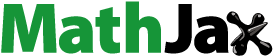
ABSTRACT
Candidiasis caused by Candida albicans infection has long been a serious human health problem. The pathogenicity of C. albicans is mainly due to its virulence factors, which are novel targets of antifungal drugs for low risk of resistance development. In this study, we identified a maleimide compound [1-(4-methoxyphenyl)-1hydro-pyrrole-2,5-dione, MPD] that exerts effective anti-virulence activity. It could inhibit the process of adhesion, filamentation, and biofilm formation in C. albicans. In addition, it exhibited low cytotoxicity, hemolytic activity, and drug resistance development. Moreover, in Galleria mellonella-C. albicans (in vivo) infection model, the survival time of infected larvae was significantly prolonged under the treatment of MPD. Further, mechanism research revealed that MPD increased farnesol secretion by upregulating the expression of Dpp3. The increased farnesol inhibited the activity of Cdc35, which then decreased the intracellular cAMP content resulting in the inhibition of virulence factors via the Ras1-cAMP-Efg1 pathway. In all, this study evaluated the inhibitory effect of MPD on various virulence factors of C. albicans and identified the underlying mechanisms. This suggests a potential application of MPD to overcome fungal infections in clinics.
Introduction
Invasive candidiasis infections have become a worldwide health problem causing Candida bloodstream infections and deep-seated candidiasis. As per reports, there are >750,000 cases of invasive candidiasis causing >50,000 deaths each year worldwide [Citation1,Citation2]. Among them, the opportunistic fungus C. albicans is the main pathogen causing fungemia and disseminated candidiasis [Citation2]. It normally colonizes the skin and mucosa of a healthy human body. However, damage to normal defence functions, such as trauma, nutritional disorders, immune function deficiencies, and the use of hormones or antibiotics disrupt the flora balance and cause C. albicans to overgrow. The abundance of C. albicans causes superficial mucosal infections in oral cavity, throat, and reproductive tract, as well as systemic invasive candidiasis of the circulatory system, bones, and brain [Citation3].
Numerous antifungal drugs are clinically used to deal with invasive candidiasis. Nonetheless, the associated drug resistance and toxic side effects greatly limit their application, thus increasing the complication and cost of fungal infection treatment. Azoles, polyenes, and echinocandins are the most commonly used antifungal drugs [Citation4]. Azoles, owning high bioavailability and low toxic side effects, are the first-line antifungal drugs. However, they are prone to develop drug resistance with the prolongation of medication time, resulting in treatment failure [Citation5]. In contrast, polyenes have a good antifungal effect and do not easily develop resistance, but have large adverse reactions, especially severe nephrotoxicity [Citation6]. Additionally, echinocandins have good safety and clinical efficacy; however, due to their large molecular weight, low oral availability, and limited gastrointestinal absorption, they can only be administered intravenously [Citation7]. Therefore, the discovery of novel antifungal drugs is imminent.
Natural compounds with maleimide ring are secondary metabolites of marine microorganisms. They exhibit hydrophobicity and can easily pass through the cell membrane [Citation8]. They show many bioactivities, such as antibacterial, antifungal, and anticancer activities [Citation9]. Hence, we synthesized 1-(4-methoxyphenyl)-1hydro-pyrrole-2,5-dione (MPD), a maleimide compound and determined its antifungal effects on C. albicans ().
Figure 1. The structure and drug resistance development of MPD. (a) The chemical structure of 1-(4-methoxyphenyl)-1hydro-pyrrole-2,5-dione (MPD). (b) Drug resistance development test of MPD and FLC against C. albicans YEM30 using consecutive treatment of 1/2 MIC of MPD or 1/2 MIC of FLC.

This study found that MPD could effectively inhibit the virulence factors of C. albicans with little cytotoxicity and drug-resistance development. Further mechanism research revealed that this inhibitory effect was attributed to the increased farnesol secretion and decreased cyclic adenosine monophosphate (cAMP) synthesis. In addition, MPD could prolong the survival time of infected larvae in vivo. These findings show that MPD may be a promising candidate drug, which can be used to target the virulence factors of fungi to fight infections related to C. albicans clinically.
Materials and methods
Strains
The Candida isolates used in this research were kindly provided by the microbiology laboratory of Affiliated Hospital of Xuzhou Medical University in China and were stored in the microbic preservation solution (normal saline containing 20% glycerol) at −80°C. Prior to each experiment, strains were re-inoculated twice on the YPD agar medium (1% yeast extract, 2% peptone, 2% dextrose, and 2% agar) at 30°C, followed by inoculating a single colony into the YPD liquid medium (1% yeast extract, 2% tryptone, and 2% dextrose) at 30°C till logarithmic growth period. Galleria mellonella was purchased from Huiyude Biotechnology Co., Ltd., China.
Chemicals
MPD was synthesized by our laboratory, and its purity was higher than 96% by high-performance 61 liquid chromatography. Amphotericin B (AMB) and Fluconazole (FLC) were all purchased from Sigma [Citation10]. The three chemicals were dissolved to 10 mg/mL with dimethyl sulphoxide (DMSO) (Sigma, USA) and stored at −20°C. The content of solvent DMSO was below 1% in each analysis.
Determination of minimum inhibitory concentration (MIC)
The MIC values of MPD were tested using a standardized broth microdilution method. According to the Clinical and Laboratory Standards Institute (CLSI) guidelines (M27-A3) (CLSI, 2008), the minimal dose of no visible growth was considered as the MIC value.
Drug resistance development test
By repeatedly treating C. albicans (YEM30) with antifungal drugs, drug resistance was induced. The MIC value of MPD to wild type C. albicans YEM30 was tested for 40 consecutive days [Citation11]. YEM30 strain was exposed to 0.5 μg/mL (1/2 MIC) of MPD at a specific passage, and then re-grew to the logarithmic growth phase, and was used again to measure the MIC of the subsequent passage with the same antifungal agent. The 0.5 μg/mL FLC treatment group was set as a positive control. The drug resistance of C. albicans was evaluated by recording the change of MIC compared with the original passage.
Inhibition of adhesion
YEM30 strain was adjusted to 1 × 106 cells/mL in RPMI 1640 medium and then incubated with different doses of MPD for 90 min, and FLC (1 μg/mL) was used as the positive control. After washing twice to remove the planktonic cells, the remaining adherent cells were detected by an XTT Cell Proliferation Assay Kit (BestBio, China) and observed in the bright field mode under an Olympus microscope.
