Abstract
The combination of the unique optical properties of chalcogenide glasses, in terms of infrared transmission and optical non-linearity, with the original guiding properties of microstructured optical fibers leads to a new category of fibers with promising applications in mid-infrared optics. The recent developments on chalcogenide microstructured optical fibers are exposed and discussed with regards to mid-IR guiding, infrared light transport and delivery, generation of new infrared sources, and infrared spectroscopy.
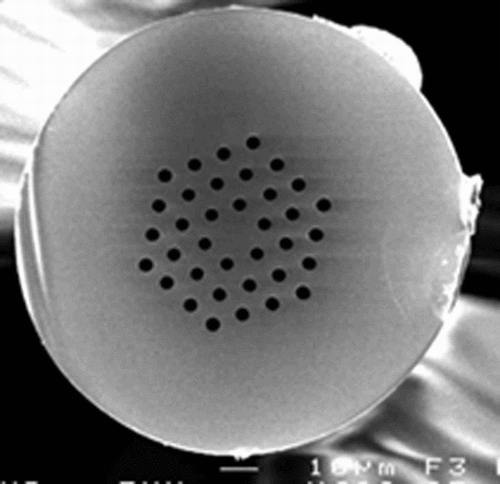
Introduction
In recent years, a growing interest has settled for optical materials and devices for the mid infrared (mid-IR) region, which corresponds to the 2–20 μm electromagnetic spectral range. This interest originates from societal needs for health and environment for instance, and also from demand for defence applications. Indeed, the mid-IR spectral region contains the atmospheric transparent windows (3–5 μm) and (8–12 μm) where thermal imaging – military and civilian – can take place. Furthermore, the infrared window is well-suited for sensing (bio)-molecules, whose fingerprints are located at wavelengths between 2 and 15 μm. In this context, the development of mid-IR transparent materials and optical fibers is essential. Chalcogenide glasses are good candidates for the realization of new and innovative mid-IR systems. Chalcogenide glasses contain at least one chalcogen elements such as S, Se and Te. Depending on the composition, chalcogenide glasses can be transparent from 0·6–2 μm to 12–25 μm [Citation1]. In the present paper, different designs of chalcogenide fibers are studied, including microstructured optical fibers (MOFs) that combine the unique optical properties of chalcogenide glasses in the mid-IR with the original optical guiding properties of such fibers. Different examples are described and discussed: fibers for mid-IR guiding, fibers for spectroscopy, and fibers for enhancing the nonlinear optical properties.
Mid-IR fibers can be classified in two different categories. The first category concerns passive fibers that are used to transmit the infrared information, particularly for infrared spectroscopy. The second category concerns active fibers that produce mid-IR light thanks to rare-earth doping or to non-linear effects (Figure ). In this review article, the interest of chalcogenide MOFs is exposed in those two fields of applications.
Fiber designs for mid-infrared-guiding
In the visible and in the near infrared spectral regions, silica fiber technologies permit the realization of deported systems for imaging or spectroscopy. In the mid-infrared, these technologies do not exist. As illustrated in Figure , for wavelengths longer than 5 μm, transmission of light can be only obtained with hollow core fibers [Citation2], crystalline silver halide fibers [Citation3,4] and chalcogenide glass fibers. Complex structures, like those of microstructured optical fibers [Citation5,6] can be achieved exclusively by using glassy materials. Among glass fibers, only chalcogenide fibers are transparent beyond 5 μm and, therefore, can be used for the transmission of infrared data and for the deported detection of molecules through their IR fingerprints.
