ABSTRACT
The arginine deiminase system (ADS) has been identified in various bacteria and functions to supplement energy production and enhance biological adaptability. The current understanding of the regulatory mechanism of ADS and its effect on bacterial pathogenesis is still limited. Here, we found that the XRE family transcriptional regulator XtrSs negatively affected Streptococcus suis virulence and significantly repressed ADS transcription when the bacteria were incubated in blood. Electrophoretic mobility shift (EMSA) and lacZ fusion assays further showed that XtrSs directly bind to the promoter of ArgR, an acknowledged positive regulator of bacterial ADS, to repress ArgR transcription. Moreover, we provided compelling evidence that S. suis could utilize arginine via ADS to adapt to acid stress, while ΔxtrSs enhanced this acid resistance by upregulating the ADS operon. Moreover, whole ADS-knockout S. suis increased arginine and antimicrobial NO in the infected macrophage cells, decreased intracellular survival, and even caused significant attenuation of bacterial virulence in a mouse infection model, while ΔxtrSs consistently presented the opposite results. Our experiments identified a novel ADS regulatory mechanism in S. suis, whereby XtrSs regulated ADS to modulate NO content in macrophages, promoting S. suis intracellular survival. Meanwhile, our findings provide a new perspective on how Streptococci evade the host’s innate immune system.
Introduction
Streptococcus suis (S. suis) is an emerging zoonotic pathogen that is responsible for various swine diseases, including septicaemia, meningitis, endocarditis, and arthritis, imposing a serious burden on the porcine industry [Citation1]. The first cases of human infection were reported in the late 1960s, followed by two epidemic outbreaks of human S. suis serotype 2 infections in China in 1998 and 2005, resulting in 229 infections and 52 deaths [Citation2,Citation3]. Since then, S. suis has become a serious public health concern. S. suis isolates were serologically classified into 29 confirmed serotypes (1–19, 21, 23–25, 27–31, and 1/2) according to capsular polysaccharides [Citation4]. Recently, 28 novel CPS loci (NCL1 to NCL-27 and Chz) S. suis strains isolated from diseased or healthy animals have been identified [Citation5]. Recently, our group reported a Chz serotype strain with high virulence, S. suis CZ130302, that can cause acute meningitis in piglets [Citation6].
S. suis pathogenicity is intimately connected with virulence factors such as extracellular factor (EF), lysozyme-releasing protein (MRP), haemolysin (SLY), and capsular polysaccharide (CPS), which cannot adequately represent the virulence of S. suis [Citation7,Citation8]. For instance, S. suis strain CZ130302 exhibited high virulence in piglets, despite lacking MRP, EF, and SLY [Citation9,Citation10]. Previous studies have shown that transcriptional factors, including SalK/SalR [Citation11], CiaRH [Citation12], and LuxS/AI-2 [Citation13], etc., could help S. suis adapt to different host environments and promote its pathogenicity by regulating the transcription of genes related to virulence and metabolism [Citation14]. Recently, we reported a novel XRE family transcriptional regulator, XtrSs, involved in bacterial adaptation to hydrogen peroxide stress in S. suis CZ130302 [Citation15]. Therefore, further research on the transcriptional regulation of S. suis during infection can provide a deeper explanation of its pathogenic mechanisms.
The arginine deiminase system (ADS) is responsible for the bacterial arginine deiminase (ADI) pathway, which converts arginine to citrulline, which provides energy for bacteria. The final product of the pathway, ammonia, binds to the environmental H+ to form NH4+, thus promoting internal pH balance and acid tolerance for bacteria [Citation16,Citation17]. ADS has been reported to contribute to bacterial biological fitness and virulence in Listeria monocytogenes [Citation18], Klebsiella pneumoniae [Citation19], Streptococcus pyogenes [Citation20], and Streptococcus pneumoniae [Citation21]. Benga et al. [Citation22] confirmed that ADS plays an essential role in enabling S. suis to survive in intracellular phagosomes for at least 24 h; however, the precise mechanism is unclear. Actually, the expression of ADS could be affected by a variety of environmental stresses, such as anaerobicity and temperature [Citation23,Citation24]. Additionally, ADS transcription is normally activated by the protein ArgR that directly interacts with the arcA promoter, as in Salmonella enterica [Citation25], Listeria monocytogenes [Citation26], and Pseudomonas aeruginosa [Citation27]. However, the regulation of ADS and its contribution to S. suis virulence are not fully understood.
In this study, we proved that S. suis ADS can be negatively regulated by the transcriptional regulator XtrSs via ArgR when mimicking bacterial infection in the blood. At the same time, we revealed a virulence regulation mechanism by which streptococcal transcriptional regulator XtrSs controls bacterial ADS against the host acidic environment and NO-mediated innate immune response.
Results
Depleted of XtrSs in S. suis CZ130302 presented increased virulence
The impact of regulator XtrSs on S. suis virulence was evaluated in a mouse model using a series of experiments. First, the median lethal dose (LD50) of the mutant strain ΔxtrSs was (1.13 ± 0.06) × 105 CFU/mouse, which was more than 10 times lower than that of the wild-type CZ130302 strain ((1.73 ± 0.14) × 106 CFU/mouse) (). We also investigated the survival curves of mice infected with the same doses of WT, ΔxtrSs and CΔxtrSs. All mice challenged with strain ΔxtrSs died within 4 days, and mortality in ΔxtrSs-infected mice was 100% (). The WT-infected mice died later than those challenged with ΔxtrSs, and the last WT-infected mice died on day 7 post-challenge (). The survival rate of CΔxtrSs-infected mice was 40% (). Next, we evaluated the survivability of the relevant strains incubated in mouse whole blood for 1.5 h. WT and CΔxtrSs showed lower survival rates when cultured in mice whole blood than ΔxtrSs (). We also recorded the blood bacterial load of mice every 12 h after the challenge with 2 × 106 CFU/mouse of various strains and found that ΔxtrSs-infected mice maintained a higher blood concentration than WT-infected and CΔxtrSs-infected groups (). These data clearly showed that XtrSs significantly attenuated the virulence of S. suis.
Figure 1. XtrSs contributed to the virulence of Streptococcus suis. (a) The Lethal Dose 50 test of ΔxtrSs and WT in a mouse infection model. Randomized groups of five mice were challenged with different strains at doses corresponding to 10-fold-diluted suspensions (104 to 108 CFU/mL). Mortality was monitored twice per day for 7 days after the challenge. (b) The survival curve of mice infected with ΔxtrSs, CΔxtrSs, and WT strains at the same doses (5 × 106 CFU/mouse). Each group contained 10 mice, and the survival rates were recorded every 24 h postinfection for 7 days. (c) Bacterial loads in the blood of S. suis-infection bacteriaemia mouse models. The blood was obtained from mouse orbital sinus at 0 h, 24 h, 36 h, and 48 h after i.p. challenge and plated on THB agar plates with serial dilution to calculate bacterial concentrations. (d) Whole blood killing assay. Cells of ΔxtrSs, CΔxtrSs, and WT strains at the mid-log phase were mixed with freshly prepared anticoagulated mouse blood, followed by incubation at 37°C for 90 min. The mixture was then plated on THA for viable bacterial enumeration. All data are represented as mean ± SD of triplicate samples (**: p < 0.01; ***: p < 0.001; ****: p < 0.0001; ns: no significance).
