ABSTRACT
Streptococcus suis is a bacterium that can cause infections in pigs and humans. Although oxidative stress is common occurrence during bacterial growth and infection, the regulation networks of S. suis under oxidative stress remain poorly understood. To address this, we utilized RNA-Seq to reveal the transcriptional landscape of S. suis in response to H2O2 stress. We identified novel genes responsible for S. suis resistance to oxidative stress, including those involved in DNA repair or protection, and essential for the biosynthesis of amino acids and nucleic acids. In addition, we found that a novel aquaporin, Aagp, belonging to atypical aquaglyceroporins and widely distributed in diverse S. suis serotypes, plays a crucial role during H2O2 stress. By performing oxidative stress assays and measuring the intracellular H2O2 concentrations of the wild-type strain and Aagp mutants during H2O2 stress, we found that Aagp facilitated H2O2 efflux. Additionally, we found that Aagp might be involved in glycerol transport, as shown by the growth inhibition and H2O2 production in the presence of glycerol. Mice infection experiments indicated that Aagp contributed to S. suis virulence. This study contributes to understanding the mechanism of S. suis oxidative stress response, S. suis pathogenesis, and the function of aquaporins in prokaryotes.
Introduction
Streptococcus suis can cause systemic diseases such as septicaemia and meningitis in pigs. It is also considered as a zoonotic pathogen for humans in close contact with infected pigs or contaminated by-products [Citation1–3]. During the growth and infection process, S. suis may encounter oxidative stress. Reactive oxygen species (ROS) like hydroxyl radicals (HO•), hydrogen peroxide (H2O2), and superoxide anion (O2−) are generated during many cellular activities [Citation4]. O2− and H2O2 result from one-electron and two-electron reduction of O2, respectively [Citation5,Citation6]. H2O2 can oxidize Fe2+ in ferritin, leading to inactivation of the enzymes and releasing Fe3+, and then Fe3+ is reduced to Fe2+ in the intracellular environment [Citation5]. When Fe2+ reacts with H2O2, Fenton chemistry is occurred and generates highly-reactive HO• [Citation7]. O2−, H2O2, and HO• can cause cell damage by oxidizing amino acids, DNA, and lipids [Citation5,Citation8]. When bacteria invade the host, innate immune cells, including neutrophils, monocytes, and macrophages, are responsible for ROS production, which is essential to eliminate bacteria [Citation9]. In addition, ROS participates as a signal molecular in activating various immune mechanisms [Citation10]. In order to defend against oxidative stress, S. suis has developed various mechanisms that are regulated by transcriptional regulators, including PerR, Rex, SpxA1, FlpS, and SrtR, which target genes encoding superoxide dismutase, catalase, thioredoxin, glutathione reductase, iron uptake (Fur), and ferritin [Citation11–15]. However, since antioxidant defence systems involve comprehensive and complex processes, studies focusing solely on individual regulators cannot fully reveal regulation networks under oxidative stress.
In this study, we performed RNA-sequencing (RNA-Seq) analysis to explore the global oxidative stress response of S. suis. Our findings revealed a comprehensive defence network in response to H2O2 stress, and we identified a novel aquaporin, Aagp, that plays a crucial role in facilitating S. suis H2O2 efflux and contributes to virulence.
Materials and methods
Bacterial strains and culture conditions
Supplementary Table S1 lists S. suis strains and plasmids. S. suis serotype 9 virulent strain GZ0565 was isolated from a meningitis pig [Citation16]. S. suis strains were cultured in Todd-Hewitt broth (THB, Hopebio, Qingdao, China) and plated on an agar (THA) medium containing 6% (vol/vol) sheep blood at 37 °C with 5% CO2. Escherichia coli strains were grown in Luria-Bertani (LB, Becton Dickinson, USA) broth at 37 °C. Antibiotics were added when in need as follows: spectinomycin (Spc, Macklin, China), 50 μg/mL for E. coli, and 100 μg/mL for S. suis, 10% (w/v) sucrose (sucrose, Macklin, China) for S. suis.
For studying glycerol utilization, S. suis strains were grown in THB to mid-exponential phase (OD600 = 0.6), washed twice in PBS, and then transferred (with a dilution of 1:100) to a 50 mL flask with 10 mL of 1/3 diluted THB or 1/3 diluted THB containing glycerol with a final concentration of 10 mM or 100 mM. The growth rates were measured at OD600 at 37 °C in a shaking incubator.
