ABSTRACT
Streptococcus suis (S. suis), a significant zoonotic bacterial pathogen impacting swine and human, is associated with severe systemic diseases such as streptococcal toxic shock-like syndrome, meningitis, septicaemia, and abrupt fatality. The multifaceted roles of complement components C5a and C3a extend to orchestrating inflammatory cells recruitment, oxidative burst induction, and cytokines release. Despite the pivotal role of subtilisin-like serine proteases in S. suis pathogenicity, their involvement in immune evasion remains underexplored. In the present study, we identify two cell wall-anchored subtilisin-like serine proteases in S. suis, SspA-1 and SspA-2, as binding partners for C3a and C5a. Through Co-Immunoprecipitation, Enzyme-Linked Immunosorbent and Far-Western Blotting Assays, we validate their interactions with the aforementioned components. However, SspA-1 and SspA-2 have no cleavage activity against complement C3a and C5a performed by Cleavage assay. Chemotaxis assays reveal that recombinant SspA-1 and SspA-2 effectively attenuate monocyte chemotaxis towards C3a and C5a. Notably, the ΔsspA-1, ΔsspA-1, and ΔsspA-1/2 mutant strains exhibit compromised survival in blood, and resistance of opsonophagocytosis, alongside impaired survival in blood and in vivo colonization compared to the parental strain SC-19. Critical insights from the murine and Galleria mellonella larva infection models further underscore the significance of sspA-1 in altering mortality rates. Collectively, our findings indicate that SspA-1 and SspA-2 are novel binding proteins for C3a and C5a, thereby shedding light on their pivotal roles in S. suis immune evasion and the pathogenesis.
Introduction
Streptococcus suis (S. suis) is an important zoonotic pathogen in the swine industry that causes high economic losses worldwide [Citation1]. It is also an emerging and re-emerging infectious pathogen to infect humans exposed to pigs or pork and causes septicaemia, meningitis and pneumonia in both pigs and humans [Citation2]. As a basis of capsular polysaccharides (CPS) antigenicity, S. suis is classified in 29 serotypes (1/2, 1–19, 21, 23–25 and 27–31) [Citation3]. The serotype 1, 2, 5, 9, 14, 16, 28, and 31 can infect humans, and serotype 2 (SS2) is the most virulent and prevalent one in swine and humans worldwide [Citation4]. Since the first reported cases of human S. suis infection in Denmark in 1968, more than 1600 cases have been reported worldwide [Citation5]. SS2 causes two large-scale outbreaks occurred in 1998 and 2005 in China resulting in 229 infections and 52 deaths [Citation6], particularly because the cases presented with streptococcal toxic shock like syndrome (STSLS). Bacterial pathogens evade host innate immune defences and maintain a high dose in blood causing bacteraemia and septicaemia. During these processes, the host complement system is an important factor facilitating clearance of bacterial pathogens [Citation7,Citation8].
The complement system consists of more than 50 soluble and cell surface-bound proteins involved in innate and adaptive immune responses responsible for the defence against pathogenic intruders, inflammation and homoeostasis of the host [Citation9]. It might be activated by three independent but interactive activation routes: the classical, the alternative, and the lectin pathways. All three pathways lead to the formation of opsonin C3b to induce phagocytosis, anaphylatoxin C3a and C5a to trigger pro-inflammatory and chemotactic responses, membrane attack complex formation or C5b-9 to directly kill Gram-negative bacteria [Citation10,Citation11]. Microbes have developed several mechanisms to disrupt the stringent cascade network of complement system to survive in the host. The bacterial complement evasion strategies are divided into five groups: (a) mimicking of complement regulatory proteins like the CD-59 like protein in B. burgdorferi [Citation12]; (b) secretion of proteases to cleave complement components like C3-degrading protein SpeB in S. pyogenes [Citation13]; (c) complement exploitation for subverting the host response like the inhibition of TLR2 activity by inducing CR3 signaling on macrophages by F. tularensis [Citation14]; (d) secretion of small bacterial proteins with complement inhibitory properties [Citation15]; (e) recruiting of fluid phase complement regulatory proteins C1-INH [Citation16], C4BP [Citation17] and fH [Citation18]. Over the past 20 years, a number of virulence factors are found to contribute to S. suis invasion of the epithelial barrier, immune evasion and persistence in the bloodstream and multiple host organs [Citation3,Citation19]. Complement evasion factors expressed by S. suis include fH-binding protein [Citation18], SntA [Citation20], IdeSsuis [Citation21], and Suilysin [Citation22] targeting fH, C1q, IgM, C3aR, and C5aR, respectively.
The complement activation products C5a and C3a are chemoattractant and anaphylatoxin with potent biological activities and are involved in recruitment of inflammatory cells, generation of oxidative burst, modulation of opsonophagocytosis, releasing of cytokines through the high-affinity C5a receptors (C5aR and C5L2) and C3aR receptors (C3aR) [Citation23,Citation24]. Two subtilisin-like serine protease (SspA-1 and SspA-2) are identified in S. suis SC-19. In the previous study, it has been reported that one (SspA-2 in this study) of them is secreted through type IV secretion system [Citation25], triggers an excessive inflammatory response [Citation26] and plays a key role in pathogenesis in mouse and pig models [Citation27,Citation28], while the research of the other one is less. The truncated SspA-1 is identified a C5a-binding partner when we screen the C5a-binding proteins in its host. In the present study, SspA-1 and SspA-2 both interact with C3a and C5a, play an important role in complement evasion and pathogenesis of S. suis.
Materials and methods
Bacterial strains, cells, and culture conditions
S. suis serotype 2 strain SC-19 (GenBank accession number: CP020863.1) used in this study was isolated from a sick pig during the epidemic outbreak in Sichuan province of China in 2005. The S. suis strains were grown in tryptic soy broth (TSB; Difco, France) or on tryptic soy agar (TSA; Difco) plates supplemented with 5% newborn bovine serum (Sijiqing, Hangzhou, China) at 37 °C. The Escherichia coli (E. coli) DH5α and Rosseta (DE3) strains were grown in LB broth or on LB agar plates at 37 °C with rotation. The bacterial strains, plasmids and primers used in this study are listed in . The human acute monocytic leukaemia cell line (THP-1) was grown in RPMI 1640 medium (Gibco, USA) supplemented with 10% foetal calf serum (FCS; Gibco), 10 U/mL penicillin/streptomycin (Gibco). The human embryonic kidney 293T cell line (HEK-293T) was grown in DMEM medium (Gibco) supplemented with 10% FCS, 10 U/mL penicillin/streptomycin. All the cells were maintained in 5% CO2 at 37 °C.
Table 1. Bacterial strains, plasmids, and primers used in this study.
Bacterial two-hybrid assay
To identify complement binding proteins (CBPs), the Bacterial two-hybrid (B2H) screening assay using the BacterioMatch® II Two-Hybrid System Library Construction Kit (Stratagene, USA) was performed according to our published method [Citation22]. To construct prey library of B2H system, the surface-bound and secreted proteins coding genes of S. suis SC 19 were amplified from its genome and cloned into pTRG plasmid. To construct complement bait of B2H system, the mouse c3a (mc3a; GeneBank accession number: NM_009778) genes were amplified from mouse brain total cDNA (Clontech, USA) using the primers listed in the and cloned into pBT plasmid. After self-activation tests, pairs of recombinant bait and prey plasmids were co-transformed into the B2H reporter strain E. coli XBM Kana strain. The transformants with the volume of 10 μL at mid-log phase were dripping on the non-selective, single selective and dual selective screening mediums to screen the interaction proteins. The transformants co-transformed with empty pBT and empty pTRG plasmids were served as a negative control. The positive strain from B2H System Library Construction Kit was served as a positive control. The experiments were repeated for three times.