Inhibition of hyphae
The RPMI 1640 medium and spider agar medium (0.1% K2HPO4, 0.5% mannitol, 1% nutrient broth, and 2% agar, pH 7.2) were used to detect the effect of MPD on the filamentation of C. albicans as previously described [Citation12,Citation13]. YEM30 strain (1 × 105 cells/mL) were suspended in the RPMI 1640 medium with different doses of MPD treatments (0, 0.25, 0.5, and 1 μg/mL), and then incubated at 37°C. Every 3 h, cells morphology was monitored using an Olympus microscope. In addition, under different treatments of MPD, the cells were also inoculated on the spider agar medium at 37°C. After 48-h incubation, the formed colonies were also imaged by microscopy.
Inhibition of biofilm formation
The effect of MPD on the C. albicans biofilm formation was measured using the XTT reduction assay, microscopy, and scanning electron microscopy (SEM). YEM30 strain under different doses of MPD treatments (0, 0.25, 0.5, and 1 μg/mL) were adjusted in the RPMI 1640 medium to 1 × 106 cells/mL and then incubated at 37°C. After 24 h incubation, the supernatant was removed, and the formed biofilms were washed with deionized water for three times. The XTT reduction assay was used to measure the formation of biofilm, and the Olympus microscope was used to observe the formed biofilm in bright field mode.
In the SEM assay, the prepared YEM30 strain was seeded on poly-L-lysine (Sigma, USA) coated glass coverslip discs (HiMedia, India) in a 12-well flat-bottom plate and statically cultured at 37°C for 24 h. The discs with formed biofilm were removed from the wells and washed with deionized water for three times. After continuous ethanol dehydration (25, 50, 65, 95, and 100% ethanol) treatment for 15 min, the samples were dried in a desiccator and then sputter coated with gold and observed by SEM (FEI Co., Ltd., Hillsboro, OR, USA) at 1 KX and 5 KX magnification [Citation1].
Inhibition of cell surface hydrophobicity (CSH)
As described previously [Citation14], YEM30 strain, prepared as above biofilm formation assay, was seeded into a 24-well flat bottom plate and incubated statically for 24 h at 37°C. The cultured cells were then washed with deionized water for three times and diluted to an OD600 of 1.0. Mix 1.2 mL of cell suspension and 0.3 mL of octane in a clean glass test tube. After a vortex for 3 min and phase separation for 15 min, the OD600 of the aqueous phase was measured by a Bio-Rad Model 680 microplate reader, and the CSH% was calculated as the following formula:
where ODC is the OD600 without added octane and ODE is the OD600 of the experimental group.
Determination of intracellular cAMP level
The intracellular cAMP concentration was measured according to the previous method with some modifications [Citation15]. YEM30 strain treated with different doses of MPD was incubated in RPMI 1640 medium at 37°C. After 12 h incubation, the intracellular cAMP concentration was measured by a cAMP ELISA Kit (Elabscience Biotechnology Co., China).
The cAMP rescue assay
To confirm whether the inhibitory effect of filamentation induced by MPD was due to the change of intracellular cAMP content or not, a cAMP rescue test was carried out. YEM30 strain, prepared as filamentation assay described above, were treated with 0.25, 0.5, and 1 µg/mL of MPD. And then, dibutyryl-cAMP (db-cAMP, Sigma, USA) was added to the MPD treated or untreated groups for a dose of 1 mM, respectively. The db-cAMP-free group was set as a negative control. After the incubation at 37°C for 4 h, the morphology of the cells under different treatments was monitored by the Olympus microscope. In addition, the cAMP rescue test was also carried out on the biofilm formation assay. YEM30 strain was treated as above biofilm formation assay described, and treated with 1 and 2 μg/mL of MPD in the presence of 1 mM db-cAMP. After 24 h incubation at 37°C, the formed biofilm was washed and imaged by microscope, and the XTT reduction assay was used for measurement.
Quantification of farnesol secretion
YEM30 strain was adjusted to 1 × 105 cells/mL in RPMI 1640 medium and treated with different doses of MPD (0, 0.5, 1, and 2 μg/mL) at 37°C for 12 h. After incubation, cells and cultured supernatants were collected, respectively. Weigh the dry weight of cells. The farnesol of the supernatant was extracted with ethyl acetate and quantified by GC/MS system (Agilent, USA) as previously described [Citation16]. The content of farnesol was calculated by the cell dry weight.
Measurement of DPP3 expression
BWP17-DPP3-GFP strain was adjusted to 1 × 105 cells/mL in RPMI 1640 medium and treated with different doses of MPD at 37°C for 4 h. The expression of Dpp3 was reflected by the fluorescence intensity of GFP, which was detected by an Olympus fluorescence microscope and a spectrofluorophotometer (BioTek Co., USA) with 486 nm excitation wavelength and 528 nm emission wavelength [Citation17].
Transcriptional levels of virulence genes
As previously described [Citation16], the quantitative reverse transcription-polymerase chain reaction (qRT-PCR) was utilized to measure the transcriptional levels of tested genes with different MPD treatments. YEM30 strain was diluted in RPMI 1640 medium and treated with 1 μg/mL of MPD at 37°C for 12 h. The cells were then collected by centrifugation and washed with sterile water. The total RNAs were extracted by the hot phenol method [Citation18] and then reverse transcribed into cDNA using a cDNA synthesis kit (TaKaRa, China). Finally, the expression of genes was detected by a SYBR Green master mix kit (TaKaRa, China). The cDNA served as template, 18S rRNA served as the internal reference gene, and the sequences of primers used in this study are shown in .
Table 1. Gene-specific primers used for real-time RT-PCR.
Inhibitory hyphal effect of MPD against DPP3 knockout mutant
As mentioned above, DPP3 knockout mutant (KWN2) was adjusted to 1 × 105 cells/mL in RPMI 1640 medium and treated with different doses of MPD (0 and 1 μg/mL) at 37°C for 12 h. Under control, its parent strain (SN152) was treated in the same manner. Both strains have the ability to form normal hyphae. Every 3 h, the cell morphology was monitored using an Olympus microscope.
Toxicity and hemolytic activity assay
Two normal cell lines and G. mellonella were utilized to evaluate the cytotoxicity of MPD. The effect of MPD on the proliferation of cell-line HT22 and HEK 293T was detected by the MTT assay as previously described [Citation19]. Briefly, the prepared cells were co-cultured with different doses of MPD for 24 h. Then, add MTT into the treated cells and incubate for 4 h in the dark. Discard the supernatant, add 150 µL of DMSO, and shake the plate slowly for 10 min. Transfer the colored solution into a new plate and measure the absorbance of each treatment using a Bio-Rad plate reader at 570 nm. The 50% inhibitory concentration (IC50) was calculated based on the value of OD570.