RNA-Seq analysis
To serve as a control group, S. suis strain GZ0565 was grown in THB to reach the mid-exponential phase. For H2O2 treatment group, when the bacteria had reached the mid-exponential phase, a final concentration of 25 mM H2O2 was added to THB medium and incubated for 25 min. After incubation, the cultures were centrifuged at 5000×g for 10 min at 4 °C, and the bacterial pellets were collected. The total RNA was extracted using the FastRNA Pro Blue Kit (MP Biomedicals) according to our previous report [Citation17]. The extracted RNA samples were submitted to Realbio Technology (Shanghai, China) for RNA-seq analysis. Each experimental group was performed in duplicate. The library construction for RNA-seq was described in our previous study [Citation18]. Sequencing was carried out using Illumina HiSeq 2500 (Illumina, USA) following the manufacturer’s protocol. The sequencing reads were aligned by Bowtie2 [Citation19]. The gene expression was calculated by FPKM (Fragments Per Kilobase of transcript per Million mapped reads), and differential expression analysis was performed using edgeR [Citation20]. The threshold of p < 0.01 and the absolute value of Fold change ≥ 2 were used to identify the differentially expressed genes.
Construction mutant strains
The detailed protocol for deleting Aagp (BFP66_RS01250) in strain GZ0565 background using S. suis-E. coli shuttle plasmid pSET4s was described in our previous study [Citation21]. In addition, we created a mutation strain of Aagp in strain GZ0565 background (Aagpmut) by inserting single “G” base into its coding sequence (CDS), causing a frameshift that rendered Aagp non-functional. This mutation strain was constructed through a two-step natural transformation [Citation22]. Step I was performed to replace the upstream sequence of Aagp with SacB-Spc cassette and resulted in sucrose sensitive and spectinomycin resistant; step II was the process of cassette replacement for single base insertion via the negative selection on sucrose THA plate (Supplementary Figure S1). We also employed the same methodology to construct deletions of exodeoxyribonuclease III (exo III, BFP66_RS05940), AguB (BFP66_RS06520), metQ (BFP66_RS08225) in strain GZ0565 background, as well as Aagp in serotype 2 strain P1/7 background (AagpP1/7). Supplementary Table S2 contains a list of primers used for constructing all these strains.
Oxidative stress assays
To assess the role of the Aagp in oxidative stress response, S. suis strains were challenged with H2O2. Wild-type strain GZ0565 (WT), ΔAagp, and Aagpmut were cultured to the mid-exponential phase. Then the final concentration of 25 mM H2O2 was added to THB. After incubation at 37 °C for 25 min, the number of bacteria was determined by spreading serial dilutions on THA plates. The survival rate at each time point was calculated as CFU at time point 25 min/CFU at time point 0. The functions of exo III, AguB, metQ and AagpP1/7 in response to oxidative stress were evaluated using the same methodology. Experiments were performed with three biological replicates. The statistical analysis was performed with a two-tailed unpaired t test.
To further assess the susceptibility of WT, ΔAagp, and Aagpmut to H2O2, the Oxford cup supplemented with 10 µL 1 M H2O2 was conducted on the THA plates. After incubation at 37 °C with 5% CO2 for 24 h, a transparent circle formed, indicating an inhibition zone.
Measurement of H2O2 in cells
After treated with 25 mM H2O2 for 25 min, one mL bacterial cultures were centrifuged at 5000 × g at 4 °C for 10 min and subsequently washed once with PBS and resuspended in 1 mL PBS. According to the manufacturer’s protocol, the concentration of H2O2 in cells was measured using the Pierce Quantitative Peroxide Assay Kits (Thermo Fisher Scientific, Shanghai, China). Experiments were performed with two biological replicates and repeated two times. A two-tailed unpaired t test was used for statistical analysis.
Determination of viable bacteria in organs
The virulence of WT and ΔAagp was assessed in mice according to our previous report [Citation23]. Mice infection was carried out in the Laboratory Animal Center of Nanjing Agricultural University with the approval of the institution’s ethics committee (Permit number SYXK (Su) 2021–0086). Bacteria were cultured to the mid-exponential phase and washed twice with PBS. Six-week SPF CD1 female mice (SiPeiFu Biotechnology Co., Ltd, China) were used for infection (five mice per group). The mice were intraperitoneally injected with a dose of 1.5 × 108 CFU of the WT or ΔAagp. All mice were euthanized at 24 h post-infection. Blood samples were collected from the heart, and liver, kidney, and brain samples were taken, weighed, suspended in PBS, and homogenized. Plating serial dilutions on THA determined the number of viable bacteria in organs and blood. Experimental results were shown as mean ± standard error of the mean (SEM). The statistical analysis was performed with a two-tailed unpaired t test.
RNA extraction and quantitative real-time PCR (RT-qPCR)
H2O2 treatment and RNA extraction were performed as described above for RNA-Seq analysis. The detailed protocol for RT-qPCR was described previously [Citation18]. Supplementary Table 2 lists primers for RT-qPCR analysis. The gene BFP66_RS5620 (ParC) was used as the internal control. Three biological replicates were used. The 2−ΔΔCT method was used to calculate the relative fold change. Data were shown as mean ±SEM.
Results
The transcriptional landscape of S. suis oxidative response
The RNA-Seq was used to explore the transcriptional landscape of S. suis in response to H2O2. Compared with the control condition in the THB medium, 371 differently expressed genes (DEGs) were observed in the H2O2 treatment condition, consisting of 112 upregulated and 259 downregulated genes (Supplementary Table 3). The putative functions of these genes were classified into different functional categories according to the gene ontology (GO) (Supplementary Figure S2A). The DEGs are involved in various biological processes, such as biosynthesis of amid acids, carbohydrate transport and metabolism, metal ion transport, and transcriptional regulation.