Expression and purification of recombinant proteins
To express recombinant proteins, the truncated sspA-1T (481–2364 bp) and sspA-2T (676–2559 bp) genes were amplified from genomic DNA of S. suis SC-19 () using the primers listed in the , digested with restriction enzyme BamH I and Xho I and cloned into pET-28a plasmid. The human c3a (GeneBank accession number: K02765; PDB ID: 4HW5) and c5a (GeneBank accession number: M57729; PDB ID of NMR structure: 1KJS; PDB ID of crystal structure: 4P39) genes were amplified from human lung total cDNA (Clontech) using the primers pGEX-6P-1-c3a-F/R and pGEX-6P-1-c5a-F/R (), enzyme digested with EcoR I and Sal I and cloned into pGEX-6P-1 plasmid. Another human c3a and c5a fragments were amplified by pGEX-6P-1-c3a-F/pGEX-GFP-c3a-R and pGEX-6P-1-c5a_F/pGEX-GFP-c5a-R, respectively. The fragments of gfp gene were amplified by GFP-c3a-F/GFP-R for c3a and GFP-c5a-F/GFP-R for c3a, respectively. The fragments of c3a-gfp and c5a-gfp amplified by overlapping PCR using pGEX-6P-1-c3a-F/GFP-R and pGEX-6P-1-c5a-F/GFP-R, respectively, enzyme digested with EcoR I and Xho I, cloned into pGEX-6P-1. The recombinant plasmids were transformed into E. coli Rosseta (DE3) competent cells (Tsingke, Beijing, China). The resulting E. coli cells at mid-log phase were induced by 1 mmol/L isopropyl-β-d-thiogalactoside (IPTG) (Sangon, Shanghai, China) at 16 °C for 16 hours to express recombinant proteins. His-tagged proteins expressed by pET-28a-sspA-1T and pET-28a-sspA-2T were purified by Ni-nitrilotriacetic acid (Ni-NTA) resin (Sigma, USA) affinity chromatography, while GST-tagged proteins expressed by pGEX-6P-1-c3a and pGEX-6P-1-c5a were purified by Glutathione Sepharose resins (Sigma). All the proteins were purified by applying gravity-assisted flow in disposable columns. Removal of GST-tag from GST-tagged proteins was performed by PreScission Protease (Yeasen, Shanghai, China) and also purified by Glutathione Sepharose resins. The purified proteins were quantified by BCA protein assay kit (Beyotime, Shanghai, China). Proteins were applied to 10% SDS-PAGE and transferred onto polyvinylidene difluoride (PVDF) membrane (Millipore, 0.45 μm). Rabbit anti-His (1:1000, CST, USA) polyclonal and mouse anti-GST (1:1000, CST) monoclonal antibodies were applied to immunoblotting separately and analysed by chemiluminescence detection (Bio-Rad, USA) with the corresponding HRP-conjugate goat anti-rabbit IgG (1:10000, Bioker, Hangzhou, China) or HRP-conjugate goat anti-mouse IgG (1:10000, Bioker) antibody by Miniche i910 (Sinsage, Beijing, China).
Immunofluorescence and confocal microscopy
To generate N-terminal Myc-tagged c3a and c5a expression constructs, c3a and c5a genes were amplified using the primers listed in the , digested with restriction enzyme EcoR I and Xho I, and cloned into the pCMV-Myc plasmid. To generate N-terminal HA-tagged sspA-1 and sspA-2 expression constructs, truncated sspA-1T and sspA-2T genes were amplified by pCMV-sspA-1T-F/R and pCMV-sspA-2T-F/R, respectively, enzyme digested with Xho I and Kpn I and cloned into the pCMV-HA plasmid. Pairs of eukaryotic expression plasmids pCMV-HA-sspA-1T or pCMV-HA-sspA-2T and pCMV-Myc-c3a or pCMV-Myc-c5a were co-transfected into HEK-293T cells. After grown in 24 h, the cells were washed with 10 mM PBS, fixed with 4% paraformaldehyde for 30 min, permeabilized with 0.5% Triton X-100 in PBS for 10 min. After additional washes in PBS, cells were applied with rabbit anti-HA (1:1000, Invitrogen, USA) and mouse anti-Myc antibodies (1:1000, Invitrogen) at the ratio of 1:1 at 37 °C for 30 min for double-labelling, followed by Alexa Fluor 594-conjugated anti-rabbit (1:1000, Invitrogen) and 488-conjugated anti-mouse (1:1000, Invitrogen) antibodies at the ratio of 1:1 at 37 °C for 45 min. Nuclei were visualized with DAPI (Beyotime), and cells were imaged by confocal microscopy (Olympus FV3000 confocal microscope, Japan).
Co-immunoprecipitation assay
Eukaryotic expression plasmid pCMV-HA-sspA-1T or pCMV-HA-sspA-2T with pCMV-Myc-c3a or pCMV-Myc-c5a was transfected into HEK-293T cells separately and in pair using jetPRIME reagent (Polyplus, France). After grown in 24 h, cells were lysed in HEK-293T RIPA lysis buffer (Beyotime, Shanghai, China) containing protease inhibitors. The mixtures of 20 μg cell lysates supernatants and 4 × SDS-PAGE loading buffer with total volume of 16 μL were used as input samples to detect protein expression in HEK-293T cells. For co-immunoprecipitation (Co-IP), the total proteins of cell lysates supernatants were quantified to 1 μg/μL, approximately 200 μL of transfected or co-transfected cell lysates were incubated with Myc-tagged antibody-coupled protein A/G plus agarose beads (Invitrogen) at 4 °C for 2 hours, washed with PBS for five times and eluted with 40 μL 1 × SDS-PAGE loading buffer. The elution mixtures were used as Co-IP samples. All the samples were applied to 10% SDS-PAGE and transferred onto PVDF membrane. Rabbit anti-HA (1:1000), mouse anti-Myc (1:1000) and β-Tubulin (1:1000, CST) antibodies were applied to immunoblotting separately and analysed by chemiluminescence detection with the corresponding HRP-conjugate goat anti-rabbit IgG or HRP-conjugate goat anti-mouse IgG (1:10000, Bioker) antibody by Miniche i910.
Far-western blotting assay
To confirm the interactions between SspA-1, SspA-2 and C3a, C5a, the far-western blotting assays were performed [Citation22,Citation30]. Briefly, 8 μg recombinant SspA-1T, SspA-2T and BSA served as a negative control were applied to 12% SDS-PAGE. Proteins were transferred onto PVDF membrane. HEK-293T cells harbouring pCMV-Myc-c3a or pCMV-Myc-c5a were lysed, poured onto PVDF membrane with a concentration of 10 μg/mL and incubated at 37 °C for 2 hours. After washed, mouse anti-Myc (1:1000) antibody was applied for Myc_C3a and Myc_C5a immunodetection. Chemiluminescence detection with HRP-conjugate goat anti-mouse IgG was performed by Miniche i910.