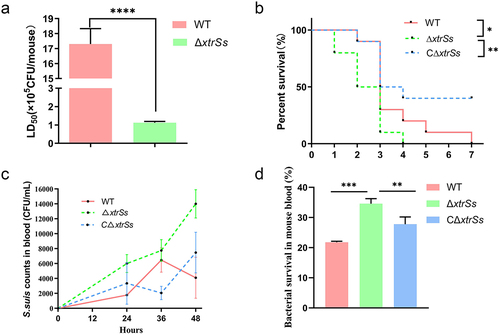
Screening of XtrSs regulated genes via transcriptomic analysis and qRT-PCR
To further determine the regulatory genes of XtrSs in vivo, the wild-type CZ130302 and ΔxtrSs strains were collected after incubating for 1.5 h in mouse whole blood and total RNA was extracted for high-throughput RNA sequencing (). Comparing the transcriptional levels of genes in ΔxtrSs with those in WT, we found 11 upregulated genes and 65 downregulated genes in ΔxtrSs (Table S3). COG annotated the functions of regulated genes, as shown in . The downregulated genes were mostly continuous and were predicted to encode prophage-related genes. As shown in , upregulated genes were identified in the gene cluster, mainly including the arginine deiminase system (ADS) for arginine metabolism, fucose operon (Fuc) for fucose metabolism, and fructose phosphotransferase system (Frut) for fructose utilization, among which ADS has been identified as a virulence-related factor in S. suis. Therefore, we aimed to investigate how XtrSs regulate ADS involvement in S. suis pathogenicity. In addition, qRT-PCR analysis showed that ADS-related genes in ΔxtrSs were significantly upregulated in the same culture environment (), which is consistent with the transcriptional results.
Figure 2. Transcriptome analysis of target genes regulated by XtrSs. (a) Schematic illustration of S. suis samples preparation for RNA-seq created with BioRender. (b) Distribution of the regulated genes into functional groups. (c) Analysis of gene cluster up-regulated in ΔxtrSs strain. Red: genes were significantly up-regulated in ΔxtrSs. (d) The transcription levels of ADS-related genes in the WT and ΔxtrSs strains. All data are represented as mean ± SEM of triplicate samples (**: p < 0.01; ***: p < 0.001; ****: p < 0.0001; ns: no significance).
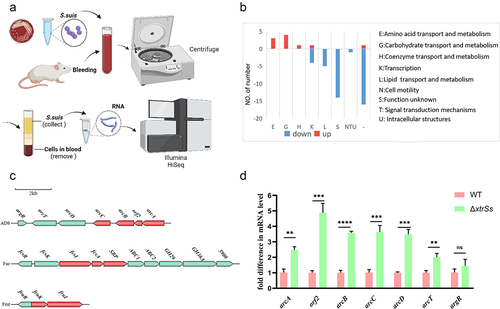
ADS analysis
The ADS gene clusters on Streptococcus genomes were aligned and contained the genes necessary for the ADI pathway, such as arcA, arcB, arcC, arcD, and arcT (). The transcriptional regulator ArgR was conserved in Streptococcus genomes near the ADS operon of S. suis, S. anginosus, S. agalactiae, S. dysgalactiae, and S. pyogenes, but far from the ADS operons of S. pneumoniae and S. oralis (). Moreover, we observed that ArgR and XtrSs homologues co-existence was commonly distributed in the Streptococcus species genome, with the highest proportions in S. anginosus (100%), S. dysgalactiae (100%), S. pneumoniae (98.54%), S. orails (81.25%), but less than 65% in S. sanguinis (62.50%), S. agalactiae (56.12%), S. suis (53.77%), and S. pyogenes (33.46%) ().
XtrSs up-regulated the ADS
As shown in , the ADS of CZ130302 was flanked by a gene argR, which has been identified as a regulator in a trimer or hexamer form [Citation28]. We found that the transcription levels of arcABC were significantly downregulated due to argR deletion (), suggesting that ArgR had a positive regulatory effect on S. suis ADS. Subsequently, we expressed rArgR and incubated it with the arcA promoter fragment for the EMSA assay, and confirmed that ArgR could bind to the arcA promoter (). The 3D structure of the ArgRtrimer or ArgRhexamer was constructed using AlphaFold 2 (Figure S1A) and used to predict the interaction with the DNA sequence of arcA promoter by molecular docking (Figure S1A). The results showed that the ArgRhexamer could bind to the sequence of “TATAATAGAAAGAATTATGCA,” which was contained in the reported ArgR-binding region [Citation29]. Moreover, XtrSs belong to the XRE family of transcriptional regulators containing a typical HTH domain, suggesting that XtrSs can bind to the promoter DNA of target genes. Considering that many transcriptional regulators are dimeric forms in nature, we constructed the 3D structures of the XtrSsdimer using AlphaFold 2, which showed that the linker could not disturb the structure of the XtrSsdimer (Figure S1B). To characterize the regulatory relationship of XtrSs on ADS, we expressed rXtrSsdimer and incubated it with the argR promoter and arcA promoter to perform EMSA. The results showed that the rXtrSsdimer can bind to the argR promoter sequence (), while it did not bind to the arcA promoter (Figure S2). To further determine the binding sequence of XtrSs on ADS, we performed two mutations in the argR promoter region and verified that the palindrome sequence “TAAACATGCTGTTGA” was the target sequence for XtrSs in the argR promoter by EMSA (). The molecular docking showed that XtrSsdimer could interact with the sequence “5‘-TAAACATGCTGTTGA-3’” in the argR promoter (Figure S1B). Consistently, we found that the argR promoter in ΔxtrSs showed higher activity than that in the WT by testing the β-galactose activity of argR-promoter-pTCV (). Additionally, by comparing the β-galactose activity of the arcA-promoter-pTCV plasmid in relevant S. suis strains, WT showed lower activity than ΔxtrSs and higher activity than ΔargR or ΔxtrSs: argR (). Notably, there was no significant difference in β-galactose activity between ΔargR and ΔxtrSs: argR (), implying that XtrSs have no effect on the transcriptional activity of the arcA promoter in the absence of ArgR. These data suggest that XtrSs affect the transcription of ADS by directly regulating the transcription of argR ().