The first chalcogenide MOF, based on Ga–La–S, was made in year 2000, but no light propagation was demonstrated at that time [Citation7]. A few years later, light guidance was obtained in sulfur and selenide chalcogenide MOFs [Citation8,9]. Soon after, single mode propagation was demonstrated in Ge–Ga–Sb–S MOF presenting three rings of holes [Citation10]. The optical losses of this first single-mode fiber reached 15–20 dB m−1 at 1·55 μm, due to the fabrication method [Citation11]. The “Stack and Draw” procedure, which has been used successfully to prepare silica MOF, turned out to be not suited for chalcogenide glasses [Citation11]. So, the main challenge in the 2000s was to obtain low-loss chalcogenide MOFs. A new technique, based on an “in-tube” casting of the glass preform, was implemented. This new technique permits to obtain fibers with losses less than 1 dB m−1 [Citation12], quite similar to the glass losses before processing. At the beginning, all chalcogenide MOFs were solid-core MOFs. The first 6-rings of holes, hollow-core fiber, obtained in 2010 did not permitted any light propagation [Citation13]. Later, A. Kosolapov et al. [Citation14], and R. Gattas et al. [Citation15] demonstrated light guidance beyond 10 μm through a Te–As–Se glass hollow-core fiber, and through an As–S hollow-core fiber respectively.
Provided that the d/Λ ratio be smaller than 0·42 (d is the holes diameter, and Λ is the distance between two holes) and that the number of rings be large enough, the second mode is never confined in the core of the fibers [Citation5,16]. As a result, the fiber is single-mode whatever the wavelength. Figure presents the mid-IR transmission of an As38Se62 MOF presenting 3 rings of hole and a core diameter of around 13 μm. With this geometry (d = 3·4 μm, Λ = 8·6 μm and d/Λ = 0·41), from an experimental point of view, this fiber is single mode whatever the wavelength, that is, in this example, from 3 to 10 μm, which corresponds to the transparency domain of the fiber. The absorption peak at 4·5 μm is the signature of Se–H chemical bonds, due to the presence Hydrogen impurities in the glass fiber.
Figure 2. Mid-IR attenuation of an As38Se62 microctrutured optical fiber presenting an endlessly single mode behavior. Inset: cross section of the fiber.
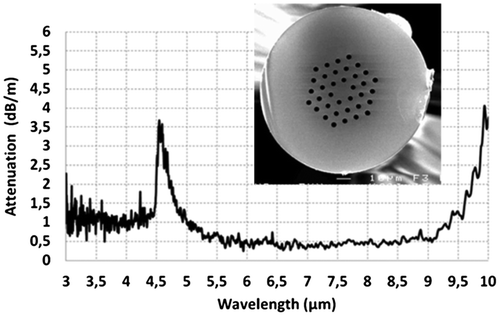
Microstructured optical fibers provide also new solutions for obtaining strong birefringence. Thus, high birefringence can be achieved by designing an asymmetric arrangement of air holes, where holes with two different diameters are placed along the orthogonal axes near the core region [Citation17]. This modification of the fiber symmetry results in an increase of the effective refractive index difference between the two orthogonal polarization modes. An asymmetric structure can be obtained in MOFs not only by altering the air holes size near the core area [Citation17], but also by changing the shape of air holes [Citation18], or by introducing mechanical stress as demonstrated in [Citation19]. It has been shown that the birefringence of silica polarization-maintaining microstructured optical fibers (PM-MOFs) reaches values of the order of 10−3 (1·4 10−3 at 1·55 μm in [Citation20]) which is one order of magnitude higher than that of PANDA or bow-tie silica fibers. Their birefringence is due to stress in the core region, and their modal birefringence is ~5 × 10−4 [Citation21]. In [Citation22], a chalcogenide PM-MOF operating in the 3–8·5 μm infrared window has been investigated. The obtained fiber has an outer diameter of 125 μm and a central solid core of 9 μm. Figure shows a scanning electron microscope image of the fiber. Holes surrounding the core have a pitch of 7·64 μm and diameters of 3·46 μm for the small holes and 6·43 μm for the large ones. This geometry permits to obtain a single-mode polarization-maintaining MOF covering the mid-IR region from 3 to 8·5 μm. As example, the birefringence of the fiber presented in Figure , is 1·5 10−3 at 7·55 μm [Citation22].
Figure 3. Cross section of a highly birefringent chalcogenide microstrudtured optical fibers [Citation22].