Enzyme-linked immunosorbent assay
ELISA was used to analyse the interaction of SspA-1, SspA-2 with C3a, C5a protein as described previously [Citation20]. For C3a and C5a bound assay, 0–7 μg/mL SspA-1T, SspA-2T and BSA as a negative control were immobilized to 96-well plate (Corning, USA), 5 μg/mL C3a and C5a were applied after blocking with 1% BSA in PBST (PBS with 20% Tween-20), respectively. Mouse C3a/C3a des Arg monoclonal antibody (Abcam, England) or rabbit C5a monoclonal antibody (Abcam) was applied at 37 °C for 1 hour to test the direct binding capacity. For competitive binding assay, 5 μg/mL SspA-1T, SspA-2T and BSA were immobilized to 96-well plate, 5 μg/mL C5a combined with 0–20 μg/mL C3a were applied after blocking with 1% BSA in PBST, respectively. Mouse anti-C5 monoclonal antibody (Proteintech, Wuhan, China) was applied at 37 °C for 1 hour to test the C5a binding capacity. The HRP-conjugate goat-anti-rabbit IgG (CST) or HRP-conjugate rabbit-anti-mouse IgG (CST) was used as the second antibody at 37 °C for 1 h before Chromogenic reaction by TMB Chromogenic Reagent kit (Sangon) and detected by SynergyTM H1 Hybrid Reader (Biotek, USA). These data were presented as means ± standard deviations (SD) from three replicates. The experiments were repeated for three times.
Cleavage assay and visualization by western blotting
Considering the proteolytic activity of serine proteases SspA-1 and SspA-2, the cleavage experiments were performed as the previous study [Citation31]. S. suis 2 SC19, ΔsspA-1, ΔsspA-2 and ΔsspA-1/2 at stationary phase (4 × 106 CFU) were washed twice in sterile PBS and incubated with GST_C3a_GFP (0.5 μg) or GST_C5a_GFP (0.5 μg) in TSB in a final volume 10 μL. All samples were incubated for 6, 12 and 16 hours, 37°C, 5% CO2. The mixtures were centrifuged and resulting supernatants analysed. Samples were applied on 12% SDS-PAGE and transferred onto PVDF membrane. Rabbit anti-complement C3 (with GST tag) monoclonal Ab (Abclonal, Wuhan, China) and mouse anti-C5 (with GST tag) monoclonal antibodies (Proteintech) were applied for C3a and C5a immunodetection, respectively. Chemiluminescence detection with HRP-conjugate goat anti-rabbit IgG and HRP-conjugate goat anti-mouse IgG was performed by Miniche i910.
Chemotaxis assay
To investigate the effect of recombinant SspA-1 and SspA-2 on chemotaxis of THP-1 cells towards C3a and C5a, the chemotaxis assays were performed as our previous study [Citation22]. 100 nM SspA-1T or SspA-2T with 10 nM C3a or C5a in RPMI 1640 medium were added to the lower chamber of a Transwell System (Neuro Probe, USA). 100 μL THP-1 cells (2 × 106 cells/mL) were added to the upper chamber of the Transwell System. After migration for 90 min in 5% CO2 at 37 °C, the migrated cells containing attached cells were observed by inverted fluorescence microscope (Olympus, Japan) and calculated. These data were presented as means ± standard deviations (SD) from three replicates. The experiments were repeated three times.
Construction of single and double gene deletion strains
The sspA-1 gene deletion strain ΔsspA-1 was constructed via a two-step procedure as described previously [Citation32]. In the first step, upstream and downstream arms of sspA-1, SCIY cassette were amplified using primers sspA-1-P1/P2, sspA-1-P5/P6, and sspA-1-P3/P4, respectively. These three DNA fragments were fused into a fragment using overlapping PCR with primers sspA-1-P1/P6 and transformed into S. suis SC-19 by natural transformation to obtain the intermediate strain. In the second step, upstream and downstream arms of sspA-1 were amplified using primers sspA-1-P1/P7 and sspA-1-P8/P6, respectively. These two DNA fragments were fused with primers sspA-1-P1/P6. The fused DNA fragment was transformed into the intermediate strain by natural transformation to obtain markerless sspA-1 gene deletion strain. The sspA-2 single gene and sspA-1/2 double genes deletion strains ΔsspA-1 and ΔsspA-1/2 were constructed according to the method as described above. All the gene deletion strains were verified by genomic PCR and RT-PCR with primes listed in .
Bactericidal assays
Experiment to investigate survival rates of S. suis in swine blood and mouse polymorphonuclear leukocytes (PMNs) were carried out as described previously [Citation20]. In swine blood killing assay, 50 μL S. suis strains cultures (5 × 107 CFU/mL) were mixed with 450 μL fresh swine blood and incubated at 37 °C for 0.5 and 1 hour. Live bacteria were counted by plating the serial diluted samples on TSA agar plates. The percentage of live bacteria was subsequently calculated as (CFU after incubation/CFU in original inoculum) × 100%. In opsonophagocytosis assay, PMNs were isolated from heparinized venous blood by mouse peripheral blood PMNs isolation kit (Haoyang, Tianjin, China). PMNs were mixed with bacteria at MOI = 1:10 in RPMI-1640 (Hyclone, USA) supplemented with 20% fresh non-immune mouse serum and incubated at 37°C under 5% CO2 for 30 min. The percentage of live bacteria was subsequently calculated as (CFU PMN+/CFU PMN-) × 100%. These data are presented as means ± standard deviations (SD) from three separate replicates. The experiments were repeated for three times.
Galleria mellonella larvae and mice infection assays
To detect the virulence of sspA-1 and sspA-2 in S. suis, Galleria mellonella (G. mellonella) larvae model was performed as described previously [Citation33]. A total of 50 G. mellonella larvae (10 per group) were injected at the left posterior proleg with 1 × 106 CFU of S. suis. Physiological saline was served as a negative control. The survival of G. mellonella larvae was recorded at 12 h intervals for 7 days. To evaluate the effect of sspA-1 and sspA-2 on survival in the bloodstream and colonization of distant organs in mice, a total of 56 female 5-week-old specific-pathogen-free (SPF) BALB/c mice (7 mice per group) were intraperitoneally infected with 1 × 108 CFU/mouse of S. suis. Bacterial counts in blood, brain, kidney, and lung were collected at 12 and 24 hours post infection (hpi). Colonization of bacteria in various tissues was analysed on TSA agar plates as described previously [Citation20].
Results
Bioinformatics analyses of subtilisin-like serine proteases SspA-1 and SspA-2
Two open reading frames (ORFs) of genes (B9H01_RS03990 and B9H01_RS09530) were annotated as subtilisin-like serine protease (SspA) in the S. suis SC-19 genome. Comparative analysis of these genes across multiple virulent strains, including S. suis P1/7, SC070731, 05ZYH33, 98HAH33 and SC84, and BM407, revealed distinct amino acids sequences. Notably, the first SspA-1 of gene in S. suis P1/7 encoded 1585 amino acids, whereas the corresponding gene in SC-19 comprised only 1270 amino acids (). Additionally, a cytosine deletion-induced stop codon led to the misannotation of the gene encoding SspA-1 as two ORFs, B9H01_RS03985 and B9H01_RS03990 in SC-19 (). A similar cytosine deletion was observed in the ORF of sspA-1 across S. suis strains 05ZYH33, SC84, 98HAH33 and BM407, all of which were derived from human cases and were not present in the corresponding genes of strains isolated from pigs with acute septicaemia, such as S. suis HA9801 and ZY05719. So, two subtilisin-like serine proteases were identified as SspA-1 (Protein ID: ARL69708.1 and ARL70750.1) and SspA-2 (Protein ID: ARL69708.1). Both subtilisin-like proteinases SspA-1 and SspA-2 and their truncated proteins SspA-1T and SspA-2T contained two domains: Peptidase_S8_family Streptococcal C5a peptidase domain and fibronectin type-III domain, and cell wall-anchored motif LPXTG at the C-terminus (). Comparison of amino acid through BLAST revealed substantial identity of SspA-1 with known Streptococcal C5a peptidase S. pyogenes ScpC (46.98%). SspA-1 shared significant identity with S. agalactiae ScpB, S. pyogenes ScpA, S. equisimilis ScpA/B, S. iniae ScpI and S. zooepidemicus ScpZ (above 30%) (). In contrast, SspA-2 displayed limited similarity with known Streptococcal C5a peptidase (below 30%, ), including SspA-1 of SC-19. No similarity was observed in Mycobacterium tuberculosis (M. tuberculosis) C5a peptidase with SspA-1 and SspA-2 (). Multiple Sequence Alignments by MEGA6.06 revealed that both SspA-1 and SspA-2 contain the classical Ser/His/Asp catalytic triad of serine proteases (). Thus, S. suis SC-19 harboured two subtilisin-like serine proteases, SspA-1 and SspA-2, sharing analogous domains but possessing low sequence identity.