Figure 4. XtrSs indirectly regulated ADS via ArgR. (a) The transcription levels of ADS-related genes in WT and ΔargR. (b) ArgR binds to the arcA promoter as identified by EMSA. (c) XtrSs bind to the argR promoter as identified by EMSA. (d) Schematic representation of argR promoter sequence mutations. (e) XtrSs was unable to bind to the argRMpromoter2 as identified by EMSA. The promoter activity of arcApromoter (f) and argRpromoter (g) in different S. suis strains identified by β-galactosidase assays. (h) Schematic diagram of ADS regulation by XtrSs and ArgR. DNA probes containing the 16S rRNA promoter region were used as negative controls in all EMSA assays. All data are represented as mean ± SEM of triplicate samples (*, p < 0.05; **, p < 0.01; ***, p < 0.001; ****, p < 0.0001; ns, not significant).
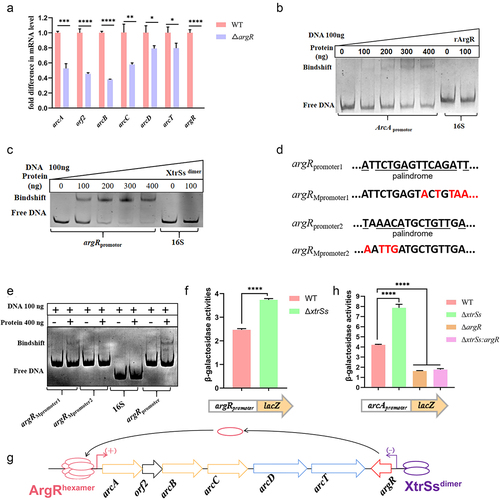
Arginine deiminase enzymatic activity
It is well known that the ADI coding by arcA is essential in the ADS pathway. We also demonstrated that the rADI of S. suis CZ130302 could decompose arginine into citrulline in a concentration-dependent manner (Figure S3A). To explore whether XtrSs affected ADI at the expression level in S. suis, whole bacterial proteins were extracted for the ADI substrate enzyme activity experiment. The citrulline produced by the WT was significantly higher than that produced by ΔADS (Figure S3B), indicating that S. suis CZ130302 could metabolize arginine to citrulline via its ADI. Citrulline production in ΔxtrSs was higher than that in WT and CΔxtrSs, although the difference was not significant (Figure S3B). These results indicate that the expression of ADI in S. suis was increased partly due to the lack of xtrSs.
The ADS played an important role in enhancing bacterial ability to resist acid via metabolizing arginine
The bacterial ADS can metabolize arginine to produce NH3 which can neutralize H+, thereby increasing the ability of pathogenic bacteria to resist acid [Citation26]. By monitoring the growth of various S. suis strains under different conditions (), we found that the WT was able to maintain a stabilization period with a higher OD600 in neutral THB (pH 7.4) with arginine supplementation than in the absence of arginine supplementation (). The WT strain growth curve at pH 6 THB exhibited a significant overall declined at 8–12 h when compared to that in neutral THB (). However, supplementation with 10 mM arginine in an acidic environment (pH 6 THB) helped the WT recover normal growth (). These findings were observed in ΔxtrSs and CΔxtrSs () but not in ΔADS when treated under the same conditions (). These results suggest that S. suis CZ130302 could metabolize arginine via ADS to recover growth in acidic THB. Moreover, qRT-PCR showed that ADS-related gene transcription was reduced after 1 h of incubation in a stressful acidic environment (), and ADS-related gene transcription levels were increased after supplementation with arginine (). These data confirmed that S. suis can deploy ADS to utilize arginine to defend against acid stress.
Figure 5. ADS helped S. suis to resist acidic environment. (a) Schematic diagram of measuring growth curves of S. suis under different conditions as marked created with BioRender.com. Growth curves of WT (b), ΔxtrSs (c), CΔxtrSs, (d) and ΔADS (e) under different conditions. The change transcription levels of ADS-related genes of WT when treated in THB (pH 7.4) or acidified THB (pH 5.5) (f) and in acidified THB (pH 5.5) with or without 10 mM arginine supplement (g). (h) Survival rates of different S. suis strains under acid stress of artificial gastric juice. All data are represented as mean ± SEM of triplicate samples (*, p < 0.05; **, p < 0.01; ***, p < 0.001; ****, p < 0.0001; ns, no significance).
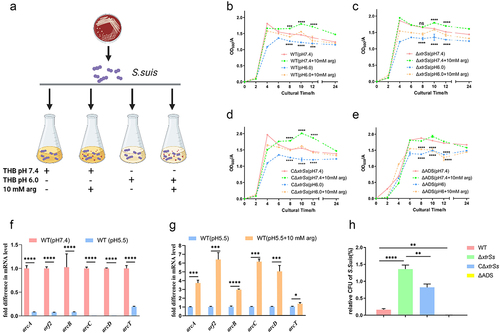
To investigate the effect of XtrSs on acid stress, we configured artificial gastric juice (pH 4.5) to simulate the gastric environment in a naive host for S. suis, such as piglet [Citation30]. After incubating for 1 h in artificial gastric juice, the survival rate of ΔxtrSs was significantly higher than that of wild-type CZ130302, while the complementary strain was restored to a certain extent (). The survival rate of ΔADS was less than 0.1% (). Based on the above results, we speculated that ΔxtrSs enhanced the ability to resist acid by upregulating ADS in artificial gastric juice.
Biological adaptation of ΔxtrSs in host cells was enhanced by promoting ADS
To further mimic in vivo infection, WT and ΔxtrSs were incubated with RAW 264.7, at 1, 2, 3, and 4 h. The intracellular viability of the ΔxtrSs strain was greatly increased compared to that of the WT at 3 and 4 h, whereas ΔADS had decreased intracellular viability at 2–4 h (). Moreover, after incubation for 3 h, the intracellular arginine content in ΔxtrSs-infected cells decreased, while that in ΔADS-infected cells increased compared to that in WT-infected cells, suggesting that the ADI pathway can consume arginine intracellularly (). Given that arginine in macrophages is also an important source of NO, we examined the cellular concentration of NO. The results showed that NO production was much higher in WT-infected cells than in ΔxtrSs-infected cells, whereas NO production was significantly increased in cells infected with ΔADS (). However, no visible change was observed in the iNOS expression of cells after being treated with various S. suis strains (Figure S4). Meanwhile, our results showed no expression of Arginase 1 (Arg 1) in RAW264.7 infected by S. suis (Figure S4). During the pathogenic bacteria invasion, macrophages should switch to the M1 type which did not express Arg 1 but express iNOS [Citation31,Citation32]. So our results also confirmed the situation of RAW264.7 in the phagocytosis experiment is M1 type. Cells without any treatment were used as a control (Mock group) and showed no expression of iNOS and Arginase-1 (Figure S4), which indicated that the cells were unactivated. These findings suggested that the increase in intracellular adaptation of ΔxtrSs was because the ADI competes with inducible nitric oxide synthase (iNOS) in host cells to catabolize arginine, thus causing cellular arginine deprivation and antimicrobial substance NO decrease (). In addition, we examined the effect of ADS on bacterial virulence. As shown in , the survival rate of the ADS mutant strain (100%) was higher than that of the WT (70%), indicating that the expression of ADS was positively correlated with the virulence of the WT, which partly explains the enhanced virulence of ΔxtrSs.