As shown in , we found that genes involved in DNA repair or protection were upregulated under H2O2 stress, including BFP66_RS05940 encoding an exodeoxyribonuclease III (exo III) and BFP66_RS06520 encoding an N-carbamoylputrescine amidase (AguB). The gene expression of enzymes encoded by BFP66_RS01580, BFP66_RS05715, BFP66_RS06935, BFP66_RS07745, BFP66_RS04160 (LDH) was upregulated under H2O2 condition, and these enzymes contribute to producing oxidized dinucleotide cofactors (NAD+, FAD+, and NADP+) which are essential for the biosynthesis of amino acids and nucleic acids. As shown in and , six genes involved in glycolysis were upregulated under H2O2 condition, contributing to producing more ATP. In contrast, several genes related to energy-consuming pathways were downregulated, including six genes related to sugar ABC transporters, 13 related to the PTS system, and seven related to glycerol metabolism. RT-qPCR was performed to verify the transcriptional data. Eight upregulated and four downregulated genes were selected, and their expressions were consistent with those obtained from the RNA-seq (Supplementary Figure S2B).
Figure 1. The transcriptional landscape of S. suis oxidative response. (a) RNA-Seq analysis was performed to explore the global H2O2 stress response of S. suis. The upregulated genes are shown in green, and the downregulated genes are shown in red. The detailed information and fold change of genes shown in this figure are summarized in table 1. (b) The survival rate of WT, ΔAagp, Δexo III, ΔAguB, and ΔmetQ was determined after 25 min treated with 25 mM H2O2. The statistical analyses were performed with a two-tailed unpaired t test. “****” indicate p < 0.001.
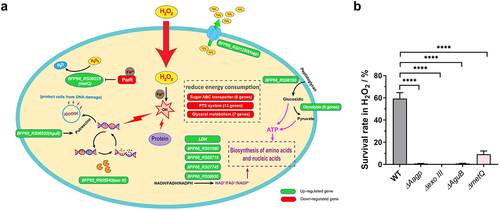
Table 1. The key differentially expressed genes under H2O2 stressa.
To further investigate the response of S. suis to H2O2 at the transcriptional level, we selected four DEGs, namely exo III, AguB, metQ (encoding a methionine transporter) and Aagp (encoding an aquaporin), to examine their function under oxidative stress conditions. After subjecting the deletion mutations to H2O2 treatment, the survival rates of mutants ΔAagp, Δexo III, ΔAguB, and ΔmetQ were 0.7075%, 0.0063%, 0.69%, and 9.1867%, respectively, which were significantly lower than that of wild-type (WT) strain (59.3133%) (). These findings suggest that these genes are involved in oxidative stress responses and support the transcriptional landscape data of S. suis in response to H2O2.
Aagp, a novel aquaporin, is widely distributed in S. suis
Out of the four genes associated with oxidative stress responses, Aagp showed the highest fold change (), making it the chosen candidate for further investigation. Aquaporins contain an aromatic/arginine (ar/R) substrate selectivity motif that comprises one arginine and three other amino acids, and these three amino acids are conserved in every aquaporin subfamily [Citation24]. In S. pneumoniae, a new aquaporin subfamily called atypical aquaglyceroporin has YVPR as the substrate selectivity motif, which is different from glycerol-transporting aquaporins with WG(F/Y)R as the motif and water-transporting aquaporins with F(H/I)XR as the motif (). S. suis aquaporin Aagp shares 68.17% amino acid identity with S. pneumoniae atypical aquaglyceroporin Pn-AqpC [Citation25], belonging to a novel aquaporin subfamily. Aagp was predicted to be a transmembrane protein with five transmembrane loops, and it possesses substrate-selective residues YVPR as Pn-AqpC ().
Figure 2. S. suis Aagp belongs to atypical aquaglyceroporins. The S. suis Aagp transmembrane helices were predicted (top panel). Asterisks specify the ar/R region residues, and black boxes mean the atypical aquaglyceroporins’ substrate-selective residues (YVPR).
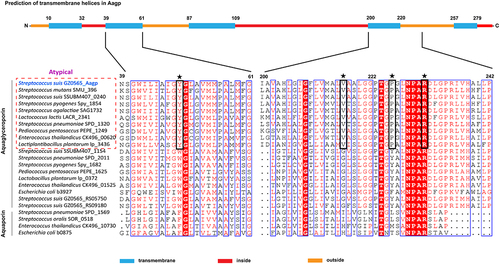
A search for Aagp in the NCBI database revealed that 126 S. suis strains contained Aagp, distributed across numerous serotypes, including serotypes 1–9, 12, 16, 19, 24, 28, and 31 (). To confirm the function of Aagp further, a deletion strain of AagpP1/7 was constructed in S. suis serotype 2 strain P1/7. When challenged with H2O2, the survival rate of ΔAagpP1/7 was reduced to 15.1%, compared to 37.0775% for strain P1/7 (). These findings provide further evidence supporting the role of S. suis Aagp in oxidative stress responses.