Figure 1. Bioinformatics analysis of S. suis SspA-1 and SspA-2. (a) Diagrams illustrating the structure features of SspA-1 and SspA-2. Both SspA-2 and SspA-1 contained subtilisin-like serine peptidase typical domains: peptidase S8 family Streptococcal C5a peptidases domain (Peptidase_S8, SspA-1_Peptidase_S8: 156-638 a.a., SspA-2_Peptidase_S8: 237-722 a.a.), fibronectin type-III domain (fn3_like, SspA-1_fn3_like: 652-762 a.a., SspA-2_ fn3_like: 737-852 a.a.), protease associated (PA) domain located at Peptidase_S8 domain existed in proteins like Streptococcus pyogenes C5a peptidase and cell wall-anchored motif LPXTG at the end of C-terminus. SP: signal peptide. Truncated SspA-1T and SspA-2T were showed as red colour. (b) Representation of SspA-1 coding genes, B9H01_RS03985 and B9H01_RS03990 in S. suis SC-19. Incorrect annotation of the gene encoding the SspA-1 occurred due to a cytosine deletion causing a premature stop codon, resulting in the emergence of two ORFs: B9H01_RS03985 and B9H01_RS03990, in S. suis SC-19, distinct from SSU_RS009530 in S. suis P1/7. (c) Multiple Sequence Alignment of SspA-1 and SspA-2 with known Streptococcal C5a peptidases. The sites of Ser/His/Asp catalytic triad are marked above using asterisks (*).
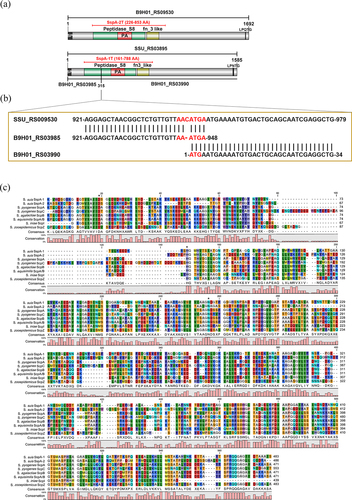
Table 2. Sequence identity alignment of the amino acid (a.a.) sequences of S. suis SC-19 SspA-1 with known C5a proteases from other species.
Table 3. Sequence identity alignment of the a.a. sequences of S. suis SC-19 SspA-2 with known C5a proteases from other species.
SspA-1 and SspA-2 co-localize intracellularly with human C3a and C5a
To examine the co-localization of SspA-1 and SspA-2 with human C3a and C5a, plasmids encoding HA_SspA-1T or HA_SspA-2T with Myc_C3a or Myc_C5a, were co-transfected into HEK-293T cells and visualized through confocal microscopy. Within the cytoplasm, HA_SspA-1T (red), HA_SspA-2T (red), Myc_C3a (green) and Myc_C5a (green) exhibited widespread distribution. Co-localization of HA_SspA-1T or HA_SspA-2T with Myc_C3a or Myc_C5a was revealed by overlapping of signals, resulting in yellow staining (). These findings demonstrated complete co-localization of SspA-1 and SspA-2 with C5a and C3a, respectively, emphasizing their intricate interplay within the cellular environment.
Figure 2. Intracellular co-localization of SspA-1 and SspA-2 with C3a and C5a protein. Immunofluorescence images depicting HA_SspA-1T (red), HA_SspA-2T (red), Myc_C3a (green) and Myc_C5a (green) proteins within HEK-293T cells. The nucleus is marked with DAPI staining (blue). Evident co-expression of SspA-1T or SspA-2T with C3a or C5a in the cytoplasm is observed in merged images. Co-localization is affirmed by the convergence of signals, manifesting as overlapping yellow regions.
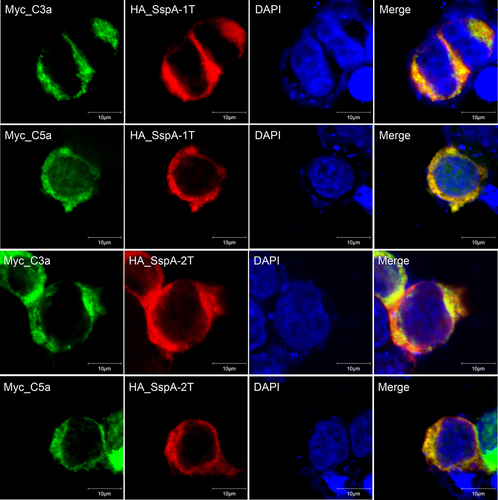
SspA-1 and SspA-2 interact with human C3a and C5a
Truncated SspA-1 was screened to be C3a bind protein by B2H from S. suis surface and secreted proteins prey library (Figure S1). Truncated His_SspA-1T (74 KDa) and His_SspA-2T (74 KDa), GST_C5a (34 KDa) and GST_C3a (35 KDa) were purified and detected through SDS-PAGE and Western blotting with anti-His and anti-GST antibodies, respectively (Figure S2). To investigate the protein-protein interaction between SspA-1 and SspA-2, and human C3a and C5a, a combination of Co-IP, ELISA and far-western blotting experiments was performed. The Co-IP assay affirmed the capability of both SspA-1T and SspA-2T to co-immunoprecipitate with C3a and C5a (). No proteins were detected in control lysates from cells transfected with a single Myc_C3a or Myc_C5a plasmid. Concurrently, ELISA validation confirmed the dose-dependent binding of SspA-1T and SspA-2T to C3a and C5a on SspA-1T-coated and SspA-2T-coated plates (). Furtherly, C5a combined with increasing concentrations of C3a were applied to SspA-1T- and SspA-2T-coated plates to perform the competitive binding assay. Results showed that C3a and C5a could not competitively bind with SspA-1T and SspA-2T (). Besides, far-western blotting confirmed the obvious interaction between eukaryotic expression C3a, C5a with SspA-1T, SspA-2T (). Taken together, these results confirmed the interactions of both SspA-1 and SspA-2 with human C3a and C5a, laying their role in modulating the immune response.
Figure 3. Interaction of SspA-1 and SspA-2 with complement C3a and C5a. The interaction between SspA-1 (a) or SspA-2 (b) and C3a and C5a was investigated using Co-Immunoprecipitation (Co-IP). HA_SspA-1T or HA_SspA-2T was co-transfected with Myc_C3a or Myc_C5a into HEK-293T cells, precipitated with anti-Myc antibody and immunoblotting with HA antibody to verify the protein-protein interaction. β-Tubulin served as a negative control. Protein extracts (Input) underwent SDS-PAGE after immunoprecipitation with HA or Myc antibody. To investigate C3a (c) and C5a (d) binding capacity and competitive binding capacity (e), ELISA experiments were conducted on SspA-1T-, SspA-2T-, and BSA-coated plates, with subsequent application of C3a or C5a. Recombinant SspA-1T and SspA-2T were applied to 10% SDS-PAGE and transferred onto PVDF membrane. The PVDF membrane was incubated with lysate of HEK-293T cell harbouring pCMV-Myc-c3a (f) or pCMV-Myc-c5a (g) and probed with an anti-Myc antibody for immunodetection. Statistical significance was determined by two-way ANOVA of Sidak’s correct for multiple comparison and indicated using asterisks (***p < 0.001).