Figure 6. ADS facilitated S. suis to evade killing from macrophages. (a) Intracellular survival ability of the WT, ΔxtrSs and ΔADS strains in RAW264.7 macrophage cells. (b) Intracellular arginine incubated with different S. suis strains. (c) NO production of RAW264.7 incubated with different S. suis strains. (d) The mechanism by which S. suis ADS resists the killing effect of RAW264.7 macrophage cells created with BioRender. The increased survival of S. suis may be mediated by decreased NO and H+ due to competition for cellular arginine by ADS. (e) Survival percentage of mice infected with the WT and ΔADS strains. Each group containing 10 mice was monitored every 24 h. All data are represented as mean ± SEM of triplicate samples (*: p < 0.05; **: p < 0.01; ***: p < 0.001; ****: p < 0.0001; ns: no significance).
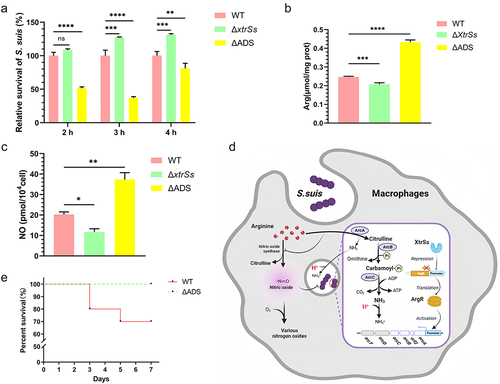
Discussion
As a zoonotic pathogen, research on S. suis pathogenic mechanism of S. suis during the infection process is necessary and helpful in solving public health problems [Citation33]. S. suis contains multiple virulence factors that are associated with complex regulatory networks [Citation8]. Currently, the known transcriptional regulators of S. suis are most likely to positively regulate virulence genes with the exception of CovR [Citation34]. Herein, we showed that XtrSs, as a negative regulator, weakened the pathogenicity of S. suis by inhibiting ADS transcription. At the same time, ADS modulated by XtrSs can utilize arginine to help S. suis adapt to acid stress in vitro and escape macrophage bactericidal effects in vivo. Our findings are important for understanding the interaction between S. suis and the host innate immune system.
As an ADS-specific transcriptional regulator, ArgR directly binds to the promoter of the ADS operon, thereby promoting the transcription of ADS genes [Citation23,Citation35]. In this study, we demonstrated that XtrSs did not directly regulate the ADS system but directly regulated ArgR and suppressed ADS transcription and expression. In addition, S. suis ADS has been reported to be regulated by carbon catabolite protein A (CcpA) [Citation23,Citation36], the two-component gene regulatory system (CovRS) [Citation34], the regulatory genes of the glucosyltransferase (Rgg) [Citation37] and FNR-like protein of S. suis (FlpS) [Citation38], all of which were obtained by RNA-seq analysis in laboratory culture. In contrast, the regulation of ADS by XtrSs in our study was discovered by RNA-seq during blood incubation, similar to the environment in vivo. Many studies have reported that genes in bacteria are differentially regulated during in vivo colonization compared with laboratory nurturing [Citation39,Citation40]. Indeed, we found that the transcription of ADS-related genes was not affected by XtrSs under THB conditions (Figure S5). Meanwhile, ADI expression was not remarkably altered by XtrSs (Figure S3B). Consistently, our study results on ADS transcriptional regulation more rigorously reflect the condition in S. suis deploys gene transcription during S. suis infection. To adapt to variable host environments, S. suis ADS transcription is likely regulated by a complex regulatory network, which requires further investigation.
ADS is relevant for the resistance to acidic conditions [Citation41,Citation42]. Growth curves and qRT-PCR analyses in stressful environments demonstrated that S. suis ADS could use arginine to alleviate acid stress and maintain growth. Since gastrointestinal infection is also an important route of S. suis infection, resistance to gastric juice acidity is crucial for S. suis colonization and reproduction. The physiological pH of the gastric juice of piglets from days 17 to 35 ranged from 5.83 to 2.60 [Citation43]. Notably, arginase expression was absent in the enterocytes of suckling piglets, which is a metabolic strategy that ensures the maximum supply of arginine in breast milk to support the rapid growth of newborn piglets [Citation44]. In this case, abundant arginine and mild acid stress in the intestinal environment of unweaned piglets create favourable conditions for pathogenic bacteria that possess ADS [Citation43]. This may be one of the reasons why newborn piglets are more susceptible to S. suis infection than weaned pigs.
Additionally, bacteria need to resist extreme environments, such as acidic environments and some reactive oxygen species, in phagocytes during infection. In this study, we found that ADS-knockout S. suis resulted in an increase in intracellular arginine and NO, which led to a decrease in bacterial viability in macrophages, ultimately affecting bacterial virulence in mice. Similarly, studies have shown that L-arginine-dependent reactive nitrogen production is the primary mechanism for killing and inhibiting Tuberculosis in mouse macrophages [Citation45,Citation46]. Moreover, the virulence of ΔarcA or ΔarcB in S. pyogenes was attenuated, but the virulence of ΔarcA recovered in iNOS−/− mice, demonstrating that the availability of ADS had no critical effect on S. pyogenes virulence when lacking NO-mediated innate immunity [Citation47,Citation48]. Together, these results provide strong evidence that bacterial ADS can compete with cellular iNOS for arginine to antagonize NO production to defend against innate immunity.
In conclusion, our work revealed a novel transcriptional regulation mechanism of S. suis ADS and confirmed that ADS promotes the virulence of S. suis by modulating intracellular NO and arginine under the regulation of XtrSs. Our results provide insights into the regulation of streptococcal arginine metabolism in response to host innate immunity.
Materials and methods
Ethics statement
All animal studies were approved by the Experimental Animal Welfare and Ethics Committee of Nanjing Agricultural University (Approval ID: SYXK(SU)2021-0086) and were performed in accordance with the Animal Welfare Agency Guidelines.
Bacterial strains, plasmids, and culture conditions
The S. suis virulent strain CZ130302 was isolated and preserved in our laboratory from piglets suffering from acute meningitis [Citation6]. The S. suis strains were grown in THB (Hopebio, Qingdao, China) liquid medium or THB agar plates (THA) supplemented with 5% sheep blood (Maojie, Nanjing, China) at 37°C incubator or shaker (180 rpm); a final concentration of 100 μg/mL spectinomycin (Spc) was added as needed. E. coli was cultured in a Luria-Bertani Broth (LB) liquid medium or LB solid medium in a 37°C incubator or shaker (180 rpm); 50 μg/mL Spc or kanamycin (Kan) 50 μg/mL was added as needed. All chemicals and enzymes used for molecular cloning were purchased from Sigma (Beijing, China) and New England Biolabs (Beijing, China) unless otherwise stated. The bacterial strains and plasmids used in the present study are listed in Table S1.