Figure 3. Aagp, a novel aquaporin, is widely distributed in S. suis. (a) The distribution of Aagp was assessed in 126 S. suis strains by examining Aagp sequences obtained from the NCBI database. (b) The survival rates of P1/7 and ΔAagpP1/7 were determined after 25 min treated with 25 mM H2O2. The statistical analyses were performed with a two-tailed unpaired t test. “**” indicate p < 0.01.
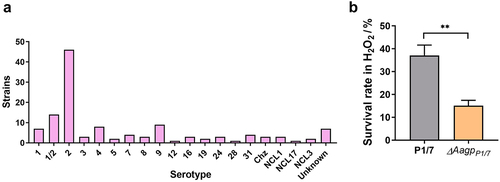
Aagp facilitates H2O2 efflux and alleviates oxidative stress
Tong et al. reported that an aquaporin, So-AqpA, of Streptococcus oligofermentans contributes to the diffusion of H2O2 across the membrane [Citation26]. To investigate the involvement of Aagp in H2O2 diffusion, we measured the concentration of H2O2 in bacterial cells treated with 25 mM H2O2. The concentration of H2O2 treated for 25 min in WT (59.22% survival rate) was 8.4067 pmol per 106 cells, which was dramatically lower than 430.731 pmol per 106 cells in ΔAagp (0.96% survival rate) (). Despite several attempts, we failed to construct an Aagp complementary vector based on plasmids pSET1, pSET2, and pSET3. Therefore, we created a frameshift mutation strain, Aagpmut, by inserting one base into its CDS, as we reported in a previous study [Citation27]. Similar to the results obtained from ΔAagp, we found that the survival rate was lower in Aagpmut compared with WT, while the concentration of H2O2 in Aagpmut was significantly higher than in WT (). Additionally, we compared the response to H2O2 between the WT and Aagp mutations via the Oxford cup test. We found that both ΔAagp and Aagpmut strains exhibited larger inhibition zones than the WT strain (). These results collectively indicate that Aagp facilitates H2O2 efflux, thereby alleviating oxidative stress.
Figure 4. Aagp facilitates H2O2 efflux. (a) The survival rates of WT and ΔAagp were determined after 25 min treated with 25 mM H2O2. (b) The H2O2 concentrations of WT and ΔAagp were measured after 25 min treated with 25 mM H2O2. (c) The survival rates of WT and Aagpmut were determined after 25 min treated with 25 mM H2O2. (d) The H2O2 concentrations of WT and Aagpmut were measured after 25 min treated with 25 mM H2O2. (e) The response to H2O2 between the wild type and Aagp mutations via the Oxford cup test. The statistical analyses were performed with a two-tailed unpaired t test. “*” indicates p < 0.05 and “**” indicates p < 0.01.
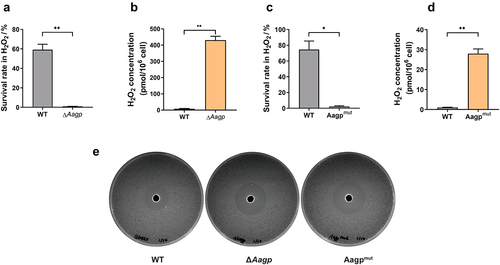
Aagp may be involved in glycerol transport
Previous studies have shown that some aquaporins facilitate glycerol diffusion [Citation28]. To determine the function of Aagp in relation to glycerol, the growth curves of ΔAagp and WT were performed in diluted THB medium (1/3 THB as a poor medium condition) containing 10 mM glycerol or 100 mM glycerol under aerobic condition. As shown in , the growth of WT was inhibited as the concentration of glycerol increased, whereas the growth of ΔAagp was not affected in the absence or presence of glycerol. As shown in , S. suis strain GZ0565 possesses genes involved in the phosphorylation pathway for glycerol metabolism, which is an energy-consuming pathway. Under the aerobic condition, glycerol may be phosphorylated by glycerol kinase (GlpK) and then oxidized by glycerol-3-phosphate oxidase (GlpO), which is accompanied by H2O2 production that can be toxic to bacteria [Citation29]. To test this hypothesis, the concentrations of H2O2 in WT and ΔAagp were measured in 1/3 THB containing 10 mM glycerol or 100 mM glycerol. The concentration of H2O2 in WT cultured in 1/3 THB was 0.0178 pmol per 106 cells, but in the presence of additional glycerol, the concentration of H2O2 increased and reached to 0.1007 pmol per 106 cells when cultured in 1/3 THB containing 100 mM glycerol. Conversely, in ΔAagp, the concentration of H2O2 was similar in the absence or presence of glycerol (). These results suggest that Aagp may be involved in glycerol transport.