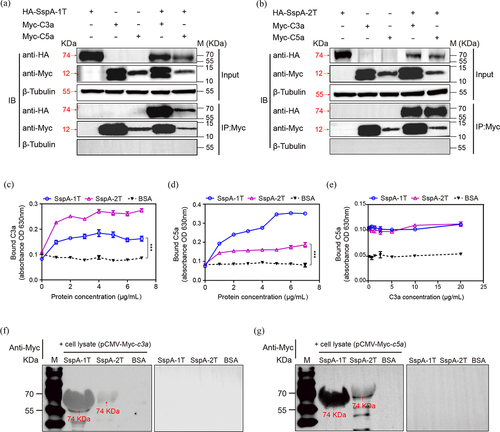
SspA-1 and SspA-2 cannot cleave C3a or C5a
Considering the proteolytic activity of serine proteases SspA-1 and SspA-2, the cleavage experiments with S. suis strains were performed to investigate the cleavage activity of SspA-1T and SspA-2T against C3a and C5a. Results showed that no obvious cleavage activity was observed in SC 19, and no significant difference among that wild-type SC 19 with the mutant strains ΔsspA-1, ΔsspA-2 and ΔsspA-1/2 (). Unfortunately, these results revealed that SspA-1T and SspA-2T may have no cleavage activity against C3a and C5a.
Figure 4. S. suis strains at stationary phase (4 × 106 CFU) were incubated with GST_C3a_GFP (0.5 μg) or GST_C5a_GFP (0.5 μg) in TSB for 6, 12 and 16 hours. Protease inhibitor cocktail in GST_C3a_GFP or GST_C5a_GFP without S. suis strains was used as a negative control. Monoclonal antibodies specific for C3 or C5 were applied for C3a and C5a immunodetection, respectively.
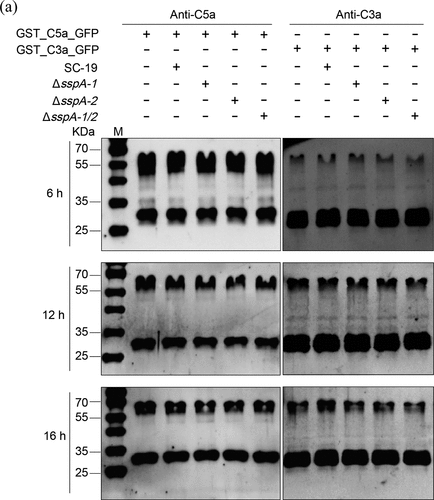
SspA-1 and SspA-2 suppress the chemotaxis of THP-1 cells
Complement fragments C3a and C5a were the potent and important chemokine involved in recruitment of immune cells [Citation23,Citation24]. In order to probe the influence of recombinant SspA-1 and SspA-2 on chemotactic activity mediated by human chemokine C3a and C5a, chemotaxis assays were performed on THP-1 cells. The findings showed that the number of THP-1 cells chemotactic towards C3a and C5a were significantly decreased upon addition of 100 nM recombinant SspA-1T or SspA-2T (). Hence, the outcomes indicated that both recombinant SspA-1 and SspA-2 exerted a significant inhibitory impact on the chemotactic activity driven by human C3a and C5a, underscoring their potential in modulating immune cell response, albeit without proteolytic activity against C3a and C5a.
Figure 5. Inhibition of THP-1 cell chemotaxis by recombinant SspA-1 and SspA-2. C3a or C5a was incubated with SspA-1 or SspA-2 to test the effect of SspA-1T (a) and SspA-2T (b) on chemotaxis by chemotaxis assay. Specifically, 10 nM C5a or C3a was incubated with 100 nM SspA-1 or SspA-2 for 30 min at 4 °C, and subsequently introduced into the lower chamber of a Transwell system. The upper chamber contained THP-1 cells to facilitate migration. The data were represented as means ± standard deviations (SD) from three independent experiments. Statistical significance was determined by two-way ANOVA of Sidak’s correct for multiple comparison and indicated using asterisks (***p < 0.001).
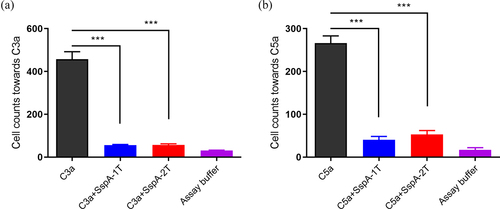
SspA-1 and SspA-2 exert obvious survival activities in blood and neutrophils
Interference of C3a and C5a resulted in disruption of human neutrophil activation, opsonophagocytosis and chemotaxis [Citation24,Citation31]. Through the creation and validation of markerless single gene deletion strains ΔsspA-1 and ΔsspA-2, as well as the double gene deletion strain ΔsspA-1/2, via genomic PCR and RT-PCR (Figure S3), the blood killing and opsonophagocytosis assays were performed to investigate the survival capacity in blood and susceptibility in PMNs with serum of S. suis mediated by SspA-1 and SspA-2. In the blood killing assay, the survival rates of SC-19 in swine blood were 128% and 114% following 0.5 and 1 hour of incubation. In contrast, the survival rates of ΔsspA-1, ΔsspA-2 and ΔsspA-1/2 exhibited significant reductions to 69% and 84%, 98% and 93%, 82% and 84% after 0.5 and 1 hour of incubation, respectively (). In opsonophagocytosis assay, PMNs supplemented with 20% active mouse serum were used to test the survival abilities of S. suis in mouse neutrophils. Results revealed that the survival rates of ΔsspA-1, ΔsspA-2 and ΔsspA-1/2 were significantly decreased 19.21%, 28.42% and 17.38% compared to SC-19 (). Collectively, these observations suggested the involvement of SspA-1 and SspA-2 in conferring resistance to the opsonophagocytosis activity of blood and PMNs, contingent upon the presence of complement.
Figure 6. Survival activity of S. suis in blood and PMNs. (a) Survival rate of S. suis in swine blood was assessed. (b) Survival rate of S. suis in PMNs in the presence of active serum was evaluated. The data were represented as means ± standard deviations (SD) from three independent experiments. Statistical significance was determined by two-way ANOVA of Sidak’s correct for multiple comparison and indicated using asterisks (***p < 0.001; **p < 0.01; *p < 0.05; ns, p > 0.05).
