrA and the non-coding RNA molecule CsrB. Mol Microbiol. 1998;29(6):1321–1330. doi:10.1046/j.1365-2958.1998.01021.x.
- Liaw SJ, Lai HC, Ho SW, Luh KT, Wang WB. Role of RsmA in the regulation of swarming motility and virulence factor expression in Proteus mirabilis. J Med Microbiol. 2003;52(Pt 1):19–28. doi:10.1099/jmm.0.05024-0.
- Preiss J. Regulation of adenosine diphosphate glucose pyrophosphorylase. Adv Enzymol Relat Areas Mol Biol. 1978;46:317–381.
- Asención Diez MD, Demonte AM, Syson K, Arias DG, Gorelik A, Guerrero SA, Bornemann S, Iglesias AA. Allosteric regulation of the partitioning of glucose-1-phosphate between glycogen and trehalose biosynthesis in Mycobacterium tuberculosis. Biochim et Biophys Acta (BBA) - Gen Subj. 2015;1850(1):13–21. doi:10.1016/j.bbagen.2014.09.023.
- Cereijo AE, Asencion Diez MD, Ballicora MA, Iglesias AA, Metcalf WW. Regulatory properties of the ADP-glucose pyrophosphorylase from the clostridial firmicutes member ruminococcus albus. J Bacteriol. 2018;200(17). doi:10.1128/JB.00172-18.
- Ballicora MA, Iglesias AA, Preiss J. ADP-glucose pyrophosphorylase, a regulatory enzyme for bacterial glycogen synthesis. Microbiol Mol Biol R. 2003;67(2):213. doi:10.1128/MMBR.67.2.213-225.2003.
- Yung SG, Paule M, Beggs R, Greenberg E, Preiss J. Biosynthesis of bacterial glycogen: characterization of adenosine diphosphate glucose synthetases from Enterobacter hafniae and Aeromonas hydrophila. Arch Microbiol. 1984;138(1):1–8. doi:10.1007/BF00425398.
- Hengge-Aronis R, Fischer D. Identification and molecular analysis of glgS, a novel growth-phase-regulated and rpoS-dependent gene involved in glycogen synthesis in Escherichia coli. Mol Microbiol. 1992;6(14):1877–1886. doi:10.1111/j.1365-2958.1992.tb01360.x.
- Sekar K, Linker SM, Nguyen J, Grünhagen A, Stocker R, Sauer U, Cann I. Bacterial glycogen provides short-term benefits in changing environments. Appl Environ Microb. 2020;86(9):e00049–20. doi:10.1128/AEM.00049-20.
- Cassani L, Gomez-Zavaglia A, Simal-Gandara J. Technological strategies ensuring the safe arrival of beneficial microorganisms to the gut: From food processing and storage to their passage through the gastrointestinal tract. Food Res Int. 2020;129:108852. doi:10.1016/j.foodres.2019.108852.
- Merritt ME, Donaldson JR. Effect of bile salts on the DNA and membrane integrity of enteric bacteria. J Med Microbiol. 2009;58(12):1533–1541. doi:10.1099/jmm.0.014092-0.
- Finkel SE. Long-term survival during stationary phase: evolution and the GASP phenotype. Nat Rev Microbiol. 2006;4(2):113–120. doi:10.1038/nrmicro1340.
- Kaczmarek JL, Thompson SV, Holscher HD. Complex interactions of circadian rhythms, eating behaviors, and the gastrointestinal microbiota and their potential impact on health. Nutr Rev. 2017;75(9):673–682. doi:10.1093/nutrit/nux036.
- Koch AL. The adaptive responses of Escherichia coli to a feast and famine existence. Adv Microb Physiol. 1971;6:147–217.
- Donaldson GP, Lee SM, Mazmanian SK. Gut biogeography of the bacterial microbiota. Nat Rev Microbiol. 2016;14(1):20–32. doi:10.1038/nrmicro3552.
- De Paepe M, Gaboriau-Routhiau V, Rainteau D, Rakotobe S, Taddei F, Cerf-Bensussan N, Casadesús J. Trade-off between bile resistance and nutritional competence drives Escherichia coli diversification in the mouse gut. PLoS Genet. 2011;7(6):e1002107–e. doi:10.1371/journal.pgen.1002107.
- Strange RE. Bacterial “glycogen” and survival. Nature. 1968;220(5167):606–607. doi:10.1038/220606a0.
- Zartl B, Silberbauer K, Loeppert R, Viernstein H, Praznik W, Mueller M. Fermentation of non-digestible raffinose family oligosaccharides and galactomannans by probiotics. Food Funct. 2018;9(3):1638–1646. doi:10.1039/C7FO01887H.
- Hernandez MA, Mohn WW, Martinez E, Rost E, Alvarez AF, Alvarez HM. Biosynthesis of storage compounds by Rhodococcus jostii RHA1 and global identification of genes involved in their metabolism. Bmc Genom. 2008;9(1):600. doi:10.1186/1471-2164-9-600.