Figure 5. Aagp is involved in glycerol transport. The growth curves of WT (a) and ΔAagp (b) cultured in 1/3 THB, 1/3 THB containing 10 mM glycerol or 100 mM glycerol were shown. (c) S. suis strain GZ0565 possesses a phosphorylation pathway for glycerol metabolism. The downregulated genes are shown in red in H2O2 treatment compared with THB condition by RNA-Seq analysis. (d) The H2O2 concentrations of WT and ΔAagp cultured in 1/3 THB, 1/3 THB containing 10 mM glycerol or 100 mM glycerol were determined. The statistical analyses were performed with a two-tailed unpaired t test. “*” indicates p < 0.05.
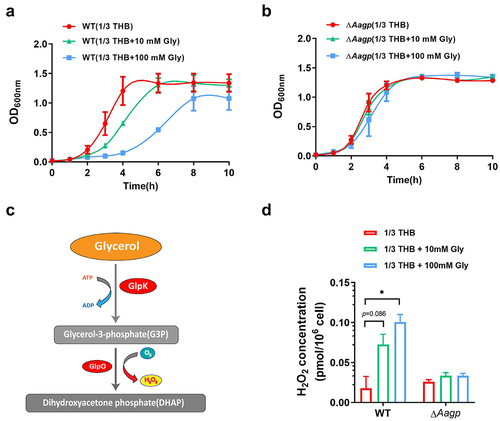
Aagp contributes to S. suis virulence in a mouse infection model
Given that Aagp alleviates S. suis oxidative stress, the contribution of Aagp to S. suis virulence was assessed in mice. Compared with WT infection groups, the number of ΔAagp in blood, liver, spleen, kidney, and brain tissues was significantly reduced, indicating that Aagp contributes to S. suis virulence (). These results indicate that Aagp contributes to S. suis virulence in a mouse infection model.
Figure 6. Aagp contributes to S. suis virulence in a mouse infection model. Five mice per group were injected intraperitoneally with 1.5 × 108 CFU of WT and ΔAagp. All mice were euthanized at 24 h post-infection. Bacteria from blood, liver, spleen, kidney and brains were plated onto THA, and colonies were expressed as Log10 CFU/mg or Log10 CFU/mL. The statistical analyses were performed with a two-tailed unpaired t test. “***” indicate p < 0.001.
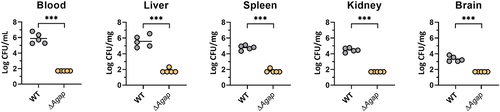
Discussion
Oxidative stress is a common challenge for S. suis to overcome in response to infection or environmental conditions. In this study, we employed RNA-seq to investigate the global transcriptional landscape of S. suis under H2O2 treatment and to reveal the adaptation mechanisms of S. suis response to oxidative stress. Our findings showed that multiple genes involved in oxidative stress were differentially expressed. Some of them have been identified in previous studies. We found that BFP66_RS05715 encoding NADH oxidase, known to contribute to oxidative stress tolerance [Citation30], was upregulated 2.30-fold by H2O2 treatment. We also observed that BFP66_RS01615 encoding trigger factor, which contributes to stress tolerance [Citation31], was upregulated 3.12-fold by H2O2 treatment. Additionally, we noted a downregulation of BFP66_RS01545 encoding Fur-like protein PerR by 3.62-fold in response to H2O2 stress, consistent with previous research showing that the PerR deletion mutant is less sensitive to H2O2 stress [Citation12]. PerR negatively regulates metQ, and its derepression increases methionine utilization, converting H2O2 to H2O [Citation12]. Although RNA-Seq analysis indicated a modest 1.35-fold up-regulation of metQ (BFP66_RS08225) (p < 0.01), RT-qPCR analysis revealed a 10.65-fold upregulation (Supplementary Figure S2B). Moreover, compared to the WT, ΔmetQ was more sensitive to H2O2, confirming the role of metQ under H2O2 conditions (). These findings indicate that the identified genes are crucial in S. suis response to oxidative stress.
Our study has uncovered novel mechanisms that may be responsible for S. suis resistance against oxidative stress caused by ROS. ROS can cause damage to DNA, proteins, and lipids, and repairing these molecules is crucial to combating oxidative stress. In E. coli, exonuclease III is a major apurinic/apyrimidinic endonuclease that recognizes base lesions and cleaves the glycosylase bond to repair DNA [Citation32]. We found that the gene BFP66_RS05940, which codes for exodeoxyribonuclease III was upregulated in response to H2O2 stress, indicating that it may be involved in DNA repair through cleaving lesions. Additionally, BFP66_RS06520 encoding an N-carbamoylputrescine amidase (AguB) was upregulated upon H2O2 stress. The AguAB pathway converts agmatine to putrescine, protecting E. coli from DNA damage via ROS scavenging [Citation33]. Our deletion studies showed that exo III and AguB are crucial for H2O2 resistance in S. suis (). Furthermore, we observed upregulated genes contributing to the biosynthesis of amino acids and nucleic acid or transport, including five genes involved in producing oxidized dinucleotide cofactors (), BFP66_RS01165 encoding a component of an amino acid ABC transporter, and BFP66_RS04785 encoding a glutamate dehydrogenase. These findings indicated that S. suis enhances its biosynthesis and transport of amino acids and nucleic acid to respond to H2O2 stress. Interestingly, under ROS stress caused by 2,4-dichlorophenoxyacetic acid, E. coli reduces energy-consuming pathways to conserve energy for vital metabolism [Citation33]. In our study, several genes related to energy-consuming pathways were downregulated under H2O2 stress (). In contrast, genes involved in glycolysis were upregulated, indicating that S. suis generates more ATP through enhanced glycolysis and reduces energy-consuming pathways for H2O2 detoxification.