Mutant construction
A series of mutant strains was created via natural DNA transformation. The signal peptide ComS13–21 (GNWGKWTDG) was employed to trigger the natural transformation of Streptococcus suis CZ130302 [Citation49]. The upstream and downstream sequences of the target gene, as well as the sacB-spc cassette, were initially amplified independently and then joined to create a “three fragments fusion” by fusion PCR which was introduced by natural transformation into the target strain and then create primary mutant strain containing antibiotic marker by homologous arm exchange. The fused homologous fragment, without sacB-spc cassette, was then transferred to the primary marked mutant for a second homologous arm exchange. Spectinomycin gene was used for positive selection, whereas sacB gene, which confers sucrose sensitivity, was used for negative selection. Primers used in this study are listed in Table S2.
Bacterial survival in mouse blood
To investigate the susceptibility of S. suis to whole blood from mice, a blood survival test was performed according to a previous method [Citation50]. Briefly, S. suis in the mid-log phase (OD600 = 0.6–0.8) was diluted into fresh mouse whole blood to maintain a concentration of 2 × 108 CFU/mL. After incubation at 37°C for 1.5 h with mixing gently every 15 min, the CFU was determined by plating on THA. The percentage of survival of different strains in mouse whole blood was calculated using the following formula: (CFU of sample before incubation/CFU of sample after incubation) × 100%.
Mouse infection assays
Streptococcus suis CZ130302 and the mutant strains were grown to logarithmic phase (OD600 = 0.6 ~ 0.8), harvested, washed thrice in PBS, diluted in the same buffer to approximately 2.5 × 107 CFU/mL counted on THA, and kept on ice until injection. Ten mice were divided into two groups. The bacterial suspension (200 μL) was inoculated intraperitoneally (i.p.). PBS was used as a control. After the injection of bacteria, the survival rate was monitored every 12 h for seven days.
Median lethal dose (LD50) assay
Groups of mice (n = 5/group) with a defined weight range (20–25 g) were used. They were intraperitoneally injected with 0.2 mL of different doses of S. suis (5 × 104–5 × 108 CFU/mouse). Control mice were injected with sterilized PBS. The LD50 values were determined by recording the deaths 7 days after the injection. The original data from the three replicate experiments were shown in Table S4, and the LD50 values were calculated using the Reed – Muench method [Citation51].
Dynamic determination of bacterial blood load
Dynamic determination of the bacterial blood load was performed as the protocol described previously [Citation52]. Briefly, three mice in each group were challenged with the indicated S. suis strains at a dose of approximately 1 × LD50 (1 × 106 CFU/mouse) by intraperitoneal injection. The negative control group was challenged with an equal volume of sterile PBS. At 24 h post-infection, blood was collected from the mouse orbital sinus every 12 h using capillary tubes sterilized by ultraviolet light in advance, and the CFUs were counted on THA.
RNA extraction and RNA-seq
To mimic the in vivo environment of S. suis infecting the host, the bacteria were gently mixed with fresh mouse whole blood containing 0.4% sodium citrate at a ratio of 1:10, resulting in a final concentration of 2 × 107 CFU/mL. The samples were incubated for 1.5 h at 37°C with gentle mixing every 15 min. Subsequently, the cellular components in the blood were separated by differential centrifugation, and the bacterial precipitates were collected for RNA extraction using the TRIzol kit (Vazyme, China). RNA samples were sent to the company for transcriptome sequencing using an Illumina HiSeqTM 2000 high-throughput sequencing platform. Differentially transcribed genes were screened if the fold change was >1 and the P-value was less than 0.05.
Quantitative real-time PCR assay
RNA was extracted using TRIzol reagent (Vazyme, China), followed by reverse transcription using HiScript Q RT SuperMix (Vazyme, China). The cDNA was then used for qRT-PCR with SYBR qPCR Green Master Mix (Vazyme, China). GAPDH was used as an internal control. The primers used for qRT-PCR are listed in Table S2.
Bioinformatics analysis
In general, the ADS gene clusters exhibit highly similar genomic sequences within the same species. Sequences of ADS gene clusters in Streptococcus species genomes were determined using BLAST. The corresponding sequence and gene annotation information were obtained from the NCBI GenBank. Homology analysis of ADS gene clusters was performed using the Easyfig software. Using the Streptococcus genomes published in NCBI as a database, the distribution of ArgR and XtrSs in Streptococcus species was identified by tBLASTn. The 3D structure of the protein was predicted using AlphaFold2 database [Citation53]. Molecular docking of proteins and DNA was predicted using HDOCK (http://hdock.phys.hust.edu.cn/) [Citation54].
Determination of the galactosidase activity
Galactosidase activity assay was performed according to a method reported previously [Citation55]. Briefly, the constructed promoter and pTCV fusion vectors were transformed into the test strains. Then, 400 μL of log-phase strain culture, 8 μL of lysozyme (2.5 mg/mL), and 25 μL of 4×Z-buffer (14 μL β-mercaptoethanol) were mixed and incubated at 37°C for 25 min. Each sample was then added to 400 μL of 1×Z-buffer (2.7 μL of β-mercaptoethanol and 2 mg of o-nitrophenyl β-D-galactopyranoside ONPG)) and continued at 37°C water bath until fully yellowed. The reaction was terminated by the addition of 400 μL 1 M Na2CO3. The samples were centrifuged, and the supernatant was collected to measure the OD420. β-galactosidase activity was calculated using the following formula: [MU]={OD420 × 1000 × Vε[μL]}/{Vs[μL] × RT[min] × OD600}. MU is the Miller-Units, VE is the total volume of 1235 μL, and Vs is the sample volume of 400 μL.
Recombinant protein expression
Engineered bacteria BL21 containing XtrSsdimer-pET28a(+) were stored in our laboratory. Details of protein expression and purification methods referred to previous descriptions [Citation15]. After LB agar with kanamycin resuscitated the BL21, individual bacterial clones were picked, and the bacterial culture was added to 1 mM IPTG in log phase and incubated at 16°C for 16 h. Subsequently, we purified soluble his-tagged XtrSsdimer using nickel columns (GE Healthcare).
Electrophoretic mobility shift assay (EMSA)
The electrophoretic mobility shift assay was performed as previously reported [Citation39]. The tested promoter region (250 bp) was amplified and the fragment was recovered using a gel purification kit. Isometric 16S rRNA sequence was used as the negative control. The DNA fragments of the mutant argR promoter were synthesised by Sangon (Shanghai, China). The DNA fragments were incubated with the above proteins for gel retardation assay (EMSA). Protein concentrations were increased from 0 to 400 ng, and DNA at 100 ng in the binding buffer (10 mM Tris base, 50 mM KCl, 5 mM MgCl2, 1 mM dithiothreitol, 0.05% Nonidet p-40, 2.5% glycerol, pH7.5) as a reaction environment. After co-incubation at 37°C for 30 min, native 6% polyacrylamide gel electrophoresis (native-PAGE) was performed at low temperature and stained in 0.5×TBE containing ethidium bromide nucleic acid dye for 20 min.