The survival rate of wild-type G. mellonella under a high dose of MPD treatment was monitored as previously described [Citation20]. The prepared healthy larvae were treated with high doses of MPD (up to eightfolds of MIC) in a plate and then cultured at 37°C for 5 days. The larvae were monitored to calculate the survival rate of different treatments.
The hemolytic assay was performed using human erythrocytes [Citation21]. Briefly, erythrocytes were diluted with normal saline to make a 4% erythrocyte suspension. The erythrocyte suspension was incubated with FLC, AMB, and MPD (0.5, 1, 2, 4, and 8 μg/mL) in a 96-well plate for 1 h, respectively. The Triton X-100 (Sigma, USA) treated and drug-free groups were set as positive and negative control groups. The value of OD414 was measured after incubation by a Bio-Rad 680 microplate reader, and the hemolysis rate was calculated as the following formula:
where ODE, ODNC, and ODPC are the absorbance of the treated group, negative control, and positive control groups at 414 nm, respectively.
Infection model
The G. mellonella infection model was used to evaluate the in vivo activity of MPD in terms of survival and fungal burden. A total of 45 larvae of similar size were selected and randomly allocated to our groups. The last right foreleg of each larva was injected with 10 μL of C. albicans YEM30 suspension (2 × 105 CFU/mL). Two hours after infection, the larvae were injected with 10 μL of drug or vehicle as follows for the three groups: MPD (2 μg per larva), FLC (2 μg per larva), and control (PBS). All larvae are cultured at 37°C in a dark environment. Failure to respond to slight contact is considered larval mortality. In the survival assay, the number of surviving larvae in each group was calculated at the same time every day for 5 days.
To determine the fungal burden, larvae were infected and treated as described above with 40 larvae per group. Six larvae per day were randomly selected from each group and homogenized with glass beads in 0.5 mL sterile PBS. The homogenate was serially diluted with sterile PBS, and 5 μL was spread on a YPD solid plate.
Statistical analysis
The data of infection model was analysed by the log rank test. The difference between control and experimental groups was determined by unpaired, two-tailed Student’s t-test. One-way or two-way ANOVA was used for comparison among multiple groups. All experiments were conducted at least three times, and the values were expressed as mean ± standard deviation (SD). Asterisks represent a statistically significant difference (* means P < 0.05; ** means P < 0.01).
Results
Effects of MPD on the proliferation of C. albicans
We determined the MIC values of MPD on various types of C. albicans to evaluate the effect of MPD on the proliferation. The results showed that MPD on different genotypes of C. albicans had effective antifungal activity (). Notably, MPD displayed the same antifungal activity as wild-type strain in isolates of azole multi-resistant C. albicans CA10 and efflux pump deletion mutant C. albicans strains.
Table 2. The MIC values of MPD against different genotypes of C. albicans.
Effects of MPD on induced drug resistance of C. albicans
Drug resistance has become a feature in most fungal species that infect humans, and this is an emerging clinical antifungal treatment problem [Citation22]. It is found that wild type C. albicans YEM30 developed drug resistance after being treated with 1/2 MIC of FLC for 5 days, and its MIC value of FLC increased from 1 to 8 μg/mL after 30 days. However, even after 40 days of continuous MPD treatment, YEM30 strain did not develop drug resistance ().
Effects of MPD on the adhesion of C. albicans
Adhesion is a necessary condition for C. albicans colonization and the first step in exerting pathogenicity [Citation23]. The results indicated that MPD could reduce the number of C. albicans adhered to polystyrene materials (). Specifically, 0.25 and 0.5 µg/mL of MPD significantly prevented about 15% and 30% of cells, respectively, from adhering to the substratum. At the same dose of MPD (1 µg/mL), the inhibition rate was higher than that of FLC, increasing to 70%. A similar trend was observed by microscopy (). The number of adherent cells in the MPD-treated group was lower than that in the control group. These results suggested that MPD effectively inhibited the adherence to non-biological materials.
MPD inhibits the yeast-to-hyphae transition of C. albicans
Hyphae secrete hydrolases to strengthen the invasion and damage of C. albicans to the host, which is the key virulence factor [Citation24]. We therefore examined the effect of MPD on filamentation under two hyphae-inducing media in vitro. MPD inhibited hyphal formation in both RPMI 1640 and spider mediums (). In RPMI 1640 medium, regular hyphae were formed within 6 h and a complex yeast network was formed within 12 h in the untreated group and the 0.25 μg/mL MPD group. Interestingly, the hyphae of 0.5 µg/mL group were much shorter than those of the control group, while cells in the 1 µg/mL group maintained the yeast form. These results were confirmed by the tests performed on solid Spider media. Specifically, MPD-free and 0.25 µg/mL of MPD groups formed radial colonies, while the 0.5 µg/mL group could partially inhibit the development of radial colonies. In contrast, the edge of the colony formed in 1 µg/mL group was smooth. Overall, MPD could inhibit the transformation of C. albicans from yeast cells to hyphal cells.
MPD suppresses the biofilm formation of C. albicans
Biofilm formation endows C. albicans with strong drug resistance [Citation25]. Therefore, we analysed the effect of MPD on biofilm formation using the XTT reduction assay and microscope. The results indicated that MPD could inhibit the formation of biofilm. At 2 µg/mL of MPD, the inhibition rate of biofilms was up to 85% (). The untreated group formed a biofilm consisting of a mixture of yeast cells and hyphae, as shown by microscopy and SEM. When the MPD concentration was 1 µg/mL, the biofilm structure was partly altered, and the hyphae were shorter than those in the control group. Additionally, in the 2 µg/mL of MPD treatment group, no biofilm was formed and only yeast cell clusters adhered to the bottom (). These findings indicated that MPD could reduce biofilm development in C. albicans by inhibiting the yeast-to-hyphae transition.
Figure 4. MPD suppresses the biofilm formation of C. albicans. YEM30 strain treated with MPD were incubated in RPMI 1640 medium at 37 °C for 24 h. After incubation, an XTT reduction assay (a), the observation with microscopy and SEM (b) were used to detect biofilm formation. (b) The first row of photographs was taken under the optical microscope and the remaining two rows were taken under the SEM. (c) The effect of MPD on the CSH of C. albicans. The bars in (a), (b), and (c) show 100 µm, 100 μm, and 20 μm, respectively. Results in (a) and (c) are shown as means ± SDs.