![Figure 3. Cross section of a highly birefringent chalcogenide microstrudtured optical fibers [Citation22].](/cms/asset/68ecb5f7-02f9-4ed1-ba48-3c69e4d64149/yadv_a_1312053_f0003_b.gif)
Fiber designs for enhancing the non-linear optical properties
At the telecom wavelength
The use of nonlinear effects has proved to be a promising technique to achieve all-optical fast processing of signals, such as data regeneration, time-domain demultiplexing or wavelength conversion. Due to their high nonlinear refractive index, and fast response time, chalcogenide glasses are one of the most favorite choices to fabricate highly nonlinear components. These nonlinear properties can be further enhanced by taking advantage of the suitability of MOFs for the design of fibers with a very small core diameter. The nonlinear parameter γ = 2πn2/λAeff, (n2 is the nonlinear refractive index, Aeff is the effective area of the mode and λ is the wavelength) permits to compare the non-linear character of the different fibers. Thus, the nonlinear parameter is equal to 70 W−1 km−1 in silica MOF [Citation23] while in chalcogenide MOF it can be as large as 46 000 W−1 km−1 [Citation24] (Figure (b)). The first designs of chalcogenide MOF developed for enhancing the nonlinear properties were suspended-core fibers and tapered suspended-core fibers (Figures (a) and (b)). Tapered fibers permit to reach very small effective area and, consequently, high non-linear parameters, while maintaining good experimental conditions for light injection in the non-tapered zone.
Figure 4. Examples of designs of chalcogenide MOF for telecom applications: (a) As–Se suspended core fiber adapted from [Citation25], (b) tapered As–Se suspended core fiber adapted from [Citation24], (c) Ge–As–Se, small core fiber adapted from [Citation26], (d) tapered Ge–As–Se small core fiber adapted from [Citation27].
![Figure 4. Examples of designs of chalcogenide MOF for telecom applications: (a) As–Se suspended core fiber adapted from [Citation25], (b) tapered As–Se suspended core fiber adapted from [Citation24], (c) Ge–As–Se, small core fiber adapted from [Citation26], (d) tapered Ge–As–Se small core fiber adapted from [Citation27].](/cms/asset/8bf3c2a3-5eeb-4549-bd1b-811a8200928c/yadv_a_1312053_f0004_oc.gif)
In those highly non-linear chalcogenide fibers, numerous non-linear effects have been obtained. For example, self-phase modulation (SPM) broadening has been observed [Citation28,29]. Also, a Mamyshef regenerator has been investigated in an As2S3 suspended-core MOF [Citation30,31]. A transfer function of the intensity of the Mamyshef regenerator was obtained, but the average power needed to observe all-optical regeneration was much higher than the damage threshold of the fiber. Concerning wavelength conversion, others studies have investigated four wave mixing (FWM) effects [Citation24,29,32]. In this case, both pulsed pump (λ1) and CW source (λ2) were injected in a chalcogenide MOF. At the output of the fibers, new wavelengths (λ3 and λ4) appeared in symmetrical pairs. The conversion bandwidth that can be obtained depends strongly on the dispersion multiplied by the length of the device [Citation32]. So, for broad wavelength conversion by FWM, compromises between the linear coefficient, the chromatic dispersion and the fiber length have to be considered. In addition, it has been shown that the propagation in suspended-core fibers was not strictly single mode at the telecom wavelengths. So, for conversion of telecom signal, new small-core fibers have been developed in the Ge–As–Se system presenting 3 rings of hole and a single mode behavior at 1·55 μm (Figures (c) and (d)). This approach permitted wavelength conversion of a 42·7 Gbit s−1 Return to Zero (RZ) 33% signal in a 1-m long Ge–As–Se MOF (Figure (d)) with a total average power of 56 mW only [Citation33]. Wavelength conversion has been also demonstrated in a chalcogenide chip, with a total power of 400 mW [Citation34]. This fiber design, shown in Figure (d), allows also all-optical wavelength conversion and time-domain demultiplexing with a 170-Gb s−1 rate [Citation27].