- Olga Z, Alexander Z, Kathleen R, Trissa B, John A. Host stress and virulence expression in intestinal pathogens: development of therapeutic strategies using mice and C. elegans. Curr Pharm Des. 2011;17(13):1254–1260. doi:10.2174/138161211795703771.
- Reese AT, Pereira FC, Schintlmeister A, Berry D, Wagner M, Hale LP, Wu A, Jiang S, Durand HK, Zhou X, et al. Microbial nitrogen limitation in the mammalian large intestine. Nature Microbiology. 2018;3(12):1441–1450. doi:10.1038/s41564-018-0267-7.
- Holmes AJ, Chew YV, Colakoglu F, Cliff JB, Klaassens E, Read MN, Solon-Biet SM, McMahon AC, Cogger VC, Ruohonen K, et al. Diet-microbiome interactions in health are controlled by intestinal nitrogen source constraints. Cell Metab. 2017;25(1):140–151. doi:10.1016/j.cmet.2016.10.021.
- Eydallin G, Viale AM, Moran-Zorzano MT, Munoz FJ, Montero M, Baroja-Fernandez E, Pozueta-Romero J. Genome-wide screening of genes affecting glycogen metabolism in Escherichia coli K-12. FEBS Lett. 2007;581(16):2947–2953. doi:10.1016/j.febslet.2007.05.044.
- Vinella D, Albrecht C, Cashel M, D’Ari R. Iron limitation induces SpoT-dependent accumulation of ppGpp in Escherichia coli. Mol Microbiol. 2005;56(4):958–970. doi:10.1111/j.1365-2958.2005.04601.x.
- Lanigan N, Bottacini F, Casey PG, O’Connell Motherway M, van Sinderen D. Genome-wide search for genes required for bifidobacterial growth under iron-limitation. Front Microbiol. 2017;8:964. doi:10.3389/fmicb.2017.00964.
- Jozefczuk S, Klie S, Catchpole G, Szymanski J, Cuadros-Inostroza A, Steinhauser D, Selbig J, Willmitzer L. Metabolomic and transcriptomic stress response of Escherichia coli. Mol Syst Biol. 2010;6(1):364. doi:10.1038/msb.2010.18.
- Svensater G, Bjornsson O, Hamilton IR. Effect of carbon starvation and proteolytic activity on stationary-phase acid tolerance of Streptococcus mutans. Microbiology. 2001;147(Pt 11):2971–2979. doi:10.1099/00221287-147-11-2971.
- Buchanan R, Doyle MP. Foodborne disease significance of Escherichia coli O157: H7 and other enterohemorrhagic E. coli. Food Technol. 1997;51:69–76.
- Hamilton IR, Buckley ND. Adaptation by Streptococcus mutans to acid tolerance. Oral Microbiol Immunol. 1991;6(2):65–71. doi:10.1111/j.1399-302X.1991.tb00453.x.
- Papadimitriou K, Alegria A, Bron PA, de Angelis M, Gobbetti M, Kleerebezem M, Lemos JA, Linares DM, Ross P, Stanton C, et al. Stress physiology of lactic acid bacteria. Microbiol Mol Biol Rev. 2016;80(3):837–890. doi:10.1128/MMBR.00076-15.
- Baucheron S, Nishino K, Monchaux I, Canepa S, Maurel M-C, Coste F, Roussel A, Cloeckaert A, Giraud E. Bile-mediated activation of the acrAB and tolC multidrug efflux genes occurs mainly through transcriptional derepression of ramA in Salmonella enterica serovar Typhimurium. J Antimicrob Chemother. 2014;69(9):2400–2406. doi:10.1093/jac/dku140.
- Seibold GM, Hagmann CT, Schietzel M, Emer D, Auchter M, Schreiner J, Eikmanns BJ. The transcriptional regulators RamA and RamB are involved in the regulation of glycogen synthesis in Corynebacterium glutamicum. Microbiology. 2010;156(4):1256–1263. doi:10.1099/mic.0.036756-0.
- Buret AG, Motta J-P, Allain T, Ferraz J, Wallace JL. Pathobiont release from dysbiotic gut microbiota biofilms in intestinal inflammatory diseases: a role for iron? J Biomed Sci. 2019;26(1):1. doi:10.1186/s12929-018-0495-4.
- Jackson DW, Suzuki K, Oakford L, Simecka JW, Hart ME, Romeo T. Biofilm Formation and Dispersal under the Influence of the Global Regulator CsrA of Escherichia coli. J Bacteriol. 2002;184(1):290. doi:10.1128/JB.184.1.290-301.2002.
- Montanez ND, Carreno H, Escobar P, Estupinan HA, Pena DY, Goel S, Endrino JL. Functional evaluation and testing of a newly developed teleost’s fish otolith derived biocomposite coating for healthcare. Sci Rep. 2020;10(1):258. doi:10.1038/s41598-019-57128-w.
- Kelly SM, Lanigan N, O’Neill IJ, Bottacini F, Lugli GA, Viappiani A, Turroni F, Ventura M, van Sinderen D. Bifidobacterial biofilm formation is a multifactorial adaptive phenomenon in response to bile exposure. Sci Rep. 2020;10(1):11598. doi:10.1038/s41598-020-68179-9.