MPD reduces CSH in C. albicans
CSH is involved in biofilm formation and is important for C. albicans adherence to abiotic and biotic surfaces [Citation26]. Thus, we examined whether MPD has an impact on the CSH of C. albicans. These results indicated that MPD reduced the CSH of C. albicans strongly with the increase in dosage (). At low concentrations (< MIC), the effect on CSH was limited. However, at the doses of 1 and 2 µg/mL of MPD, the CSH was 53% and 10%, respectively. This is similar to the results of microscopic observation of biofilm formation and XTT reduction assay, and suggests that MPD suppresses biofilm formation by reducing CSH in C. albicans.
MPD decreases intracellular cAMP content to inhibit the formation of hyphae and biofilm
Accumulating evidences suggest that cAMP plays an essential role in regulating filamentation in C. albicans. Increased intracellular cAMP promotes hyphal growth [Citation27]. Thus, we examined the effect of MPD on the intracellular cAMP concentrations of C. albicans. The results indicated that starting from 0.5 μg/mL of MPD could significantly decrease intracellular cAMP concentrations (). Moreover, when exogenous db-cAMP was added, the MPD-induced defects of hyphal and biofilm formation were partially restored in the presence of 0.5, 1, or 2 μg/mL of MPD treatment (). Precisely, under 2 μg/mL MPD treatment, the biofilm formation rate increased from 17% to 64% by adding 1 mM cAMP (). These results suggested that reduced intracellular cAMP contributed to the MPD-induced hyphae and biofilm inhibition.
Figure 5. The effect of cAMP on the hyphal and biofilm formation in the presence of MPD treatment. (a) YEM30 strain treated with MPD were incubated in RPMI 1640 medium at 37 °C for 12 h. The intracellular cAMP content was measured by cAMP Elisa kit. (b) YEM30 strain were diluted in RPMI 1640 medium containing different concentrations of MPD, and then added with 1 mM db-Camp. After incubation at 37 °C for 4 h, the imaging was observed by microscopy. The effect of exogenous cAMP on the 1 and 2 μg/mL of MPD induced biofilm inhibition was detected by the XTT reduction assay (c) and microscopy (d). The bars in (b) and (d) show 100 μm. Results in (a) and (c) are shown as means ± SDs.

MPD induces the expression of DPP3 to synthesize farnesol
Yeast-to-hyphae transition is regarded as the key process of C. albicans biofilm formation, which is inhibited by farnesol [Citation28]. Thus, we detected the effect of MPD on farnesol secretion using GC-MS. The results indicated that MPD stimulated the secretion of farnesol in culture supernatant. The 0.5, 1, and 2 µg/mL of MPD treatment increased the farnesol secretion by 3.51, 6.06, and 7.36 times when compared with the MPD-free group ().
Figure 6. The effect of MPD on the farnesol secretion and related genes expression. (a) After farnesol was extracted from the supernatant of YEM30 strain treated with MPD, the content of farnesol was analysed by GC-MS. The fluorescence intensity of BWP17-DPP3-GFP strain was detected to reflect the expression of Dpp3. The intensity of fluorescence was monitored by fluorescence microscopy (b) and spectrofluorophotometry (c). (d) Qrt-PCR was used to detect the expression of hypha-related genes involved in Ras-Camp-Efg1 signalling pathway under MPD treatment. The bar in (b) indicates 50 µm. Results in (a), (c), and (d) are shown as means ± SDs.

The Dpp3 is an important enzyme involved in the conversion of farnesyl pyrophosphate (FPP) into farnesol [Citation29]. According to our previous studies, some natural products can increase the synthesis of farnesol by enhancing the activity of Dpp3 [Citation30]. In this study, a Dpp3 labeled green fluorescent protein strain (BWP17-DPP3-GFP) was utilized to detect the transcriptional level of Dpp3 in the presence of MPD, using the fluorescence intensity of GFP as an indicator. These results indicated that MPD treatment enhanced the fluorescence intensity of Dpp3-gfp (). Specifically, compared with control, 0.5, 1, and 2 μg/mL of MPD caused the increase in Dpp3 expression by 1.66-fold, 2.49-fold, and 2.55-fold, respectively, which was revealed by multifunctional microplate reader detection (). Collectively, the data showed that MPD could induce farnesol synthesis by stimulating the expression of Dpp3.
Effect of MPD on the expression of virulence related genes in C. albicans
Since MPD inhibits different virulence factors of C. albicans, we investigated the potential molecular mechanisms by evaluating the expression of related genes using qRT-PCR. We observed that MPD downregulated the adhesion-related genes ALS3 and HWP1 (). The genes that regulate hyphal formation in the Ras-cAMP-PKA pathway were also down-regulated. The MPD treatment decreased the expression of RAS1, CDC35, EFG1, and TEC1 genes by 0.18-fold, 0.44-fold, 0.29-fold, and 0.36-fold, respectively.
Effect of MPD on hyphal formation of DPP3 knockout mutant of C. albicans
In order to confirm whether the increase of Dpp3 is the only mechanism of MPD inhibiting hypha, the inhibitory effect of MPD on the hyphal formation of DPP3 knockout mutant was tested. As shown in , SN152 and KWN2 strains were able to form normal hyphae in the absence of MPD, and formed complex hyphal networks after 12 h. The addition of MPD significantly inhibited the hyphal formation of SN152 strain. KWN2 strain remained in the yeast state within 6 h under the treatment of MPD, but gradually formed hyphae after 12 h. Notably, MPD still inhibited the hyphae of KWN2 strain to a certain extent compared with the control group. This result indicated that increased DPP3 is not the only mechanism of MPD inhibiting hyphae, but its main contribution cannot be ignored.
MPD shows low cytotoxicity and hemolytic activity
Two normal cell lines were used to analyze the cytotoxicity of MPD. These results indicated that the IC50 values of MPD against HT22 and HEK293T were 12.53 and >100 μg/mL, respectively, well above the MIC of MPD against C. albicans (). The hemolytic assay was performed on human erythrocytes. MPD had negligible hemolytic effects on human erythrocytes even at high concentrations (4 and 8 μg/mL) (). What is more, MPD and FLC exhibited similar hemolytic activity, which was much lower than that of clinical antifungal drug AMB. These results implied that MPD has low cytotoxicity and hemolytic activity in vivo.
Table 3. The IC50 values of MPD against different cell lines.
Table 4. Hemolytic activity of MPD against human erythrocytes.
MPD exhibits antifungal therapeutic efficacy in vivo
To evaluate the antifungal activity of MPD in vivo, we utilized the G. mellonella infection model. First, the potential toxicity of MPD to G. mellonella was assessed. Compared with the control group, the treatment with 1, 2, 4, and 8 μg/mL MPD for 5 days had no significant effect on the survival of healthy larvae (). The survival time of infected larvae in the MPD-treated group was greater than in the control group according to the survival curve. The same dose of MPD and FLC (2 μg/larva) had similar therapeutic effect on the infected larvae, and the survival rates were 80% and 73%, respectively (). In addition, MPD and FLC greatly reduced the fungal burden compared with the control group (). These results indicated the potential use of MPD in overcoming C. albicans infection in vivo.