Stimulated Brillouin scattering (SBS) in optical fibers are important nonlinear interactions that can be exploited to obtain wavelength conversion and highly coherent lasers, specifically in chalcogenide fibers where the Brillouin gain (gB) can reach more than 120 times the gain measured in a silica fiber. Thus, gB is as high as 5·5 10−9 m W−1 and 6·0 10−9 m W−1 in chalcogenide MOF and step index fibers (SIFs), respectively [Citation35,36]. Only the Brillouin thresholds are significantly different. Indeed, the Brillouin threshold reach 85 mW in a 4·9 m-long SIF [Citation35], while a threshold of only 6 mW were observed in only 3-m long MOF [Citation36] (In that study the fiber design was close to the design illustrated in Figure (c)). The single-frequency Brillouin laser made with this fiber showed a promising frequency noise reduction of nearly 6 dB, as compared to the noise of the pump laser. It can be noticed that the Brillouin shift is slightly composition-dependent, with values of 8·2 GHz in a sulfur fiber [Citation28] and 7·95 GHz in As–Se fibers (SIF or MOF) [Citation35,36].
In the mid-infrared region
Applications in the mid-IR region are among the most promising applications for chalcogenide MOFs due to their high nonlinearity and their broadband transmission in the infrared. Numerous simulations and computing studies have shown the high potential of such glassy matrices [Citation37–39]. These simulations show that it can be possible to generate light at wavelengths greater than 12 μm in the infrared. Several experimental studies have demonstrated the generation of supercontinum in chalcogenide MTIR-MOF by using various pump lasers, as shown in Figure . The first experimental studies of such wavelength conversion have been performed with pumping sources between 1·55 μm [Citation30] and 2·5 μm [Citation40]. But, to observe an efficient and broad supercontinuum, the pump wavelength has to be close to the zero-dispersion wavelength (ZDW) of the fiber. However, the intrinsic ZDWs of chalcogenide bulk glasses are located far in the mid-IR, at 4·8 μm and 7·2 μm for As2S3 and As2Se3, respectively. Thanks to the realization of specific MOF designs, the total chromatic dispersion can be shifted to shorter wavelengths [Citation30,41–45]. As an example, for an As2S3 suspended-core fiber, the ZDW could be fixed at 2 μm, with a fiber core-diameter of around 2·6 μm [Citation30]. To obtain the same ZDW in a As2Se3 fiber, the core size must be close to 1 μm. With such small core-diameters, reasonable conditions of light injection into the fiber necessitates the use of tapered geometries in the active zone [Citation46]. However, even with this design, by pumping at a wavelength of 2 μm with pulse duration of 4 ps, the spectral broadening still remains in the 1·3–2·6 μm range. This is mainly due to the strong absorption of the short wavelengths generated below 1·3 μm and to the damage threshold of a so small fiber core (1 μm of diameter).
Figure 5. Mid-IR supercontinum generated in chalcogenide microstructured optical fibers, (a) in a As–S suspended core fiber pumped at 1·5 μm, [Citation30], (b) in the same fiber pumped at 2·5 μm [Citation40], (c) in a As–Se suspended core fiber pumped at 4·7 μm, [Citation49].
![Figure 5. Mid-IR supercontinum generated in chalcogenide microstructured optical fibers, (a) in a As–S suspended core fiber pumped at 1·5 μm, [Citation30], (b) in the same fiber pumped at 2·5 μm [Citation40], (c) in a As–Se suspended core fiber pumped at 4·7 μm, [Citation49].](/cms/asset/18694aad-1f3b-4bca-96cb-c678056541d3/yadv_a_1312053_f0005_oc.gif)
In order to obtain generation of broader supercontinuum in chalcogenide fiber, experiments using longer pump wavelengths have been implemented. With longer pump wavelengths, it is possible to work with chalcogenide fibers presenting higher ZDWs and larger cores, which, in turn, permits to inject more power in the fiber and observe stronger non-linear effects. In step-index fibers (SIF) several examples of generation of supercontinuum have been reported. The most significant results have been obtained by pumping a 8·5-cm long Ge–As–Se fiber, with a 100-fs laser centered at 6·3 μm, which generates a supercontinuum that extends from 1·4 to 13 μm [Citation47], and by pumping at 9·5 μm in a 3-cm As–Se fiber, which leads to a 2–15 μm supercontinuum [Citation48].