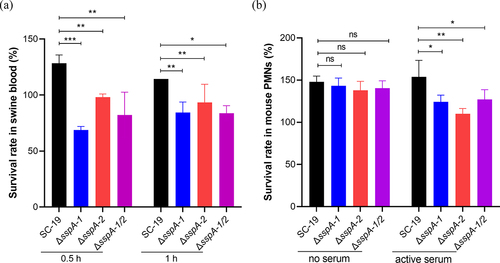
SspA-1 and SspA-2 contribute to the virulence of S. suis
Furtherly, the role of sspA-1 and sspA-2 in the virulence of S. suis was examined through infection models involving Galleria mellonella (G. mellonella) larvae and mouse. Initially, groups of 10 G. mellonella larvae were injected with 1 × 106 CFU S. suis SC-19, ΔsspA-1, ΔsspA-2, or ΔsspA-1/2 via the left posterior proleg, while physiological saline was used as a negative control. Mortality outcomes demonstrated that within one day-post infection (dpi), 8 larvae infected with SC-19 had perished, with an additional 2 died by 2 dpi. In contrast, only 2, 5, and 0 larvae infected with ΔsspA-1, ΔsspA-2, and ΔsspA-1/2 succumbed within one dpi. The survival rates of larvae at 7 dpi were 0%, 70%, 30%, and 70% for SC-19, ΔsspA-1, ΔsspA-2, and ΔsspA-1/2, respectively (). These findings revealed that mortality of G. mellonella larvae infected with ΔsspA-1, ΔsspA-2, and ΔsspA-1/2 were significantly reduced when compared with the parental SC-19.
Figure 7. Impact of SspA-1 and SspA-2 on pathogenesis in murine and G. mellonella larvae infection models. (a) Survival curves of G. mellonella larvae challenged with S. suis. Larvae groups (n = 10) were administered with 1 × 106 CFU of S. suis SC-19, ΔsspA-1, ΔsspA-2, or ΔsspA-1/2. Physiological saline served as a negative control. Log Rank test for trend was employed to assess survival discrepancies between groups. Bacterial loads in blood (b), brain (c), lung (d) and kidney (e) were determined. Seven mice per group were intraperitoneally infected with mixtures of S. suis at 1 × 108 CFU/mouse. Results were shown as log10 of recovered bacterial counts after discarding the highest and lowest values (CFU/mL in Blood and CFU/g in organs). Statistical significance was determined by two-way ANOVA of Sidak’s correct for multiple comparison and indicated using asterisks (***p < 0.001; **p < 0.01; *p < 0.05).
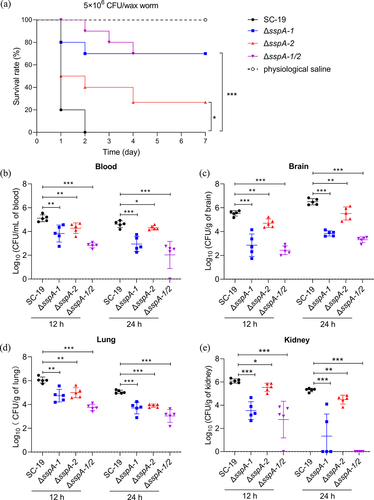
Subsequently, groups of 7 mice were intraperitoneally infected with SC-19, ΔsspA-1, ΔsspA-2, and ΔsspA-1/2. Blood and brain, lung, kidney colonization was assessed at 12 and 24 hours post infection (hpi). Notably, the colonization efficiency of all gene deletion strains, including ΔsspA-1, ΔsspA-2 and ΔsspA-1/2, exhibited marked reductions in comparison to SC-19 in blood (), brain (), lung () and kidney () at both 12 and 24 hpi, with ΔsspA-1/2 displaying the most pronounced attenuation. In summary, these results indicated that SspA-1 and SspA-2 played integral roles in virulence and survival within blood, and colonization within specific organs.
Discussion
S. suis is an important swine bacterial pathogen that can be transmitted to humans, the leading cause of severe systemic diseases such as meningitis, septicaemia with sudden death in piglets and STSS first reported in humans in 2005 [Citation6,Citation34]. Recently, plenty of virulence factors or traits consisting of surface-associated and secreted proteins, regulators and metabolic pathways have been demonstrated to enable S. suis to successfully colonize, invade, and disseminate in its host during infection [Citation35,Citation36]. However, how S. suis escapes the immune surveillance to survive in blood and most organs is largely unknown. In this study, we found that the cell wall-anchored subtilisin-like serine proteases SspA-1 and SspA-2 contribute to the inhibition of monocytes chemotaxis, the susceptibility in blood and PMNs, survival in blood and organs in vivo of the zoonotic pathogen S. suis, and this may be attributed to the interaction between SspA-1 and SspA-2 with C5a and C3a.
The complement C5a and C3a are involved in diverse processes of recruiting inflammatory cells, producing oxidative burst and releasing cytokines [Citation23,Citation24]. The bacterial surface-associated and secreted proteins are considered to contribute to S. suis interaction with host factors and cells in host infection [Citation37]. Here, C3a-binding partners were screened using B2H prey library of 57 proteins containing surface-associated and secreted proteins [Citation22]. Truncated SspA-1 coding gene B9H01_RS03990 was screened (Figure S2). In the present study, co-localization, Co-IP, ELISA and far-western blotting experiments have been performed to confirm the the co-localization and interaction between SspA-1T and SspA-2T with C3a and C5a. These results show that SspA-1 and SspA-2 can co-localize and substantially bind with C3a and C5a in vitro and in vivo (). Concerning the proteolytic activity of serine proteases SspA-1 and SspA-2, the cleavage activities of SspA-1T and SspA-2T were performed in TSB in vitro and detected with western blotting. Unfortunately, no obvious cleavage activity was observed among all the S. suis wild-type and mutant strains (). Although SspA-1T and SspA-2T contains the important domains of their full-length proteins SspA-1 and SspA-2, yet it also has the possibility that these intact proteases SspA-1 and SspA-2 could have proteolytic activities under different alternative in vitro and in vivo conditions as in the literature [Citation31,Citation38]. In this study, for SspA-2, this may be attributed to the limit similarities among SspA-2 with all the known Streptococcal C5a peptidases (<30%, ). For SspA-1, although the similarities among SspA-1 with all the known Streptococcal C5a peptidases were not bad (>30%, ), the cytosine deletion observed in the ORF of sspA-1 may disrupt the cleavage activity of intact SspA-1 against C3a and C5a, and have no influence in the interaction between truncated SspA-1 with C3a and C5a (Figure S2). A similar cytosine deletion was observed in the ORF of sspA-1 across S. suis strains 05ZYH33 [Citation39], SC84 [Citation29], 98HAH33 [Citation39], and BM407 [Citation29], all of which were interestingly derived from human cases, underscoring its potential in human infection and deserved to be further study.
In the previous study, the Group A Streptococcus (GAS) C5a peptidase ScpA cleaves C5a and C3a and reduce human neutrophil activation, phagocytosis and chemotaxis [Citation31]. Group B Streptococcus C5a peptidase ScpB and GAS ScpC cleave C5a and chemokine CXCL8, respectively [Citation40,Citation41]. ScpA, ScpB and ScpC are subtilisin-like serine proteases that evade the innate immune response and act as essential virulence factors, whether SspA-1 and SspA-2 without proteolytic activity against C3a and C5a could contribute to the chemotaxis and phagocytosis which also belong to subtilisin-like serine proteases. Results performed by chemotaxis assays show that the recombinant protein SspA-1 and SspA-2 can significantly inhibit the THP-1 cells chemotaxis towards C3a and C5a (), this may attributed to the interactions between SspA-1, SspA-2 and C3a, C5a, not because the proteolytic activity. S. pyogenes multifunctional protein Plr/SDH/GAPDH binds to C5a and inhibits C5a-activated chemotaxis and H2O2 production [Citation42]. Resveratrol binding to human complement fragment C5a (hC5a) may modulate the C5aR signalling axes [Citation43]. C5a Receptor Antagonist inhibits C5a-mediated chemotaxis of human PMNs, C5a-stimulated release of cytokines from human monocytes in vitro and induces inflammatory response of microglial cells [Citation44,Citation45]. S. suis Suilysin inhibits monocyte chemotaxis by interplaying with G protein-coupled receptors C3aR and C5aR [Citation22]. Thus, the interference of C5a/C5aR signalling pathways has potential to regulate the C5aR signalling axes and suppress the recruitment of immune cells. Simultaneously, SspA-1 and SspA-2 have been proved to contribute to the survival in whole blood and PMNs with serum in vitro with intact complement (). The survival factors of S. suis SC 19 in blood and PMNs were over 100%. Similarity results were observed in human, swine and mouse blood and PMNs of S. suis 2 in the previous study [Citation20,Citation46], suggesting that blood and PMNs reveal bactericidal effects on S. suis 2 resisted by some virulence-related factors at an early stage of infection and subsequent provide nutrition to grow. The unit gravity sedimentation rate of mouse bone marrow red blood cells (RBCs) was sharply defined to be ranged between 1.8 and 2.2 mm/hour, which is usually faster than that of white blood cells (WBCs) [Citation47], suggesting that bacterial contact of with PMNs tends to form a suspension during the blood killing and opsonophagocytosis assays within 1 hour.