Figure 8. The effect of MPD on the G. mellonella-C. albicans infection model. (a) Healthy larvae were incubated with different doses of MPD for 5 days. (b) Larvae were infected with YEM30 strain and treated with PBS (control), FLC (2 μg/larva), or MPD (2 μg/larva) after 2 h of infection. Monitor larvae each day and calculate the survival rate. (c) The fungal burden of G. mellonella infected with C. albicans under different drug treatments was monitored every day after infection.

Discussion
C. albicans, as an opportunistic pathogen, mainly causes skin, mucosa, and even systemic infection of immunocompromised individuals [Citation31]. Invasive infections caused by Candida are a great threat to human health. In addition, most fungal infections require long-term treatment due to recurrence and persistence. However, drug resistance and side effects generally hinder the application of antifungal drugs [Citation32]. Therefore, novel antifungal therapeutic strategies and drugs are needed.
The maleimide compound contains a complete imide ring, a structure that exhibits hydrophobicity, allowing it to easily cross cell membranes. And this structure gives the maleimide compound biological activity and medicinal use [Citation33]. Therefore, we investigated whether maleimide compounds could be developed as novel antifungal agents.
Uncontrolled proliferation caused by an impaired host immune function is the primary pathogenic cause of C. albicans in vivo. In this study, MPD, a maleimide compound synthesized by our lab, exhibited effective antifungal activities against different C. albicans strains, including wild-type strains, a multi-drug strain, and efflux pump deletion mutants. Importantly, the MIC values of MPD were comparable to FLC, a clinically used antifungal drug. Interestingly, MPD exerted the same activity against the wild-type and efflux pump deletion mutant strains. This suggests that the mode of action of MPD is independent of the efflux pump. Hence, MPD has a low probability to develop resistance since efflux pumps are vital for the development of drug resistance. Additionally, the induced drug resistance testing confirmed that MPD did not lead to drug resistance even when exposed for a long time, while fluconazole was prone to develop resistance. In addition, MPD showed low cytotoxicity in normal cell lines and human erythrocytes. These results encouraged us to carry out further research on the antifungal activities of MPD.
Conventional drugs mainly kill fungal cells or indirectly inhibit fungal growth by targeting cell wall synthesis (echinocandins) and cell membrane (azoles and AMB). C. albicans possesses various virulence factors that promote its colonization, invasion, and spread in tissues [Citation34]. Hence, a promising therapeutic strategy would be to target the virulence factors that play an anti-C. albicans role. Fortunately, the present study found that MPD inhibited the virulence factors in vitro and had a curative effect on the infected larvae in vivo.C. albicans firmly adheres to human epithelial cells, endothelial cells, and even non-biological medical devices, which are essential for colonization of host. Adherence is regulated by various fungal cell wall adhesins, such as agglutinin-like sequence (ALS), eIF4E-associated protein 1 (Eap1), and hyphal wall protein 1 (Hwp1) [Citation35]. C. albicans mutant als1Δ/als1Δ displayed a lower pathogenesis and virulence compared with wild-type strain in the infected mice (in vivo). Contrastingly, an ALS1 overexpressing strain exhibited an increased adherence to the endothelial cells [Citation36]. The reconstituted human epithelium (RHE) model studies showed no RHE damage in als3Δ/als3Δ strain of C. albicans with a complete defect in adhesion [Citation37]. Hwpl is a mannoprotein located on the cell membrane surface of C. albicans, which holds a key role in biofilm formation and cell adhesion [Citation38]. This study found that even 0.25 μg/mL of MPD (1/4 MIC) effectively inhibited the adhesion of C. albicans to abiotic polystyrene surfaces by reducing the transcription of adhesin-related genes, including ALS3 and HWP1.C. albicans exists in two cell forms, yeast, and hypha. Yeast cells colonize the body and spread via blood, causing bloodstream infection. Hyphal cells have strong tissue invasion and infiltration abilities, hence regarded as a key virulence factor for the pathogenicity of C. albicans [Citation39]. When conditions are suitable, the colonized yeast cells are transformed into the hyphal state [Citation40]. Moreover, the tip of hypha secretes hemolysins, phospholipases, and secreted aspartyl proteinases (SAPs), which damage the cell membrane of host epithelial cells, increase the cell membrane permeability, and weaken the defence ability of host cells [Citation41]. C. albicans mutants with defective hyphal formation exhibited attenuated pathogenicity in mice [Citation42]. Therefore, inhibiting the morphological transformation of C. albicans to the hyphal form reduces its pathogenicity [Citation43]. Strikingly, we found that the hyphal development was repressed by 0.5 μg/mL of MPD (1/2 MIC).
Hyphae are crucial for biofilm formation, as previously reported that cells with defective hyphae formation develop impaired biofilms [Citation44]. Biofilm is another important pathogenic trait of C. albicans. Biofilm formation is a continuous process. First, yeast cells adhere and proliferate on the substrate, and then yeast cells transform into hyphal cells, then extracellular substances secrete and accumulate, and finally biofilm is formed [Citation45]. Biofilms are more resistant to host immune factors and antimicrobial drugs. Since MPD inhibits the adhesion and filamentation of C. albicans, it is expected to have an anti-biofilm activity. Further, we explored the molecular mechanisms by which MPD inhibits adhesion, yeast-to-hyphal transformation, and biofilm formation.
Hydrophobicity of C. albicans promotes its adherence to material surfaces and thus increases biofilm formation [Citation46]. In this study, we observed that MPD effectively reduced the cell surface hydrophobicity (CSH) in C. albicans, which is one of the reasons for the attenuated virulence of C. albicans.
Farnesol is secreted by C. albicans into the supernatant that decreases hyphae formation by directly inhibiting the activity of Cdc35. The cAMP-dependent protein kinase A (PKA) pathway is an important signalling pathway that regulates the filamentization of C. albicans [Citation47]. cAMP, synthesized by Cdc35, is a potent regulator of hyphal formation. Inhibition of cAMP synthesis blocks the yeast-to-hyphae transition of C. albicans under most hyphal-inducing conditions [Citation48]. In this study, we found that MPD could enhance the activity of Dpp3, a key protein involved in farnesol synthesis. We tested the response of the dpp3-deficient mutant of C. albicans to treatment with MPD and found that hyphal inhibition of MPD was reduced. This confirmed that the increased DPP3 promoted the secretion of farnesol, which led to the inhibition of mycelium growth. The increased farnesol could inhibit Cdc35 activity to reduce the intracellular cAMP concentrations. These results were confirmed by an exogenous cAMP rescue assay. Moreover, the qRT-PCR results verified that MPD retarded the virulence factors of C. albicans by modulating the Ras1-cAMP-Efg1 pathway ().