The aquaporins are divided into three subfamilies: supergene channel superaquaporins, water-transporting aquaporin, and glycerol-transporting aquaglyceroporins [Citation34–36]. Recent studies have demonstrated that aquaporins can transport H2O2 and regulate its levels in animals and plants, preventing cells from oxidative stress [Citation37–40]. However, little is known about the function of aquaporins in H2O2 regulation in bacteria. S. oligofermentans aquaporin So-AqpA, sharing only 21.90% amino acid identity with S. suis Aagp, has been reported to facilitate the efflux of endogenous H2O2 and protect the bacterium from oxidant damage [Citation26]. Both S. pneumoniae Pn-AqpC and S. suis Aagp are atypical aquaglyceroporins and contribute to bacterial resistance to H2O2 stress, but their mechanisms are different. Pn-AqpC can facilitate oxygen permeation into pneumococcal, which promotes the generation of endogenous H2O2 and helps S. pneumoniae adapt to higher exogenous H2O2 [Citation25]. In contrast, S. suis Aagp contributes to resistance to oxidative stress by facilitating H2O2 efflux, similar to So-AqpA. Previous studies have shown that in S. oligofermentans, the expression of So-aqpA is negatively regulated by MntR and PerR [Citation26,Citation41]. In our RNA-seq data, we observed a significant reduction in the expressions of MntR (encoded by BFP66_RS09925) and PerR (encoded by BFP66_RS01545), accompanied by a significant upregulation of Aagp during H2O2 stress in S. suis. These findings suggest that MntR and PerR in S. suis may play similar roles in regulating Aagp under H2O2 stress conditions. Additionally, we propose that Aagp may possess dual functions of both H2O2 efflux and glycerol transport. In Lactobacillus plantarum, GlpF2, GlpF3, and GlpF4, sharing 30.37%, 36.63%, and 32.73% amino acid identity with Aagp, respectively, have been shown to be permeable to both H2O2 and glycerol [Citation42]. In our study, we have confirmed that Aagp facilitates H2O2 efflux by oxidative stress assays and measurement of intracellular H2O2 level. However, further investigation is required to determine the glycerol transport ability of Aagp.
Through in-depth analysis, we have identified homologs of Aagp that play a role in virulence in other pathogenic bacteria. In Group B Streptococcus, GlpF, sharing 81% amino acid identity with Aagp, enhances the invasion capacity of Group B Streptococcus into eukaryotic cells [Citation43]. In S. pneumococci, Pn-AqpC contributes to pneumococcal pathogenicity by modulating H2O2 production and pneumolysin release [Citation25]. In L. monocytogenes, the expression of GlpF, sharing 37% amino acid identity with Aagp, is upregulated during intracellular growth [Citation44]. When bacteria invade, host innate immune cells generate ROS such as H2O2. Neutrophils, monocytes, and macrophages, abundant in blood and tissues, play crucial roles in producing ROS to eliminate bacteria. The deletion of Aagp would impair the defence capability of S. suis survival in blood and tissues. Finally, we assume that Aagp contributes to S. suis oxidative stress resistance and promotes its survival in the host by facilitating H2O2 efflux.
In summary, we depicted S. suis defence network response to H2O2 stress and identified a novel aquaporin, Aagp, that alleviated S. suis oxidative stress via facilitating H2O2 efflux, which contributes to virulence. In addition, Aagp might be involved in glycerol transport.
Supplemental Material
Download Zip (386.5 KB)Disclosure statement
No potential conflict of interest was reported by the authors.
Data Availability statement
The data supporting this study’s findings are available from the corresponding author upon reasonable request.
Supplementary material
Supplemental data for this article can be accessed online at https://doi.org/10.1080/21505594.2023.2249789
Additional information
Funding
References
- Goyette-Desjardins G, Auger JP, Xu J, et al. Streptococcus suis, an important pig pathogen and emerging zoonotic agent-an update on the worldwide distribution based on serotyping and sequence typing. Emerg Microbes Infect. 2014 Jun;3(6):e45. doi: 10.1038/emi.2014.45
- Ferrando ML, de Greeff A, van Rooijen WJ, et al. Host-pathogen interaction at the intestinal mucosa correlates with zoonotic potential of Streptococcus suis. J Infect Dis. 2015 Jul 1;212(1):95–11.