In the previous study, SspA-2 secreted through type IV secretion system [Citation25] triggers a pro-inflammatory response in macrophages [Citation26] and contributes to virulence in pig [Citation27] and mouse infection model [Citation25]. In this study, the subtilisin-like proteases SspA-1 and SspA-2 promote complement evasion and facilitate S. suis survival in blood, lung, kidney and brain colonization in vivo (). To penetrate the blood-brain barrier (BBB) and invade the meninges and central nervous system (CNS), bacterial pathogens need to evade host innate immune defences including complement system, survive, and proliferate in intravascular to maintain a high degree of bacteraemia [Citation48]. This may indicate that the C3a- and C5a- binding proteins SspA-1 and SspA-2 May play roles in promoting S. suis to translocate over the BBB and colonize in brain. Because of the major role of the opsonin C3b in serum-mediated phagocytosis, SspA-1 and SspA-2 May also have the possibility to interfere C3b. Thus, two multifunctional serine proteases SspA-1 and SspA-2 May facilitate in S. suis pathogenesis with multiple mechanisms. In addition to that, there was also a number of bacterial proteins that mediated complement evasion strategies targeting at complements such as fH [Citation18], C1q [Citation20], C3 [Citation18], IgM [Citation21], C3aR, and C5aR [Citation22]. Thus, the role of complement evasion players in penetrating the BBB deserves to be further research.
In conclusion, the cell wall anchored subtilisin-like serine proteases SspA-1 and SspA-2 can interplay with complement C3a and C5a, inhibit the monocyte chemotaxis and opsonophagocytosis, and facilitate survival in blood and colonization in specific organs. These complement evasion strategies may be crucial for the pathogenesis of S. suis.
Supplemental Materialclean.docx
Download MS Word (1.5 MB)Disclosure statement
No potential conflict of interest was reported by the author(s).
Data availability statement
The data that support the findings of this study in this manuscript and its supplemental materials are available.
Supplemental data
Supplemental data for this article can be accessed online at https://doi.org/10.1080/21505594.2023.2301246.
Additional information
Funding
References
- Haas B, Grenier D. Understanding the virulence of Streptococcus suis: a veterinary, medical, and economic challenge. Med Mal Infect. 2018;48(3):159–17. doi: 10.1016/j.medmal.2017.10.001
- Segura M, Zheng H, de Greeff A, et al. Latest developments on Streptococcus suis: an emerging zoonotic pathogen: part 1. Future Microbiol. 2014;9(4):441–444. doi: 10.2217/fmb.14.14
- Tan C, Zhang A, Chen H, et al. Recent proceedings on prevalence and pathogenesis of Streptococcus suis. Curr Issues Mol Biol. 2019;32:473–520. doi: 10.21775/cimb.032.473
- Bleuze M, Gottschalk M, Segura M. Neutrophils in Streptococcus suis infection: from host defense to pathology. Microorganisms. 2021;9(11):2392. doi: 10.3390/microorganisms9112392
- Goyette-Desjardins G, Auger JP, Xu J, et al. Streptococcus suis, an important pig pathogen and emerging zoonotic agent-an update on the worldwide distribution based on serotyping and sequence typing. Emerg Microbes Infect. 2014;3(6):e45. doi: 10.1038/emi.2014.45
- Feng Y, Zhang H, Ma Y, et al. Uncovering newly emerging variants of Streptococcus suis, an important zoonotic agent. Trends Microbiol. 2010;18(3):124–31. doi: 10.1016/j.tim.2009.12.003
- Liu P, Pian Y, Li X, et al. Streptococcus suis adenosine synthase functions as an effector in evasion of PMN-mediated innate immunit. J Infect Dis. 2014;210(1):35–45. doi: 10.1093/infdis/jiu050
- Doran KS, Fulde M, Gratz N, et al. Host-pathogen interactions in bacterial meningitis. Acta Neuropathol. 2016;131(2):185–209. doi: 10.1007/s00401-015-1531-z
- Merle NS, Church SE, Fremeaux-Bacchi V, et al. Complement system part I - Molecular mechanisms of activation and regulation. Front Immunol. 2015;6:262. doi: 10.3389/fimmu.2015.00262
- Ghebrehiwet B. The complement system: an evolution in progress. F1000Res. 2016;5:2840. doi: 10.12688/f1000research.10065.1
- Laarman A, Milder F, van Strijp J, et al. Complement inhibition by gram-positive pathogens: molecular mechanisms and therapeutic implications. J Mol Med (Berl). 2010;88(2):115–20. doi: 10.1007/s00109-009-0572-y
- Pausa M, Pellis V, Cinco M, et al. Serum-resistant strains of borrelia burgdorferi evade complement-mediated killing by expressing a CD59-like complement inhibitory molecule. J Immunol. 2003;170(6):3214–22. doi: 10.4049/jimmunol.170.6.3214
- Honda-Ogawa M, Ogawa T, Terao Y, et al. Cysteine proteinase from Streptococcus pyogenes enables evasion of innate immunity via degradation of complement factors. J Biol Chem. 2013;288(22):15854–64. doi: 10.1074/jbc.M113.469106
- Dai S, Rajaram MV, Curry HM, et al. Fine tuning inflammation at the front door: macrophage complement receptor 3-mediates phagocytosis and immune suppression for Francisella tularensis. PLOS Pathog. 2013;9(1):e1003114. doi: 10.1371/journal.ppat.1003114
- Jongerius I, Kohl J, Pandey MK, et al. Staphylococcal complement evasion by various convertase-blocking molecules. J Exp Med. 2007;204(10):2461–2471. doi: 10.1084/jem.20070818
- Lathem WW, Bergsbaken T, Welch RA. Potentiation of C1 esterase inhibitor by StcE, a metalloprotease secreted by Escherichia coli O157: H7. J Exp Med. 2004;199(8):1077–87. doi: 10.1084/jem.20030255
- Cartwright K. Pneumococcal disease in western Europe: burden of disease, antibiotic resistance and management. Eur J Pediatr. 2002;161(4):188–95. doi: 10.1007/s00431-001-0907-3
- Li X, Liu P, Gan S, et al. Mechanisms of host-pathogen protein complex formation and bacterial immune evasion of Streptococcus suis Protein Fhb. J Biol Chem. 2016;291(33):17122–32. doi: 10.1074/jbc.M116.719443
- Fittipaldi N, Segura M, Grenier D, et al. Virulence factors involved in the pathogenesis of the infection caused by the swine pathogen and zoonotic agent Streptococcus suis. Future Microbiol. 2012;7(2):259–279. doi: 10.2217/fmb.11.149