Figure 9. The underlying mechanism of MPD inhibiting virulence factors of C. albicans. MPD treatment increased the activity of Dpp3 in YEM30 strain to promote the secretion of farnesol. Farnesol directly inhibits the activity of Cdc35. Cdc35 converts ATP into cAMP, which combines with Bcy1, promoting transcription of downstream genes. These genes regulate a variety of virulence characteristics, such as the regulation of the hyphal morphology induced by EFG1 and TEC1. Meanwhile, EFG1 also regulates the adhesion induced by HWP1 and ALS3. Overall, MPD inhibited virulence factors through the Ras1-Camp-Efg1 pathway.

However, increased DPP3 is unlikely to be the only mechanism of MPD inhibiting hyphae. Farnesol is secreted by C. albicans during the sterol synthetic pathway via dephosphorylation of FPP. FPP, as a forerunner for dolichol and ubiquinone, plays an important role in the ergosterol biosynthesis pathway. Inhibition of the sterol synthetic pathway downstream of farnesol, for example, subinhibitory concentrations of zaragozic acid B, could promote accumulation of FPP, resulting in 8–10 folds higher farnesol levels compared to untreated cells. Accumulation of farnesol triggered by the drug may contribute to their antifungal action [Citation49]. In another study of ours, MPD also exhibited inhibitory effects on the synthesis pathway of ergosterol in C. albicans (data not published). The specific mechanism is similar to FLC targeting membrane synthesis, but MPD has better inhibitory effects on the hyphae of C. albicans.
Although the inhibitory effect of farnesol on mycelium in vitro shows its regulatory function during C. albicans infection, the actual effect of farnesol in vivo is still controversial. One view is that farnesol can inhibit the transformation of yeast to hyphae and has a therapeutic effect in infection models. The other view is that farnesol is a virulence factor that can increase the pathogenicity of C. albicans. Navarathna et al. showed that farnesol (20 mM/mouse) acts as a virulence factor in the mouse tail vein injection assay [Citation50]. However, Hisajima et al. published the finding that farnesol (9 µM/mouse) has a protective role in an oral model of murine infection [Citation51]. These differences in the effects of farnesol, which appear contradictory, can be explained by the different conditions of the administered dose or route of farnesol, immunosuppressed conditions of mice, and in its local and systemic impacts. This raises the question of exactly how much farnesol is produced by C. albicans and remains at the infected site. Finding a definitive answer to this question will be difficult. One thing is certain, however, that excessive exogenous concentrations of farnesol used can cause tissue concentrations to exceed those achieved in normally progressive infections. It is difficult to conclude whether similar effects would be seen with more physiologically relevant farnesol levels.
Regarding the concentration of farnesol needed to block filamentation of C. albicans, the effective concentration is reported from 4 μM to 250 μM [Citation52,Citation53]. In one study, exposure to a high concentration of farnesol (75 μM) led to a significant increase in phospholipase activity of C. albicans, which may be one of the reasons why farnesol was considered as a virulence factor [Citation54]. Another study showed that high concentrations of farnesol (>56 mM) inhibited the anti-Candida activity of macrophages by inducing oxidative stress [Citation55]. Our results showed that the farnesol produced when MPD inhibited mycelium was only 15 μM or even lower. This concentration is lower than the concentration at which farnesol acts as a virulence factor. To confirm that the farnesol induced by MPD does not enhance the virulence of C. albicans in vivo, we utilized the G. mellonella infection model. The model is widely used to assess fungal virulence and antifungal drug efficiency [Citation56]. The results showed that MPD prolonged the survival time of larvae infected with C. albicans and reduced the fungal burden in vivo. Therefore, we speculate that farnesol accumulates in vivo under the action of MPD. When it exceeds the threshold level, yeast will be prevented from transforming into mycelium. But the concentration is not enough to damage the host.
Conclusion
In summary, our findings reveal that MPD effectively inhibits the virulence of C. albicans in vitro by stimulating farnesol secretion and reducing intracellular cAMP content. In addition, MPD exerts curative effects on infected G. mellonella in vivo. Most importantly, MPD does not display cytotoxicity in vitro and in vivo. These findings indicate that MPD plays an antifungal role by targeting virulence factors.
Author contributions
Conceptualization, YL, ZZ, and ZX; Methodology, YL and CC; Investigation, ZZ; Resources, ZX, YL, and ZZ; Data curation, MS, ZH, and SM; Writing – original draft preparation, CC; Software, CC and ZH; Validation, CC, LC, and MS; Formal analysis, LC and SM; Writing – review and editing, CC, YL, ZZ, JM, and SM; Supervision, YL and ZZ; Project administration, ZX and YL.; Funding acquisition, YL, ZZ, and ZX.
Acknowledgements
Thanks to the teachers and students of Public Experimental Research Center and Medical Technology College of Xuzhou Medical University for their help and support.
Disclosure statement
It is stated by the author that the research was conducted without any commercial or financial relationship that may be regarded as a potential conflict of interest.
Data availability statement
The data that support the findings of this study are available at https://www.jianguoyun.com/p/DfLClH8Qn66aCxiR2ugEIAA.