Determination of arginine deiminase (ADI) activity
ADI activity was determined by measuring the production of L-citrulline from L-arginine and evaluated according to a protocol described previously [Citation18]. In total, 50 mL cultures of the tested strains in the plateau phase were centrifuged, washed twice with PBS, and resuspended in 1 mL lysis buffer (2% TritonX-100, 100 mM NaCl, 10 mM Tris-HCl, pH 8.0, 1 mM EDTA). After breaking the bacteria using an MP instrument, the bacterial proteins were collected from the supernatant by centrifugation. The 250 μL of bacterial protein was mixed with 100 μL of substrate (80 mM arginine, 100 mM potassium phosphate buffer, pH 6.2) reacting for 1 h at 37°C. Then, 120 μL of the reaction solution was added to 400 μL of chromogenic solution, treated at 95°C for 15 min, and cooled at room temperature for 10 min. OD550 was measured to determine the production of citrulline according to the citrulline standard curve. Citrulline standards and uninoculated reagents were used as positive and blank controls, respectively. The results are expressed as nanomoles of citrulline produced per hour per mg of whole cell protein.
Monitoring of bacterial growth
S. suis strains from overnight cultures were centrifuged and washed twice with sterilized PBS before the OD600 was adjusted to approximately 0.6, and then diluted 1:50 into the desired medium, such as neutral (pH = 7.4) or acidic stress (pH = 6.0) in THB liquid medium, with or without 10 mM supplemental arginine. The OD600 was measured every 2 h from 0 to 12 h, and again at 24 h, with shaking for 10 s before each measurement.
Acid stress challenges
To evaluate the role of XtrSs in acid tolerance, WT and mutant strains were challenged with artificial gastric juice according to previously described protocols [Citation18]. The log-phase bacteria cultured in THB were harvested, washed in PBS, and resuspended in artificial gastric juice (8.3 g proteose peptone, 3.5 g d-glucose, 2.05 g NaCl, 0.6 g KH2PO4, 0.11 g CaCl2, 0.37 g KCl, 0.05 g bile, 0.1 g lysozyme, and 13.3 mg pepsin), with pH 4.5 which were achieved by adjustment with HCl. Changes in the cell population were determined by plate counting after 1 h of incubation. The test was performed in triplicate and repeated three times.
Intracellular survival assays
Intracellular survival assays were performed as described previously [Citation56]. Briefly, the bacterial strains in the mid-exponential growth phase (OD600 = 0.6) were incubated with macrophages RAW264.7 for 1.5 h at a multiplicity of infection (MOI) of 100 in DMEM without antibiotics. Adhesion to macrophages did not differ between the WT and mutant strains (data not shown). The infected cells were washed three times with PBS and incubated in DMEM containing gentamicin (100 g/mL) and penicillin (5 g/mL) to eliminate extracellular bacteria that had not been internalized by RAW264.7. To measure the survival of intracellular bacteria, infected cells were sampled 1, 2, 3, and 4 h after the addition of antibiotics. Serial dilutions of cell lysates were plated onto THA. Each assay was independently repeated thrice.
Determination of the arginine and nitric-oxide production
The different strains were added into the RAW264.7 cell holes at an MOI of 100 incubating for 1.5 h. The hole without strain was used as the control. The culture medium in these wells was replaced with DMEM containing gentamicin (100 g/mL) and penicillin (5 g/mL). The arginine content produced by RAW264.7 was determined using an arginine assay kit (Solarbio, China) after incubating for 3 h. The NO content produced by RAW264.7 cells was determined using a micro NO content assay kit (Solarbio, China) after 24 h.
Western blot
Western blot was performed to test the expression of iNOS and Arg 1 in RAW264.7 cells after infection with different strains. As mentioned above, the treatments applied to RAW264.7 cells were performed as described previously [Citation56], the infected cells were selected at 3 h after antibiotic addition. RAW264.7 cells without any treatment were used as a control group. Three biological replicates were performed for each group. Lysis was performed in a cellular lysate buffering solution (1× RIPA, CST, America) for 0.5 h, followed by centrifugation at 12,000 g at 4°C for 15 min to harvest the supernate. The cell proteins were separated on SDS-PAGE gel and moved onto a PVDF film (Millipore, America). After being blocked in 5% skimmed milk containing 0.1% Tween 20 for 120 min at room temperature, the membranes were incubated overnight at 4°C with primary antibodies. Rabbit-sourced primary antibodies of iNOS, Arg 1, and β-actin (1:1000) were obtained from Abmart (Shanghai, China). β-Actin was utilized as internal control. After incubation with an anti-rabbit secondary antibody bound to horseradish peroxidase (1:5000, Santa Cruz Biotechnology, America), the protein was visualized by an enhanced chemiluminescence reagent (Thermo Pierce, USA).
Statistical analyses
Statistical analyses were performed using GraphPad Prism (version 8.0) (GraphPad Software, La Jolla, CA, USA). One-way analysis of variance (ANOVA) was performed to analyse the data from the experiments on bacterial survival in mouse blood, acid stress challenges, intracellular survival assays, and determination of arginine and nitric-oxide production. Growth curve analyses were performed using a two-way ANOVA. Two-tailed unpaired Student’s t-test was used for other assays. Survival curves were analysed using the log-rank (Mantel-Cox) test. Statistical significance was set at a p value of 0.05.
240105 Supplementary material.docx
Download MS Word (949.3 KB)Acknowledgements
We thank Shaynoor Dramsi for providing the plasmid pTCV-lac.
Disclosure statement
No potential conflict of interest was reported by the author(s).
Data Availability statement
The datasets are available from the corresponding author on reasonable request.