In chalcogenide MOFs, the most powerful supercontinuum demonstrated beyond 5 μm was obtained by pumping in the 3·5–4·7 μm range. For example, by pumping at 4·7 μm in a 18-cm long As-Se suspended core fiber, with a peak power of 5·2 kW, a supercontinuum has been observed from 1·7 to 8 μm with an average output power of 15·6 mw [Citation49].
Fibers designs for spectroscopy
Chalcogenide fibers have been successfully implemented in fiber evanescent-wave spectroscopy experiments, for the detection of biochemical molecules in various fields of applications including water pollution [Citation50,51], microbiology, and medicine [Citation52,53]. It has been shown also that the infrared signature of gases embedded in the holes of a chalcogenide MOF can be detected. Indeed, the signature of CO2 gas has been observed at 4·26 μm, by means of a 36-hole As2S3 MOF filled with pure CO2 [Citation54]. However, in this configuration, filling the chemical species into the fiber holes is a cumbersome experiment. Then, a new configuration of microstructured fibers has been developed: exposed-core fibers [Citation55]. This design, which was proposed for the first time by Y. L. Hoo and al. in 2003, consists of an optical fiber with a suspended micron-scale core that is partially exposed to the external environment [Citation56]. This designs has been also realized in chalcogenide fibers [Citation57]. This configuration has permitted to detect infrared signatures of chemical species that are in contact with the exposed core of the fiber. Figure gives infrared signatures of acetone molecules recorded with a 110-μm diameter single-index fiber and a chalcogenide MTIR-MOF presenting a core diameter of 15 μm (with an outer diameter of 220 μm). The results, obtained for similar lengths of fiber interacting with acetone, show that the microstructured exposed-core (MEC) fiber is significantly more sensitive than the single-index fiber. This is essentially because evanescent wave absorption is inversely proportional to the fiber diameter.
Figure 6. Mid-IR detection of acetone using microstrutured exposed-core (MEC) fiber, inset (MEC fiber cross section), adapted from [Citation57].
![Figure 6. Mid-IR detection of acetone using microstrutured exposed-core (MEC) fiber, inset (MEC fiber cross section), adapted from [Citation57].](/cms/asset/ac84ca28-9c34-4d97-b6dc-71477df03904/yadv_a_1312053_f0006_oc.gif)
Conclusion
Since the first realization of a chalcogenide microstructured optical fiber in year 2000, strong improvements have been obtained: regular and controlled geometries, low optical losses, single mode propagation and demonstration of highly nonlinear fibers. These improvements open new investigations in numerous fields of applications like mid-IR wavelength delivery and mid-IR spectroscopy (biomedical, industry and environment domains). Strong non-linear effects have been observed in such fibers, which permitted to realize high-speed wavelength conversion and generation of supercontinuum in the mid-IR spectral range. In addition, the broad infrared transmission of chalcogenide fibers permits the realization of infrared sensors. The high sensitivity of exposed-core microstructured fibers has been demonstrated for the detection of acetone by evanescent wave spectroscopy.
Disclosure statement
No potential conflict of interest was reported by the authors.
References
- Shiryaev V, Churbanov M. Preparation of high purity chalcogenide glasses. In: Zhang XH, Adam JL, editors. Chalcogenide glasses, preparation, properties and applications. Oxford: Woodhead Publishing; 2014. p. 3–35.
- Temelkuran B, Hart SD, Benoit G, et al. Wavelength-scalable hollow optical fibres with large photonic bandgaps for CO2 laser transmission. Nature. 2002;420:650–653.10.1038/nature01275
- Butvina LN, Sereda OV, Dianov EM, et al. Single-mode microstructured optical fiber for the middle infrared. Opt Lett. 2007;32:334–336.10.1364/OL.32.000334
- Shalem S, Tsun A, Rave E, et al. Silver halide single-mode fibers for the middle infrared. Appl Phys Lett. 2005;87. doi:http://dx.doi.org/10.1063/1.2034102.