Additional information
Funding
References
- McCarty TP, White CM, Pappas PG. Candidemia and invasive candidiasis. Infect Dis Clin North Am. 2021;35(2):389–17. doi: 10.1016/j.idc.2021.03.007
- Jeffery-Smith A, Taori SK, Schelenz S, et al. Candida auris: a review of the literature. Clin Microbiol Rev. 2018;31(1). doi: 10.1128/CMR.00029-17
- Tong Y, Tang J. Candida albicans infection and intestinal immunity. Microbiol Res. 2017;198:27–35. doi: 10.1016/j.micres.2017.02.002
- An Y, Dong Y, Liu M, et al. Novel naphthylamide derivatives as dual-target antifungal inhibitors: design, synthesis and biological evaluation. Eur J Med Chem. 2021;210:112991. doi: 10.1016/j.ejmech.2020.112991
- Pristov KE, Ghannoum MA. Resistance of Candida to azoles and echinocandins worldwide. Clin Microbiol Infect. 2019;25(7):792–798. doi: 10.1016/j.cmi.2019.03.028
- Dahiya S, Sharma N, Punia A, et al. Antimycotic drugs and their mechanisms of resistance to Candida species. Curr Drug Targets. 2022;23(2):116–125. doi: 10.2174/1389450122666210719124143
- Gioia F, Gomez-Lopez A, Alvarez ME, et al. Pharmacokinetics of echinocandins in suspected Candida peritonitis: a potential risk for resistance. Int J Infect Dis. 2020;101:24–28. doi: 10.1016/j.ijid.2020.09.019
- Sortino M, Cechinel Filho V, Corrêa R, et al. N-Phenyl and N-phenylalkyl-maleimides acting against Candida spp.: time-to-kill, stability, interaction with maleamic acids. Bioorgan Med Chem. 2008;16(1):560–568. doi: 10.1016/j.bmc.2007.08.030
- Bhagare AM, Aher JS, Gaware MR, et al. Novel Schiff bases derived from N-aryl maleimide derivatives as an effective antimicrobial agent: the oretical and experimental approach. Bioorg Chem. 2020;103:104129. doi: 10.1016/j.bioorg.2020.104129
- CLSI (2020). Clinical and Laboratory Standards Institute Method for Antifungal Disk Diffusion Susceptibility Testing of Yeasts CLSI Document M44. 3rd ed (Wayne, PA: Clinical and Laboratory Standards Institute;). Available at: https://clsi.org/standards/products/microbiology/documents/m44/
- Song M, Zhang M, Lu J, et al. Palmarumycin P3 reverses mrr1-mediated azole resistance by blocking the efflux pump Mdr1. Antimicrob Agents Chemother. 2022;66(3):e0212621. doi: 10.1128/aac.02126-21
- Chang W, Li Y, Zhang L, et al. Retigeric acid B enhances the efficacy of azoles combating the virulence and biofilm formation of Candida albicans. Biol Pharm Bull. 2012;35(10):1794–1801. doi: 10.1248/bpb.b12-00511
- Wang M, Gu K, Ding W, et al. Antifungal effect of a new photosensitizer derived from BODIPY on Candida albicans biofilms. Photodiagn Photodyn. 2022;39:102946. doi: 10.1016/j.pdpdt.2022.102946
- Biniarz P, Baranowska G, Feder-Kubis J, et al. The lipopeptides pseudofactin II and surfactin effectively decrease Candida albicans adhesion and hydrophobicity. Anton leeuw int j g. 2015;108(2):343–353. doi: 10.1007/s10482-015-0486-3
- Li Y, Shan M, Yan M, et al. Anticandidal activity of kalopanaxsaponin a: effect on proliferation, cell morphology, and key virulence attributes of Candida albicans. Front Microbiol. 2019;10:2844. doi: 10.3389/fmicb.2019.02844
- Li Y, Chang W, Zhang M, et al. Natural product solasodine-3-O-β-D-glucopyranoside inhibits the virulence factors of Candida albicans. FEMS Yeast Res. 2015;15(6):fov060. doi: 10.1093/femsyr/fov060
- Zhang L, Chang W, Sun B, et al. Bisbibenzyls, a new type of antifungal agent, inhibit morphogenesis switch and biofilm formation through upregulation of DPP3 in Candida albicans. PLoS One. 2011;6(12):e28953. doi: 10.1371/journal.pone.0028953
- Wu XZ, Cheng AX, Sun LM, et al. Effect of plagiochin E, an antifungal macrocyclic bis(bibenzyl), on cell wall chitin synthesis in Candida albicans. Acta Pharmacol Sin. 2008;29(12):1478–1485. doi: 10.1111/j.1745-7254.2008.00900.x
- Li Y, Chang W, Zhang M, et al. Diorcinol D exerts fungicidal action against Candida albicans through cytoplasm membrane destruction and ROS accumulation. PLoS One. 2015;10(6):e0128693. doi: 10.1371/journal.pone.0128693
- Duan X, Xie Z, Ma L, et al. Selective metal chelation by a thiosemicarbazone derivative interferes with mitochondrial respiration and ribosome biogenesis in Candida albicans. Microbiol Spectr. 2022;10(3):e0195121. doi: 10.1128/spectrum.01951-21
- Girardot M, Millot M, Hamion G, et al. Lichen polyphenolic compounds for the eradication of Candida albicans biofilms. Front Cell Infect Microbiol. 2021;11(null):698883. doi: 10.3389/fcimb.2021.698883
- Sanglard D, Odds FC. Resistance of Candida species to antifungal agents: molecular mechanisms and clinical consequences. Lancet Infect Dis. 2002;2(2):73–85. doi: 10.1016/s1473-3099(02)00181-0
- Ardizzoni A, Boaretto G, Pericolini E, et al. Effects of benzydamine and mouthwashes containing benzydamine on Candida albicans adhesion, biofilm formation, regrowth, and persistence. Clin Oral Invest. 2022;26(4):3613–3625. doi: 10.1007/s00784-021-04330-8
- Qiu L, Zhang TS, Song JZ, et al. BbWor1, a regulator of morphological transition, is involved in conidium-hypha switching, blastospore propagation, and virulence in Beauveria bassiana. Microbiol Spectr. 2021;9(1):e0020321. doi: 10.1128/Spectrum.00203-21
- Kanchanapiboon J, Kongsa U, Pattamadilok D, et al. Boesenbergia rotunda extract inhibits Candida albicans biofilm formation by pinostrobin and pinocembrin. J Ethnopharmacol. 2020;261:113193. doi: 10.1016/j.jep.2020.113193
- Honorato L, de Araujo JFD, Ellis CC, et al. Extracellular vesicles regulate biofilm formation and yeast-to-hypha differentiation in Candida albicans. MBio. 2022;13(3):e0030122. doi: 10.1128/mbio.00301-22
- Chen M, Cheng T, Xu C, et al. Sodium houttuyfonate enhances the mono-therapy of fluconazole on oropharyngeal candidiasis (OPC) through HIF-1α/IL-17 axis by inhibiting cAMP mediated filamentation in Candida albicans-Candida glabrata dual biofilms. Virulence. 2022;13(1):428–443. doi: 10.1080/21505594.2022.2035066