- Deng S, Xu T, Fang Q, et al. The surface-exposed protein SntA contributes to complement evasion in zoonotic Streptococcus suis. Front Immunol. 2018;9:1063. doi: 10.3389/fimmu.2018.01063
- Seele J, Beineke A, Hillermann LM, et al. The immunoglobulin M-degrading enzyme of Streptococcus suis, IdeSsuis, is involved in complement evasion. Vet Res. 2015;46(1):45. doi: 10.1186/s13567-015-0171-6
- Deng S, Zhao L, Zhu J, et al. Complement C3aR/C5aR-binding protein Suilysin of Streptococcus suis contributes to monocyte chemotaxis. Vet Microbiol. 2020;242:108599. doi: 10.1016/j.vetmic.2020.108599
- Lo MW, Woodruff TM. Complement: bridging the innate and adaptive immune systems in sterile inflammation. J Leukocyte Biol. 2020;108(1):339–351. doi: 10.1002/JLB.3MIR0220-270R
- Ward PA, Fattahi F. New strategies for treatment of infectious sepsis. J Leukocyte Biol. 2019;106(1):187–192. doi: 10.1002/JLB.4MIR1118-425R
- Yin S, Li M, Rao X, et al. Subtilisin-like protease-1 secreted through type IV secretion system contributes to high virulence of Streptococcus suis 2. Sci Rep. 2016;6(1):27369. doi: 10.1038/srep27369
- Bonifait L, Grenier D. The SspA subtilisin-like protease of Streptococcus suis triggers a pro-inflammatory response in macrophages through a non-proteolytic mechanism. BMC Microbiol. 2011;11(1):47. doi: 10.1186/1471-2180-11-47
- Hu Q, Liu P, Yu Z, et al. Identification of a cell wall-associated subtilisin-like serine protease involved in the pathogenesis of Streptococcus suis serotype 2. Microb Pathog. 2010;48(3–4):103–9. doi: 10.1016/j.micpath.2009.11.005
- Bonifait L, de la Cruz Dominguez-Punaro M, Vaillancourt K, et al. The cell envelope subtilisin-like proteinase is a virulence determinant for Streptococcus suis. BMC Microbiol. 2010;10(1):42. doi: 10.1186/1471-2180-10-42
- Holden MT, Hauser H, Sanders M, et al. Rapid evolution of virulence and drug resistance in the emerging zoonotic pathogen Streptococcus suis. PloS One. 2009;4(7):e6072. doi: 10.1371/journal.pone.0006072
- Li Q, Liu H, Du D, et al. Identification of novel laminin- and fibronectin-binding proteins by Far-Western Blot: capturing the adhesins of Streptococcus suis type 2. Front Cell Infect Microbiol. 2015;5:82. doi: 10.3389/fcimb.2015.00082
- Lynskey NN, Reglinski M, Calay D, et al. Multi-functional mechanisms of immune evasion by the streptococcal complement inhibitor C5a peptidase. PLOS Pathog. 2017;13(8):e1006493. doi: 10.1371/journal.ppat.1006493
- Zheng C, Wei M, Qiu J, et al. A markerless gene deletion system in Streptococcus suis by using the Copper-Inducible Vibrio parahaemolyticus YoeB Toxin as a counterselectable marker. Microorganisms. 2021;9(5):1095. doi: 10.3390/microorganisms9051095
- Li H, Li T, Hu Q, et al. Inhibitors targeting the autophosphorylation of serine/threonine kinase of Streptococcus suis show potent antimicrobial activity. Front Microbiol. 2022;13:990091. doi: 10.3389/fmicb.2022.990091
- Wertheim HF, Nghia HD, Taylor W, et al. Streptococcus suis: an emerging human pathogen. Clin Infect Dis. 2009;48(5):617–25. doi: 10.1086/596763
- Gottschalk M, Xu J, Calzas C, et al. Streptococcus suis: a new emerging or an old neglected zoonotic pathogen? Future Microbiol. 2010;5(3):371–391. doi: 10.2217/fmb.10.2
- Segura M, Fittipaldi N, Calzas C, et al. Critical Streptococcus suis virulence factors: are they all really critical? Trends Microbiol. 2017;25(7):585–599. doi: 10.1016/j.tim.2017.02.005
- Baums CG, Valentin-Weigand P. Surface-associated and secreted factors of Streptococcus suis in epidemiology, pathogenesis and vaccine development. Anim Health Res Rev. 2009;10(1):65–83. doi: 10.1017/S146625230999003X
- Li H, Dang G, Liu H, et al. Characterization of a novel mycobacterium tuberculosis serine protease (Rv3194c) activity and pathogenicity. Tuberculosis (Edinb). 2019;119:101880. doi: 10.1016/j.tube.2019.101880
- Chen C, Tang J, Dong W, et al. A glimpse of streptococcal toxic shock syndrome from comparative genomics of S. suis 2 Chinese isolates. PloS One. 2007;2(3):e315. doi: 10.1371/journal.pone.0000315
- Jobichen C, Tan YC, Prabhakar MT, et al. Structure of ScpC, a virulence protease from Streptococcus pyogenes, reveals the functional domains and maturation mechanism. Biochem J. 2018;475(17):2847–2860. doi: 10.1042/BCJ20180145
- Chmouryguina I, Suvorov A, Ferrieri P, et al. Conservation of the C5a peptidase genes in group a and B streptococci. Infect Immun. 1996;64(7):2387–90. doi: 10.1128/iai.64.7.2387-2390.1996
- Terao Y, Yamaguchi M, Hamada S, et al. Multifunctional glyceraldehyde-3-phosphate dehydrogenase of Streptococcus pyogenes is essential for evasion from neutrophils. J Biol Chem. 2006;281(20):14215–23. doi: 10.1074/jbc.M513408200
- Mishra R, Das A, Rana S. Resveratrol binding to human complement fragment 5a ((h)C5a) may modulate the C5aR signaling axes. J Biomol Struct Dyn. 2021;39(5):1766–1780. doi: 10.1080/07391102.2020.1738958
- Haynes DR, Harkin DG, Bignold LP, et al. Inhibition of C5a-induced neutrophil chemotaxis and macrophage cytokine production in vitro by a new C5a receptor antagonist. Biochem Pharmacol. 2000;60(5):729–33. doi: 10.1016/S0006-2952(00)00361-0
- Liu Y, Xu SQ, Long WJ, et al. C5aR antagonist inhibits occurrence and progression of complement C5a induced inflammatory response of microglial cells through activating p38MAPK and ERK1/2 signaling pathway. Eur Rev Med Pharmacol Sci. 2018;22(22):7994–8003. doi: 10.26355/eurrev_201811_16428
- Fan J, Zhao L, Hu Q, et al. Screening for Virulence-Related Genes via a Transposon Mutant Library of Streptococcus suis Serotype 2 Using a Galleria mellonella Larvae Infection Model. Microorganisms. 2022;10(5):868. doi: 10.3390/microorganisms10050868
- Heath DS, Axelrad AA, McLeod DL, et al. Separation of the erythropoietin-responsive progenitors BFU-E and CFU-E in mouse bone marrow by unit gravity sedimentation. Blood. 1976;47(5):777–92. doi: 10.1182/blood.V47.5.777.777
- Yang R, Wang J, Wang F, et al. Blood–brain barrier integrity damage in bacterial meningitis: the underlying link, mechanisms, and therapeutic targets. Int J Mol Sci. 2023;24(3):2852. doi: 10.3390/ijms24032852