- Rahimpour M, Montero M, Almagro G, Viale Alejandro M, Sevilla Á, Cánovas M, Muñoz F, Baroja-Fernández E, Bahaji A, Eydallin G, et al. GlgS, described previously as a glycogen synthesis control protein, negatively regulates motility and biofilm formation in Escherichia coli. Biochem J. 2013;452(3):559–573. doi:10.1042/BJ20130154.
- Lagkouvardos I, Pukall R, Abt B, Foesel BU, Meier-Kolthoff JP, Kumar N, Bresciani A, Martínez I, Just S, Ziegler C, et al. The Mouse Intestinal Bacterial Collection (miBC) provides host-specific insight into cultured diversity and functional potential of the gut microbiota. Nature Microbiology. 2016;1(10):16131. doi:10.1038/nmicrobiol.2016.131.
- Wu M, McNulty NP, Rodionov DA, Khoroshkin MS, Griffin NW, Cheng J, Latreille P, Kerstetter RA, Terrapon N; Henrissat B et al. Genetic determinants of in vivo fitness and diet responsiveness in multiple human gut Bacteroides. Science. 2015;350(6256):aac5992.
- Mann E, Wetzels SU, Wagner M, Zebeli Q, Schmitz-Esser S. Metatranscriptome sequencing reveals insights into the gene expression and functional potential of rumen wall bacteria. Front Microbiol. 2018;9:43. doi:10.3389/fmicb.2018.00043.
- Esteban-Torres M, Santamaria L, Cabrera-Rubio R, Plaza-Vinuesa L, Crispie F, de Las Rivas B, Cotter P, Muñoz R. A diverse range of human gut bacteria have the potential to metabolize the dietary component gallic acid. Appl Environ Microbiol. 2018;84(19). doi:10.1128/AEM.01558-18.
- Walker AW, Duncan SH, Louis P, Flint HJ. Phylogeny, culturing, and metagenomics of the human gut microbiota. Trends Microbiol. 2014;22(5):267–274. doi:10.1016/j.tim.2014.03.001.
- van der Maarel MJ, Leemhuis H, van der Maarel MJEC. Starch modification with microbial alpha-glucanotransferase enzymes. Carbohydr Polym. 2013;93(1):116–121. doi:10.1016/j.carbpol.2012.01.065.
- Lee S, Cantarel B, Henrissat B, Gevers D, Birren BW, Huttenhower C, Ko G. Gene-targeted metagenomic analysis of glucan-branching enzyme gene profiles among human and animal fecal microbiota. Isme J. 2014;8(3):493–503. doi:10.1038/ismej.2013.167.
- Ranjan R, Rani A, Finn PW, Perkins DL. Multiomic strategies reveal diversity and important functional aspects of human gut microbiome. Biomed Res Int. 2018;2018:1–13. doi:10.1155/2018/6074918.
- Abu-Ali GS, Mehta RS, Lloyd-Price J, Mallick H, Branck T, Ivey KL, Drew DA, DuLong C, Rimm E, Izard J, et al. Metatranscriptome of human faecal microbial communities in a cohort of adult men. Nature Microbiology. 2018;3(3):356–366. doi:10.1038/s41564-017-0084-4.
- Gosalbes MJ, Compte J, Moriano-Gutierrez S, Valles Y, Jimenez-Hernandez N, Pons X, Artacho A, Francino MP. Metabolic adaptation in the human gut microbiota during pregnancy and the first year of life. EBioMedicine. 2019;39:497–509. doi:10.1016/j.ebiom.2018.10.071.
- McMeechan A, Lovell MA, Cogan TA, Marston KL, Humphrey TJ, Barrow PA. Glycogen production by different Salmonella enterica serotypes: contribution of functional glgC to virulence, intestinal colonization and environmental survival. Microbiology. 2005;151(12):3969–3977. doi:10.1099/mic.0.28292-0.
- Janoir C, Deneve C, Bouttier S, Barbut F, Hoys S, Caleechum L, Chapetón-Montes D, Pereira FC, Henriques AO, Collignon A, et al. Adaptive strategies and pathogenesis of Clostridium difficile from in vivo transcriptomics. Infect Immun. 2013;81(10):3757–3769. doi:10.1128/IAI.00515-13.
- Hasan MK. Role of glycogen and cellobiose PTS operon in clostridiodes difficile virulence and pathogenesis. Kansas State University; 2019.
- Browne HP, Forster SC, Anonye BO, Kumar N, Neville BA, Stares MD, Goulding D, Lawley TD. Culturing of ‘unculturable’ human microbiota reveals novel taxa and extensive sporulation. Nature. 2016;533(7604):543–546. doi:10.1038/nature17645.
- Dietzler DN, Leckie MP, Lais CJ, Henry DA, Rothert JH, Ferguson RM. Periodic inventory review as a strategy for survival in Escherichia coli. The observation of precisely timed, rapid, and simultaneous shifts in glycogen synthesis and glucose utilization in the absence of an external stimulus during prolonged nitrogen starvation. J Biol Chem. 1979;254:8288–8294.