- Birks TA, Knight JC, Russell PS. Endlessly single-mode photonic crystal fiber. Opt Lett. 1997;22:961–963.10.1364/OL.22.000961
- Birks TA, Roberts PJ, Russell PSJ, et al. Full 2-D photonic bandgaps in silica/air structures. Electron Lett. 1995;31:1941–1943.10.1049/el:19951306
- Monro TM, West YD, Hewak DW, et al. Chalcogenide holey fibres. Electron Lett. 2000;36:1998–2000.10.1049/el:20001394
- Le Person J, Smektala F, Chartier T, et al. Light guidance in new chalcogenide holey fibres from GeGaSbS glass. Mater Res Bull. 2006;41:1303–1309.10.1016/j.materresbull.2006.01.007
- Sanghera JS, Aggarwal ID, Shaw LB, et al. Nonlinear properties of chalcogenide glass fibers. J Optoelectron Adv Mater. 2006;8:2148–2155.
- Brilland L, Smektala F, Renversez G, et al. Fabrication of complex structures of Holey fibers in chalcogenide glass. Opt Express. 2006;14:1280–1285.10.1364/OE.14.001280
- Brilland L, Troles J, Houizot P, et al. Interfaces impact on the transmission of chalcogenide photonic crystal fibres. J Ceram Soc Jpn. 2008;116: Epub 1027.
- Coulombier Q, Brilland L, Houizot P, et al. Casting method for producing low-loss chalcogenide microstructured optical fibers. Opt Express. 2010;18:9107–9112.10.1364/OE.18.009107
- Désévédavy F, Renversez G, Troles J, et al. Chalcogenide glass hollow core photonic crystal fibers. Opt Mater. 2010;32:1532–1539.10.1016/j.optmat.2010.06.016
- Kosolapov AF, Pryamikov AD, Biriukov AS, et al. Demonstration of CO2-laser power delivery through chalcogenide-glass fiber with negative-curvature hollow core. Opt Express. 2011;19:25723–25728.10.1364/OE.19.025723
- Gattass RR, Rhonehouse D, Gibson D, et al. Infrared glass-based negative-curvature anti-resonant fibers fabricated through extrusion. Opt Express. 2016;24:25697–25703.10.1364/OE.24.025697
- Renversez G, Bordas F, Kuhlmey BT. Second mode transition in microstructured optical fibers: determination of the critical geometrical parameter and study of the matrix refractive index and effects of cladding size. Opt Lett. 2005;30:1264–1266.10.1364/OL.30.001264
- Ortigosa-Blanch A, Knight JC, Wadsworth WJ, et al. Highly birefringent photonic crystal fibers. Opt Lett. 2000;25:1325–1327.10.1364/OL.25.001325
- Yue Y, Kai G, Wang Z, et al. Highly birefringent elliptical-hole photonic crystal fiber with squeezed hexagonal lattice. Opt Lett. 2007;32:469–471.10.1364/OL.32.000469
- Schreiber T, Röser F, Schmidt O, et al. Stress-induced single-polarization single-transverse mode photonic crystal fiber with low nonlinearity. Opt Express. 2005;13:7621–7630.10.1364/OPEX.13.007621
- Suzuki K, Kubota H, Kawanishi S, et al. Optical properties of a low-loss polarization-maintaining photonic crystal fiber. Opt Express. 2001;9:676–680.10.1364/OE.9.000676
- Noda J, Okamoto K, Sasaki Y. Polarization-maintaining fibers and their applications. J Lightwave Technol. 1986;4:1071–1089.10.1109/JLT.1986.1074847
- Caillaud C, Gilles C, Provino L, et al. Highly birefringent chalcogenide optical fiber for polarization-maintaining in the 3–8.5 μm mid-IR window. Opt Express. 2016;24:7977–7986.10.1364/OE.24.007977
- Lee JH, Belardi W, Furusawa K, et al. Four-wave mixing based 10-Gb/s tunable wavelength conversion using a holey fiber with a high SBS threshold. Photon Technol Lett, IEEE. 2003;15:440–442.