Supplemental data
Supplemental data for this article can be accessed online at https://doi.org/10.1080/21505594.2024.2306719
Additional information
Funding
References
- Goyette-Desjardins G, Auger JP, Xu J, et al. Streptococcus suis, an important pig pathogen and emerging zoonotic agent-an update on the worldwide distribution based on serotyping and sequence typing. Emerg Microbes Infect. 2014;3:e45. doi: 10.1038/emi.2014.45
- Tang J, Wang C, Feng Y, et al. Streptococcal toxic shock syndrome caused by Streptococcus suis serotype 2. PLOS Med. 2006;3(5):e151. doi: 10.1371/journal.pmed.0030151
- Gao T, Yuan F, Liu Z, et al. MnmE, a central tRNA-modifying GTPase, is essential for the growth, pathogenicity, and arginine metabolism of Streptococcus suis serotype 2. Front Cell Infect Microbiol. 2019;9:173. doi: 10.3389/fcimb.2019.00173
- Wileman TM, Weinert LA, Howell KJ, et al. Pathotyping the zoonotic pathogen Streptococcus suis: novel genetic markers to differentiate invasive disease-associated isolates from non-disease-associated isolates from England and Wales. J Clin Microbiol. 2019;57(7). doi: 10.1128/JCM.01712-18
- Liang P, Wang M, Gottschalk M, et al. Genomic and pathogenic investigations of Streptococcus suis serotype 7 population derived from a human patient and pigs. Emerg Microbes Infect. 2021;10(1):1960–15. doi: 10.1080/22221751.2021.1988725
- Pan Z, Ma J, Dong W, et al. Novel variant serotype of Streptococcus suis isolated from piglets with meningitis. Appl Environ Microbiol. 2015;81(3):976–985. doi: 10.1128/AEM.02962-14
- Segura M, Fittipaldi N, Calzas C, et al. Critical Streptococcus suis virulence factors: are they all really critical? Trends Microbiol. 2017;25(7):585–599. doi: 10.1016/j.tim.2017.02.005
- Fittipaldi N, Segura M, Grenier D, et al. Virulence factors involved in the pathogenesis of the infection caused by the swine pathogen and zoonotic agent Streptococcus suis. Future Microbiol. 2012;7:259–279. doi: 10.2217/fmb.11.149
- Vecht U, Wisselink HJ, Jellema ML, et al. Identification of two proteins associated with virulence of Streptococcus suis type 2. Infect Immun. 1991;59(9):3156–3162. doi: 10.1128/iai.59.9.3156-3162.1991
- Tenenbaum T, Asmat TM, Seitz M, et al. Biological activities of suilysin: role in Streptococcus suis pathogenesis. Future Microbiol. 2016;11:941–954. doi: 10.2217/fmb-2016-0028
- Shen X, Zhong Q, Zhao Y, et al. Proteome analysis of the two-component SalK/SalR system in epidemic Streptococcus suis serotype 2. Curr Microbiol. 2013;67(1):118–122. doi: 10.1007/s00284-013-0343-4
- Li J, Tan C, Zhou Y, et al. The two-component regulatory system CiaRH contributes to the virulence of Streptococcus suis 2. Vet Microbiol. 2011;148(1):99–104. doi: 10.1016/j.vetmic.2010.08.005
- Wang Y, Wang Y, Sun L, et al. The LuxS/AI-2 system of Streptococcus suis. Appl Microbiol Biotechnol. 2018;102(17):7231–7238. doi: 10.1007/s00253-018-9170-7
- Zheng C, Li L, Ge H, et al. Role of two-component regulatory systems in the virulence of Streptococcus suis. Microbiol Res. 2018;214:123–128. doi: 10.1016/j.micres.2018.07.002
- Zhang Y, Liang S, Pan Z, et al. XRE family transcriptional regulator XtrSs modulates Streptococcus suis fitness under hydrogen peroxide stress. Arch Microbiol. 2022;204(5):244. doi: 10.1007/s00203-022-02854-5
- Noens EE, Kaczmarek MB, Zygo M, et al. ArcD1 and ArcD2 arginine/Ornithine exchangers encoded in the arginine deiminase pathway gene cluster of Lactococcus lactis. J Bacteriol. 2015;197(22):3545–3553. doi: 10.1128/JB.00526-15
- Cotter PD, Hill C. Surviving the acid test: responses of gram-positive bacteria to low pH. Microbiol Mol Biol Rev. 2003;67(3):429–453. table of contents. doi: 10.1128/MMBR.67.3.429-453.2003
- Chen J, Cheng C, Xia Y, et al. Lmo0036, an ornithine and putrescine carbamoyltransferase in Listeria monocytogenes, participates in arginine deiminase and agmatine deiminase pathways and mediates acid tolerance. Microbiology (Reading). 2011;157(11):3150–3161. doi: 10.1099/mic.0.049619-0
- Ahn D, Penaloza H, Wang Z, et al. Acquired resistance to innate immune clearance promotes Klebsiella pneumoniae ST258 pulmonary infection. JCI Insight. 2016;1(17):e89704. doi: 10.1172/jci.insight.89704
- Freiberg JA, Le Breton Y, Harro JM, et al. The arginine deiminase pathway impacts antibiotic tolerance during Biofilm-Mediated Streptococcus pyogenes infections. MBio. 2020;11(4):11. doi: 10.1128/mBio.00919-20
- Schulz C, Gierok P, Petruschka L, et al. Regulation of the arginine deiminase system by ArgR2 interferes with arginine metabolism and fitness of Streptococcus pneumoniae. MBio. 2014;5. doi: 10.1128/mBio.01858-14
- Benga L, Goethe R, Rohde M, et al. Non-encapsulated strains reveal novel insights in invasion and survival of Streptococcus suis in epithelial cells. Cell Microbiol. 2004;6(9):867–881. doi: 10.1111/j.1462-5822.2004.00409.x
- Gruening P, Fulde M, Valentin-Weigand P, et al. Structure, regulation, and putative function of the arginine deiminase system of Streptococcus suis. J Bacteriol. 2006;188(2):361–369. doi: 10.1128/JB.188.2.361-369.2006
- Winterhoff N, Goethe R, Gruening P, et al. Identification and characterization of two temperature-induced surface-associated proteins of Streptococcus suis with high homologies to members of the arginine deiminase system of Streptococcus pyogenes. J Bacteriol. 2002;184(24):6768–6776. doi: 10.1128/JB.184.24.6768-6776.2002
- Choi Y, Choi J, Groisman EA, et al. Expression of STM4467-encoded arginine deiminase controlled by the STM4463 regulator contributes to Salmonella enterica serovar Typhimurium virulence. Infect Immun. 2012;80(12):4291–4297. doi: 10.1128/IAI.00880-12
- Ryan S, Begley M, Gahan CG, et al. Molecular characterization of the arginine deiminase system in Listeria monocytogenes: regulation and role in acid tolerance. Environ Microbiol. 2009;11:432–445. doi: 10.1111/j.1462-2920.2008.01782.x
- Lu CD, Winteler H, Abdelal A, et al. The ArgR regulatory protein, a helper to the anaerobic regulator ANR during transcriptional activation of the arcD promoter in Pseudomonas aeruginosa. J Bacteriol. 1999;181(8):2459–2464. doi: 10.1128/JB.181.8.2459-2464.1999
- Van Duyne GD, Ghosh G, Maas WK, et al. Structure of the oligomerization and L-arginine binding domain of the arginine repressor of Escherichia coli. J Mol Biol. 1996;256:377–391. doi: 10.1006/jmbi.1996.0093