- Ferrando ML, Schultsz C. A hypothetical model of host-pathogen interaction of Streptococcus suis in the gastro-intestinal tract. Gut Microbes. 2016;7(2):154–162. doi: 10.1080/19490976.2016.1144008
- Fuangthong M, Helmann JD. The OhrR repressor senses organic hydroperoxides by reversible formation of a cysteine-sulfenic acid derivative. Proc Natl Acad Sci U S A. 2002 May 14;99(10):6690–6695.
- Imlay JA. Pathways of oxidative damage. Annu Rev Microbiol. 2003;57(1):395–418. doi: 10.1146/annurev.micro.57.030502.090938
- Sobota JM, Imlay JA. Iron enzyme ribulose-5-phosphate 3-epimerase in Escherichia coli is rapidly damaged by hydrogen peroxide but can be protected by manganese. Proc Natl Acad Sci U S A. 2011 Mar 29;108(13):5402–5407.
- Dubbs JM, Mongkolsuk S. Peroxide-sensing transcriptional regulators in bacteria. J Bacteriol. 2012 Oct;194(20):5495–5503. doi: 10.1128/JB.00304-12
- Imlay JA. The molecular mechanisms and physiological consequences of oxidative stress: lessons from a model bacterium. Nat Rev Microbiol. 2013 Jul;11(7):443–454. doi: 10.1038/nrmicro3032
- Panday A, Sahoo MK, Osorio D, et al. NADPH oxidases: an overview from structure to innate immunity-associated pathologies. Cell Mol Immunol. 2015 Jan;12(1):5–23. doi: 10.1038/cmi.2014.89
- Li H, Zhou X, Huang Y, et al. Reactive oxygen species in pathogen clearance: the killing mechanisms, the adaption response, and the side effects. Front Microbiol. 2020;11:622534. doi: 10.3389/fmicb.2020.622534
- Willenborg J, Koczula A, Fulde M, et al. FlpS, the FNR-Like protein of Streptococcus suis is an essential, oxygen-sensing activator of the arginine deiminase System. Pathogens. 2016 Jul 21;5(3):51.
- Zhang T, Ding Y, Li T, et al. A Fur-like protein PerR regulates two oxidative stress response related operons dpr and metQIN in Streptococcus suis. BMC Microbiol. 2012 May 30;12(1):85.
- Hu Y, Hu Q, Wei R, et al. The XRE family transcriptional regulator SrtR in Streptococcus suis is involved in oxidant tolerance and virulence. Front Cell Infect Microbiol. 2018;8:452. doi: 10.3389/fcimb.2018.00452
- Zheng C, Xu J, Li J, et al. Two spx regulators modulate stress tolerance and virulence in Streptococcus suis serotype 2. PLoS One. 2014;9(9):e108197. doi: 10.1371/journal.pone.0108197
- Zhu H, Wang Y, Ni Y, et al. The redox-sensing regulator rex contributes to the virulence and oxidative stress response of Streptococcus suis serotype 2. Front Cell Infect Microbiol. 2018;8:317. doi: 10.3389/fcimb.2018.00317
- Wu Z, Zhang W, Lu C. Comparative proteome analysis of secreted proteins of Streptococcus suis serotype 9 isolates from diseased and healthy pigs. Microb Pathog. 2008 Sep;45(3):159–166. doi: 10.1016/j.micpath.2008.04.009
- Wang S, Ma M, Liang Z, et al. Pathogenic investigations of Streptococcus pasteurianus, an underreported zoonotic pathogen, isolated from a diseased piglet with meningitis. Transbound Emerg Dis. 2022 Sep;69(5):2609–2620. doi: 10.1111/tbed.14413
- Wu Z, Wu C, Shao J, et al. The Streptococcus suis transcriptional landscape reveals adaptation mechanisms in pig blood and cerebrospinal fluid. RNA. 2014 Jun;20(6):882–898. doi: 10.1261/rna.041822.113
- Langmead B, Salzberg SL. Fast gapped-read alignment with bowtie 2. Nat Methods. 2012 Mar 4;9(4):357–359.
- Robinson MD, McCarthy DJ, Smyth GK. edgeR: a bioconductor package for differential expression analysis of digital gene expression data. Bioinformatics. 2010 Jan 1;26(1):139–140.
- Dai J, Lai L, Tang H, et al. Streptococcus suis synthesizes deoxyadenosine and adenosine by 5’-nucleotidase to dampen host immune responses. Virulence. 2018;9(1):1509–1520. doi: 10.1080/21505594.2018.1520544
- Zhu Y, Dong W, Ma J, et al. Utilization of the ComRS system for the rapid markerless deletion of chromosomal genes in Streptococcus suis. Future Microbiol. 2019 Feb;14(3):207–222. doi: 10.2217/fmb-2018-0279
- Wu Z, Shao J, Ren H, et al. A Streptococcus suis LysM domain surface protein contributes to bacterial virulence. Vet Microbiol. 2016 May 1;187:64–69.