- Lee JH, Kim YG, Khadke SK, et al. Antibiofilm and antifungal activities of medium-chain fatty acids against Candida albicans via mimicking of the quorum-sensing molecule farnesol. Microbiol Biotechnol. 2021;14(4):1353–1366. doi: 10.1111/1751-7915.13710
- Liu RH, Shang ZC, Li TX, et al. In Vitro Antibiofilm Activity of Eucarobustol E against Candida albicans. Antimicrob Agents Ch. 2017;61(8). doi: 10.1128/AAC.02707-16
- Pukkila-Worley R, Ausubel FM, Mylonakis E, et al. Candida albicans infection of Caenorhabditis elegans induces antifungal immune defenses. PLOS Pathog. 2011;7(6):e1002074. doi: 10.1371/journal.ppat.1002074
- Wang B, Wang Y, Zhang L, et al. Surveillance study of epidemiology, antifungal susceptibility and risk factors of invasive candidiasis in critically ill Patients. Clin Lab. 2022;68(6/2022). doi: 10.7754/Clin.Lab.2021.211001
- Hoenigl M, Sprute R, Arastehfar A, et al. Invasive candidiasis: investigational drugs in the clinical development pipeline and mechanisms of action. Expert Opin Inv Drug. 2022;31(8):1–18. doi: 10.1080/13543784.2022.2086120
- Fan Y, Lu Y, Chen X, et al. Anti-leishmanial and cytotoxic activities of a series of maleimides: synthesis, biological evaluation and structure-activity relationship. Molecules. 2018;23(11):2878. doi: 10.3390/molecules23112878
- Mayer FL, Wilson D, Hube B. Candida albicans pathogenicity mechanisms. Virulence. 2013;4(2):119–128. doi: 10.4161/viru.22913
- McCall AD, Pathirana RU, Prabhakar A, et al. Candida albicans biofilm development is governed by cooperative attachment and adhesion maintenance proteins. NPJ Biofilms Microbiomes. 2019;5(1):21. doi: 10.1038/s41522-021-00264-x
- Fu Y, Ibrahim AS, Sheppard DC, et al. Candida albicans Als1p: an adhesin that is a downstream effector of the EFG1 filamentation pathway. Mol Microbiol. 2002;44(1):61–72. doi: 10.1046/j.1365-2958.2002.02873.x
- Zhao X, Oh SH, Cheng G, et al. ALS3 and ALS8 represent a single locus that encodes a Candida albicans adhesin; functional comparisons between Als3p and Als1p. Microbiol-sgm. 2004;150(7):2415–2428. doi: 10.1099/mic.0.26943-0
- Ene IV, Bennett RJ. Hwp1 and related adhesins contribute to both mating and biofilm formation in Candida albicans. Eukaryot Cell. 2009;8(12):1909–1913. doi: 10.1128/EC.00245-09
- Kumar A. The complex genetic basis and multilayered regulatory control of yeast pseudohyphal growth. Ann Rev Genet. 2021;55(1):1–21. doi: 10.1146/annurev-genet-071719-020249
- Naglik JR, Moyes DL, Wächtler B, et al. Candida albicans interactions with epithelial cells and mucosal immunity. Microbes Infect. 2011;13(12–13):963–976. doi: 10.1016/j.micinf.2011.06.009
- Mitchell AP. Microbiology: fungus produces a toxic surprise. Nature. 2016;532(7597):41–42. doi: 10.1038/nature17319
- Antinori S, Milazzo L, Sollima S, et al. Candidemia and invasive candidiasis in adults: a narrative review. Eur J Intern Med. 2016;34:21–28. doi: 10.1016/j.ejim.2016.06.029
- Chen H, Zhou X, Ren B, et al. The regulation of hyphae growth in Candida albicans. Virulence. 2020;11(1):337–348. doi: 10.1080/21505594.2020.1748930
- Nobile CJ, Mitchell AP. Genetics and genomics of Candida albicans biofilm formation. Cell Microbiol. 2006;8(9):1382–1391. doi: 10.1111/j.1462-5822.2006.00761.x
- Zarnowski R, Noll A, Chevrette MG, et al. Coordination of fungal biofilm development by extracellular vesicle cargo. Nat Commun. 2021;12(1):6235. doi: 10.1038/s41467-021-26525-z
- Monniot C, Boisramé A, Da Costa G, et al. Rbt1 protein domains analysis in Candida albicans brings insights into hyphal surface modifications and Rbt1 potential role during adhesion and biofilm formation. PLoS One. 2013;8(12):e82395. doi: 10.1371/journal.pone.0082395
- Song P, Peng G, Yue H, et al. Candidalysin, a virulence factor of candida albicans, stimulates mast cells by mediating cross-talk between signaling pathways activated by the dectin-1 receptor and MAPKs. J Clin Immunol. 2022;42(5):1009–1025. doi: 10.1007/s10875-022-01267-9
- Huang G, Huang Q, Wei Y, et al. Multiple roles and diverse regulation of the Ras/cAMP/protein kinase a pathway in Candida albicans. Mol Microbiol. 2019;111(1):6–16. doi: 10.1111/mmi.14148
- Hornby JM, Kebaara BW, Nickerson KW. Farnesol biosynthesis in Candida albicans: cellular response to sterol inhibition by zaragozic acid B. Antimicrob Agents Ch. 2003;47(7):2366–2369. doi: 10.1128/AAC.47.7.2366-2369.2003
- Navarathna DHMLP, Hornby JM, Krishnan N, et al. Effect of farnesol on a mouse model of systemic candidiasis, determined by use of a DPP3 knockout mutant of Candida albicans. Infect Immun. 2007;75(4):1609–1618. doi: 10.1128/IAI.01182-06
- Hisajima T, Maruyama N, Tanabe Y, et al. Protective effects of farnesol against oral candidiasis in mice. Microbiol Immunol. 2008;52(7):327–333. doi: 10.1111/j.1348-0421.2008.00044.x
- Kruppa M, Krom BP, Chauhan N, et al. The two-component signal transduction protein Chk1p regulates quorum sensing in Candida albicans. Eukaryot Cell. 2004;3(4):1062–1065. doi: 10.1128/EC.3.4.1062-1065.2004
- Nagy F, Vitális E, Jakab Á, et al. In vitro and in vivo effect of exogenous farnesol exposure against Candida auris. Front Microbiol. 2020;11(null):957. doi: 10.3389/fmicb.2020.00957
- Mosel DD, Dumitru R, Hornby JM, et al. Farnesol concentrations required to block germ tube formation in Candida albicans in the presence and absence of serum. Appl Environ Microb. 2005;71(8):4938–4940. doi: 10.1128/AEM.71.8.4938-4940.2005
- Abe S, Tsunashima R, Iijima R, et al. Suppression of anti-Candida activity of macrophages by a quorum-sensing molecule, farnesol, through induction of oxidative stress. Microbiol Immunol. 2009;53(6):323–330. doi: 10.1111/j.1348-0421.2009.00128.x
- Jemel S, Guillot J, Kallel K, et al. Galleria mellonella for the evaluation of antifungal efficacy against medically important fungi, a narrative review. Microorganisms. 2020;8(3):390. null. doi: 10.3390/microorganisms8030390