- Fulde M, Willenborg J, de Greeff A, et al. ArgR is an essential local transcriptional regulator of the arcABC operon in Streptococcus suis and is crucial for biological fitness in an acidic environment. Microbiol-Sgm. 2011;157:572–582. doi: 10.1099/mic.0.043067-0
- Ferrando ML, Schultsz C. A hypothetical model of host-pathogen interaction of Streptococcus suis in the gastro-intestinal tract. Gut Microbes. 2016;7(2):154–162. doi: 10.1080/19490976.2016.1144008
- Brodsky IE. JAK-ing into M1/M2 polarization SteErs salmonella-containing macrophages away from immune attack to promote bacterial persistence. Cell Host Microbe. 2020;27(1):3–5. doi: 10.1016/j.chom.2019.12.007
- Misra S, Lee TJ, Sebastian A, et al. Loss of selenoprotein W in murine macrophages alters the hierarchy of selenoprotein expression, redox tone, and mitochondrial functions during inflammation. Redox Biol. 2023;59:59. doi: 10.1016/j.redox.2022.102571
- Segura M, Calzas C, Grenier D, et al. Initial steps of the pathogenesis of the infection caused by Streptococcus suis: fighting against nonspecific defenses. FEBS Lett. 2016;590(21):3772–3799. doi: 10.1002/1873-3468.12364
- Pan X, Ge J, Li M, et al. The orphan response regulator CovR: a globally negative modulator of virulence in Streptococcus suis serotype 2. J Bacteriol. 2009;191(8):2601–2612. doi: 10.1128/JB.01309-08
- Fulde M, Willenborg J, de Greeff A, et al. ArgR is an essential local transcriptional regulator of the arcABC operon in Streptococcus suis and is crucial for biological fitness in an acidic environment. Microbiology (Reading). 2011;157(2):572–582. doi: 10.1099/mic.0.043067-0
- Willenborg J, de Greeff A, Jarek M, et al. The CcpA regulon of Streptococcus suis reveals novel insights into the regulation of the streptococcal central carbon metabolism by binding of CcpA to two distinct binding motifs. Mol Microbiol. 2014;92(1):61–83. doi: 10.1111/mmi.12537
- Zheng F, Ji H, Cao M, et al. Contribution of the Rgg transcription regulator to metabolism and virulence of Streptococcus suis serotype 2. Infect Immun. 2011;79(3):1319–1328. doi: 10.1128/IAI.00193-10
- Willenborg J, Koczula A, Fulde M, et al. FlpS, the FNR-Like protein of Streptococcus suis is an essential, oxygen-sensing activator of the arginine deiminase system. Pathogens. 2016;5(3):51. doi: 10.3390/pathogens5030051
- Liu G, Gao T, Zhong X, et al. The novel streptococcal transcriptional regulator xtgs negatively regulates bacterial virulence and directly represses psep transcription. Infect Immun. 2020;88(10):88. doi: 10.1128/IAI.00035-20
- Cook LCC, Hu H, Maienschein-Cline M, et al. A vaginal tract signal detected by the group B Streptococcus SaeRS system elicits transcriptomic changes and enhances murine colonization. Infect Immun. 2018;86(4). doi: 10.1128/IAI.00762-17
- Xiong L, Teng JL, Watt RM, et al. Arginine deiminase pathway is far more important than urease for acid resistance and intracellular survival in laribacter hongkongensis: a possible result of arc gene cassette duplication. BMC Microbiol. 2014;14(1):42. doi: 10.1186/1471-2180-14-42
- Tian J, Utter DR, Cen L, et al. Acquisition of the arginine deiminase system benefits epiparasitic Saccharibacteria and their host bacteria in a mammalian niche environment. Proc Natl Acad Sci U S A. 2022;119(2):119. doi: 10.1073/pnas.2114909119
- Warneboldt F, Sander SJ, Beineke A, et al. Clearance of Streptococcus suis in stomach contents of differently fed growing pigs. Pathogens. 2016;5(3):56. doi: 10.3390/pathogens5030056
- Zou S, Wang X, Liu P, et al. Arginine metabolism and deprivation in cancer therapy. Biomed Pharmacother. 2019;118:109210. doi: 10.1016/j.biopha.2019.109210
- Chan J, Xing Y, Magliozzo RS, et al. Killing of virulent mycobacterium tuberculosis by reactive nitrogen intermediates produced by activated murine macrophages. J Exp Med. 1992;175(4):1111–1122. doi: 10.1084/jem.175.4.1111
- McKell MC, Crowther RR, Schmidt SM, et al. Promotion of anti-tuberculosis macrophage activity by L-Arginine in the absence of nitric oxide. Front Immunol. 2021;12:653571. doi: 10.3389/fimmu.2021.653571
- Cusumano ZT, Watson ME Jr., Caparon MG, et al. Streptococcus pyogenes arginine and citrulline catabolism promotes infection and modulates innate immunity. Infect Immun. 2014;82(1):233–242. doi: 10.1128/IAI.00916-13
- Starikova EA, Golovin AS, Vasilyev KA, et al. Role of arginine deiminase in thymic atrophy during experimental Streptococcus pyogenes infection. Scand J Immunol. 2019;89(2):e12734. doi: 10.1111/sji.12734
- Zhang Y, Lu P, Pan Z, et al. SssP1, a Streptococcus suis Fimbria-Like Protein Transported by the SecY2/A2 System, contributes to bacterial virulence. Appl Environ Microbiol. 2018;84(18):84. doi: 10.1128/AEM.01385-18
- Echenique-Rivera H, Muzzi A, Del Tordello E, et al. Transcriptome analysis of Neisseria meningitidis in human whole blood and mutagenesis studies identify virulence factors involved in blood survival. PLoS Pathog. 2011;7(5):e1002027. doi: 10.1371/journal.ppat.1002027
- Stanic M. A simplification of the estimation of the 50 percent endpoints according to the Reed and Muench method. Pathol Microbiol (Basel). 1963;26:298–302. doi: 10.1159/000161378
- Liu G, Zhang S, Gao T, et al. Identification of a novel broad-spectrum endolysin, Ply0643, with high antibacterial activity in mouse models of streptococcal bacteriaemia and mastitis. Res Vet Sci. 2022;143:41–49. doi: 10.1016/j.rvsc.2021.12.014
- Jumper J, Evans R, Pritzel A, et al. Highly accurate protein structure prediction with AlphaFold. Nature. 2021;596(7873):583–589. doi: 10.1038/s41586-021-03819-2
- Yan Y, Zhang D, Zhou P, et al. HDOCK: a web server for protein-protein and protein-DNA/RNA docking based on a hybrid strategy. Nucleic Acids Res. 2017;45:W365–W73. doi: 10.1093/nar/gkx407
- Aviv G, Gal-Mor O. lacZ Reporter System as a Tool to Study Virulence Gene Regulation in Bacterial Pathogens. Methods Mol Biol. 2018;1734:39–45.
- Tang Y, Zhang X, Wu W, et al. Inactivation of the sodA gene of Streptococcus suis type 2 encoding superoxide dismutase leads to reduced virulence to mice. Vet Microbiol. 2012;158(3–4):360–366. doi: 10.1016/j.vetmic.2012.02.028