- Le SD, Nguyen DM, Thual M, et al. Efficient four-wave mixing in an ultra-highly nonlinear suspended-core chalcogenide As38Se62 fiber. Opt Express. 2011;19:B653–B60.10.1364/OE.19.00B653
- Troles J, Coulombier Q, Canat G, et al. Low loss microstructured chalcogenide fibers for large non linear effects at 1995 nm. Opt Express. 2010;18:26647–26654.10.1364/OE.18.026647
- Toupin P, Brilland L, Trolès J, et al. Small core Ge-As-Se microstructured optical fiber with single-mode propagation and low optical losses. Opt Mater Express. 2012;2:1359–1366.10.1364/OME.2.001359
- Le SD, Gay M, Bramerie L, et al. All-optical time-domain demultiplexing of 170.8 Gbit/s signal in chalcogenide GeAsSe microstructured fibre. Electron Lett. 2013;49:136–138.10.1049/el.2012.4104
- Fatome J, Fortier C, Nguyen TN, et al. Linear and nonlinear characterizations of chalcogenide photonic crystal fibers. J Lightwave Technol. 2009;27:1707–1715.10.1109/JLT.2009.2021672
- Nguyen DM, Le SD, Lengle K, et al. Demonstration of nonlinear effects in an ultra-highly nonlinear AsSe suspended-core chalcogenide fiber. IEEE Photon Technol Lett. 2010;22:1844–1846.10.1109/LPT.2010.2088386
- El-Amraoui M, Gadret G, Jules JC, et al. Microstructured chalcogenide optical fibers from As2S3 glass: towards new IR broadband sources. Opt Express. 2010;18:26655–26665.10.1364/OE.18.026655
- Mamyshev PV. All-optical data regeneration based on self-phase modulation effect. Optical Communication, 1998 24th European Conference. 1998;1.
- Brès CS, Zlatanovic S, Wiberg AOJ, et al. Continuous-wave four-wave mixing in cm-long chalcogenide microstructured fiber. Opt Express. 2011;19:B621–B7.10.1364/OE.19.00B621
- Le SD, Nguyen DM, Thual M, et al. 42.7 Gbit/s RZ-33% wavelength conversion in a chalcogenide microstructured fiber OFC. Proc. Optical Fiber Communication Conference; 2012; Los Angeles (CA), paper Oth4h.4.
- Pelusi MD, Luan F, Madden S, et al. Wavelength conversion of high-speed phase and intensity modulated signals using a highly nonlinear chalcogenide glass chip. Photon Technol Lett, IEEE. 2010;22:3–5.10.1109/LPT.2009.2035094
- Abedin KS. Observation of strong stimulated Brillouin scattering in single-mode As2Se3 chalcogenide fiber. Opt Express. 2005;13:10266–10271.10.1364/OPEX.13.010266
- Tow KH, Léguillon Y, Fresnel S, et al. Linewidth-narrowing and intensity noise reduction of the 2(nd) order Stokes component of a low threshold Brillouin laser made of Ge10As22Se68 chalcogenide fiber. Opt Express. 2012;20:B104–B9.10.1364/OE.20.00B104
- Dabas B, Sinha RK. Dispersion characteristic of hexagonal and square lattice chalcogenide As2Se3 glass photonic crystal fiber. Opt Commun. 2010;283:1331–1337.10.1016/j.optcom.2009.11.091
- Hu J, Menyuk CR, Shaw LB, et al. Computational study of 3–5 mu m source created by using supercontinuum generation in As2S3 chalcogenide fibers with a pump at 2 mu m. Opt Lett. 2010;35:2907–2909.10.1364/OL.35.002907
- Hu J, Menyuk CR, Shaw LB, et al. Maximizing the bandwidth of supercontinuum generation in As2Se3 chalcogenide fibers. Opt Express. 2010;18:6722–6739.10.1364/OE.18.006722
- Mouawad O, Picot-Clémente J, Amrani F, et al. Multioctave midinfrared supercontinuum generation in suspended-core chalcogenide fibers. Opt Lett. 2014;39:2684–2687.10.1364/OL.39.002684