- Savage DF, O’Connell JD 3rd, Miercke LJ, et al. Structural context shapes the aquaporin selectivity filter. Proc Natl Acad Sci U S A. 2010 Oct 5;107(40):17164–17169.
- Hu Q, Tong H, Wang J, et al. A novel aquaporin subfamily imports oxygen and contributes to pneumococcal virulence by controlling the production and release of virulence factors. MBio. 2021 Aug 31;12(4):e0130921.
- Tong H, Wang X, Dong Y, et al. A Streptococcus aquaporin acts as peroxiporin for efflux of cellular hydrogen peroxide and alleviation of oxidative stress. J Biol Chem. 2019 Mar 22;294(12):4583–4595.
- Ma Z, Peng J, Yu D, et al. A streptococcal Fic domain-containing protein disrupts blood-brain barrier integrity by activating moesin in endothelial cells. PLOS Pathog. 2019 May;15(5):e1007737. doi: 10.1371/journal.ppat.1007737
- Borgnia MJ, Agre P. Reconstitution and functional comparison of purified GlpF and AqpZ, the glycerol and water channels from Escherichia coli. Proc Natl Acad Sci U S A. 2001 Feb 27;98(5):2888–2893.
- Doi Y. Glycerol metabolism and its regulation in lactic acid bacteria. Appl Microbiol Biotechnol. 2019 Jul;103(13):5079–5093. doi: 10.1007/s00253-019-09830-y
- Zheng C, Ren S, Xu J, et al. Contribution of NADH oxidase to oxidative stress tolerance and virulence of Streptococcus suis serotype 2. Virulence. 2017 Jan 2;8(1):53–65.
- Wu T, Zhao Z, Zhang L, et al. Trigger factor of Streptococcus suis is involved in stress tolerance and virulence. Microb Pathog. 2011 Jul-Aug;51(1–2):69–76. doi: 10.1016/j.micpath.2010.10.001
- Shokolenko IN, Alexeyev MF, Robertson FM, et al. The expression of Exonuclease III from E. coli in mitochondria of breast cancer cells diminishes mitochondrial DNA repair capacity and cell survival after oxidative stress. DNA Repair (Amst). 2003 May 13;2(5):471–482.
- Bhat SV, Booth SC, Vantomme EA, et al. Oxidative stress and metabolic perturbations in Escherichia coli exposed to sublethal levels of 2,4-dichlorophenoxyacetic acid. Chemosphere. 2015 Sep;135:453–461.
- Tesse A, Grossini E, Tamma G, et al. Aquaporins as targets of dietary bioactive phytocompounds. Front Mol Biosci. 2018;5:30. doi: 10.3389/fmolb.2018.00030
- Mitchell TJ, Dalziel CE. The biology of pneumolysin. Subcell Biochem. 2014;80:145–160.
- Ishibashi K, Morishita Y, Tanaka Y. The evolutionary aspects of aquaporin family. Adv Exp Med Biol. 2017;969:35–50.
- Bienert GP, Chaumont F. Aquaporin-facilitated transmembrane diffusion of hydrogen peroxide. Biochim Biophys Acta. 2014 May;1840(5):1596–1604. doi: 10.1016/j.bbagen.2013.09.017
- Siefritz F, Tyree MT, Lovisolo C, et al. PIP1 plasma membrane aquaporins in tobacco: from cellular effects to function in plants. Plant Cell. 2002 Apr;14(4):869–876. doi: 10.1105/tpc.000901
- Al Ghouleh I, Frazziano G, Rodriguez AI, et al. Aquaporin 1, Nox1, and Ask1 mediate oxidant-induced smooth muscle cell hypertrophy. Cardiovasc Res. 2013 Jan 1;97(1):134–142.
- Medra?O-Fernandez I, Bestetti S, Bertolotti M, et al. Stress regulates aquaporin-8 permeability to impact cell growth and survival. Antioxid Redox Signal. 2016;24(18):1031–1044. doi: 10.1089/ars.2016.6636
- Chen Z, Wang X, Yang F, et al. Molecular insights into hydrogen peroxide-sensing mechanism of the metalloregulator mntr in controlling bacterial resistance to oxidative stresses. J Biol Chem. 2017 Mar 31;292(13):5519–5531.
- Bienert GP, Desguin B, Chaumont F, et al. Channel-mediated lactic acid transport: a novel function for aquaglyceroporins in bacteria. Biochem J. 2013 Sep 15;454(3):559–570.
- Johri AK, Margarit I, Broenstrup M, et al. Transcriptional and proteomic profiles of group B Streptococcus type V reveal potential adherence proteins associated with high-level invasion. Infect Immun. 2007 Mar;75(3):1473–1483. doi: 10.1128/IAI.00638-06
- Monniot C, Zebre AC, Ake FM, et al. Novel listerial glycerol dehydrogenase- and phosphoenolpyruvate-dependent dihydroxyacetone kinase system connected to the pentose phosphate pathway. J Bacteriol. 2012 Sep;194(18):4972–4982. doi: 10.1128/JB.00801-12