- Duhant M, Renard W, Canat G, et al. Fourth-order cascaded Raman shift in AsSe chalcogenide suspended-core fiber pumped at 2 μm. Opt Lett. 2011;36:2859–2861.10.1364/OL.36.002859
- Kulkarni OP, Xia C, Lee DJ, et al. Third order cascaded Raman wavelength shifting in chalcogenide fibers and determination of Raman gain coefficient. Opt Express. 2006;14:7924–7930.10.1364/OE.14.007924
- Thielen PA, Shaw LB, Pureza PC, et al. Small-core As-Se fiber for Raman amplification. Opt Lett. 2003;28:1406–1408.10.1364/OL.28.001406
- White RT, Monro TM. Cascaded Raman shifting of high-peak-power nanosecond pulses in As2S3 and As2Se3 optical fibers. Opt Lett. 2011;36:2351–2353.10.1364/OL.36.002351
- Cheng T, Kanou Y, Deng D, et al. Fabrication and characterization of a hybrid four-hole AsSe2-As2S5 microstructured optical fiber with a large refractive index difference. Opt Express. 2014;22:13322–13329.10.1364/OE.22.013322
- Duhant M, Renard W, Canat G, et al. Mid-infrared strong spectral broadening in microstructured tapered chalcogenide AsSe fiber. Proc. SPIE 8237 Fiber Lasers IX: Technology, Systems, and Applications; 2012; San Francisco (CA). p. 823735–823740.
- Petersen CR, Møller U, Kubat I, et al. Mid-infrared supercontinuum covering the 1.4–13.3 μm molecular fingerprint region using ultra-high NA chalcogenide step-index fibre. Nat Photon. 2014;8:830–834.10.1038/nphoton.2014.213
- Deng DH, Liu L, Tuan TH, et al. Mid-infrared supercontinuum covering 3-10 μm using a As2Se3 core and As2S5 cladding step-index chalcogenide fiber. J Ceram Soc Jpn. 2016;124:103–105.10.2109/jcersj2.15203
- Møller U, Yu Y, Kubat I, et al. Multi-milliwatt mid-infrared supercontinuum generation in a suspended core chalcogenide fiber. Opt Express 2015;23:3282–3291.10.1364/OE.23.003282
- Michel K, Bureau B, Pouvreau C, et al. Development of a chalcogenide glass fiber device for in situ pollutant detection. J Non-Cryst Solids. 2003;326–327:434–438.10.1016/S0022-3093(03)00438-1
- Michel K, Bureau B, Boussard-Plédel C, et al. Monitoring of pollutant in waste water by infrared spectroscopy using chalcogenide glass optical fibers. Sens Actuators B: Chem. 2004;101:252–259.10.1016/j.snb.2004.03.014
- Keirsse J, Boussard-Pledel C, Loreal O, et al. Chalcogenide glass fibers used as biosensors. J Non-Cryst Solids. 2003;326–327:430–433.10.1016/S0022-3093(03)00434-4
- Brandily ML, Monbet V, Bureau B, et al. Identification of foodborne pathogens within food matrices by IR spectroscopy. Sens Actuators B: Chem. 2011;160:202–206.10.1016/j.snb.2011.07.034
- Charpentier F, Troles J, Coulombier Q, et al. CO2 detection using microstructured chalcogenide fibers. Sensor Lett. 2009;7:745–749.10.1166/sl.2009.1142
- Warren-Smith SC, Afshar VS, Monro TM. Theoretical study of liquid-immersed exposed-core microstructured optical fibers for sensing. Opt Express. 2008;16:9034–9045.10.1364/OE.16.009034
- Hoo YL, Jin W, Shi C, et al. Design and modeling of a photonic crystal fiber gas sensor. Appl Opt. 2003;42:3509–3515.10.1364/AO.42.003509
- Toupin P, Brilland L, Boussard-Plédel C, et al. Comparison between chalcogenide glass single index and microstructured exposed-core fibers for chemical sensing. J Non-Cryst Solids. 2013;377:217–219.10.1016/j.jnoncrysol.2012.12.026