ABSTRACT
Micro RNAs (miRNAs) have been implicated in the regulation of maturation, proliferation, differentiation, and activation of immune cells. In this study, we demonstrated that miR-29a antagonizes IFN-γ production at early times post-LSDV infection in cattle. miR-29a was predicted to target upstream IFN-γ regulators, and its inhibition resulted in enhanced IFN-γ production in sensitized peripheral blood mononuclear cells (PBMCs). Further, stimulation of PBMCs with LSDV antigen exhibited lower levels of miR-29a, concomitant with a potent cell-mediated immune response (CMI), characterized by an increase in LSDV-specific CD8+ T cell counts and enhanced levels of IFN-γ, which eventually facilitated virus clearance. In addition, a few immunocompromised cattle (developed secondary LSDV infection at ~ 6 months) that failed to mount a potent cell-mediated immune response, were shown to maintain higher miR-29a levels. Furthermore, as compared to the sensitized crossbred cattle, PBMCs from sensitized Rathi (a native Indian breed) animals exhibited lower levels of miR-29a along with an increase in CD8+ T cell counts and enhanced levels of IFN-γ. Finally, we analysed that a ≥ 60% decrease in miR-29a expression levels in the PBMCs of sensitized cattle correlated with a potent CMI response. In conclusion, miR-29a expression is involved in antagonizing the IFN-γ response in LSDV-infected cattle and may serve as a novel biomarker for the acute phase of LSDV infection, as well as predicting the functionality of T cells in sensitized cattle. In addition, Rathi cattle mount a more potent CMI response against LSDV than crossbred cattle.
Introduction
Lumpy skin disease (LSD) is characterized by high fever, development of nodules on the skin and internal organs, enlargement of lymph nodes, skin oedema, and emaciation, which may eventually result in a reduction in milk yield, sterility in bulls, damage to hides, and death [Citation1]. Recent outbreaks of LSD in Asian countries have been associated with high morbidity and mortality [Citation2].
LSD is caused by Capripoxvirus which belongs to the family Poxviridae. Two other capripoxviruses, sheep poxvirus (SPV) and goat poxvirus (GPV), which are responsible for the disease in sheep and goats, respectively, are antigenically indistinguishable from LSD virus (LSDV) [Citation2–4]. Attenuated LSDV strains are considered highly effective in inducing protection against LSD [Citation3–5]. Some countries also use SPV/GPV-based attenuated strains (heterologous strains) to induce protection against LSD in cattle [Citation6,Citation7]. However, heterologous vaccines exhibit poor efficacy, and are therefore generally discouraged by the World Organization for Animal Health (WOAH) for use in cattle against LSD [Citation8]. For several decades, LSDV has been restricted to Africa. Since the beginning of the 21st century, it has spread to several European countries and to the Middle East. In 2019, it spread to several countries in Asia [Citation9]. LSDV, particularly its immune response, has been poorly characterized. The antibody and IFNγ, the two important parameters that are used to correlate with humoral and cell-mediated immune responses (CMI), respectively, are variable following LSDV vaccination [Citation6]. A few vaccinated animals that do not develop detectable amounts of anti-LSDV antibodies and IFN-γ have been found to resist virulent challenge infections [Citation9–11]. In this context, each time a challenge test is required to ascertain the immunogenicity/efficacy of an individual vaccine batch. Therefore, a reliable biomarker (other than antibodies and IFN-γ) is required to correlate the protection offered by the LSD vaccines.
miRNAs are a class of approximately 22-nucleotide long non-coding RNAs [Citation12] that can influence gene expression [Citation13]. miRNAs have been shown to regulate various physiological and pathological processes such as cell proliferation and differentiation, development, angiogenesis, inflammation, cancer, and apoptosis [Citation13–21]. miRNAs have also emerged as crucial regulators of the innate and adaptive immune responses [Citation22–26]. We recently conducted miRNA profiling of LSDV-infected primary lamb testicular cells at various time points post-infection and identified several dysregulated miRNAs [Citation27]. However, the specific roles of miRNAs in LSDV replication and pathogenesis remain unclear.
Cattle serve as the principal host for LSDV infection. Evidence of LSDV infection has been observed in several other domestic/wild animals, such as camels [Citation6], giraffes [Citation28], Arabian oryx [Citation29] and African buffaloes [Citation30,Citation31]. However, the disease in these unnatural hosts is usually mild in nature [Citation6,Citation28–31]. Several field studies have shown that the risk of LSD is higher in Bos taurus than in Bos indicus and that crossbred cattle are more prone to LSD than native African breeds [Citation32–34]. During the 2022 outbreak of LSD in India, higher morbidity and mortality were observed in the crossbred (Holstein-Friesian) than in the indigenous (Rathi) cattle breed in the Nohar district of Rajasthan (India). Previous studies on some viral infections have established a link between miRNAs and resistance or susceptibility to the disease [Citation35,Citation36]. For example, miR-491 and miR-34a have been shown to determine the susceptibility of inbred mouse lines to influenza A (H7N9) infection [Citation37]. Likewise, goats showing greater susceptibility to peste des petites ruminants virus (PPRV) than sheep were shown to attain high miR-3 levels [Citation38]. Despite numerous clinical observations, the mechanisms underlying innate resistance to LSD in native African and Indian cattle breeds remain unknown. This study provides experimental evidence for breed susceptibility to LSDV infection.
Materials and methods
Ethics Statement
This study was approved by the National Research Centre on Equines, Indian Council of Agricultural Research, Hisar, India. This study included only the collection of blood (5 ml) from cattle and did not involve any invasive procedures. Consent was obtained from the concerned farmer wherever required. Serum and peripheral blood mononuclear cells (PBMCs) from a controlled experimental trial in cattle were available and have been previously described by our group. [Citation39]
Animals
Animals from Rathi and Sahiwal, the indigenous (native Indian) cattle breeds, belong to the Livestock Research Station and Dadhich cattle farm Nohar, Rajasthan (India). The crossbred cattle also belonged to Nohar town; animals from the Shri Radha Krishan Dairy Farm and Durga Colony were included in the study. All of these farms experienced an LSD outbreak in the preceding year (~7 months ago), and most of the existing adult animals had a history of LSD at the time of sample collection. Past exposure to LSD was confirmed by the presence of anti-LSDV antibodies in the serum. Five animals from each breed with an LSDV neutralizing antibody titre ≥ 32 were selected for immune response analysis.
To analyse the anamnestic immune response, five cattle that developed secondary LSDV infection at ~7 months post-primary infection (termed immunocompromised) were made available by Ganga Gaushala (cowshelter), Nokha (India). From the same herd, five apparently healthy animals that resisted secondary LSDV infection, had a history of LSDV infection (~7 months ago), and had anti-LSDV antibodies in serum (termed immunocompetent) were also included in the study.
Cells
Primary lamb testicle cells [Citation40] and African green monkey kidney (Vero) cells [Citation41] were available at the National Center for Veterinary Type Cultures (NCVTC), Hisar, and were grown in Dulbecco’s Modified Eagle’s medium (DMEM) supplemented with antibiotics and 10–15% foetal calf serum. Peripheral blood mononuclear cells were separated from the cattle blood using Histopaque®-1077 Hybri-MaxTM (Sigma, Steinheim, Germany) and cultured in RPMI 1640 (Lonza, Walkersville, MD, USA) supplemented with antibiotics and 10% foetal calf serum.
Virus
LSDV was isolated by our group in 2019 from an outbreak in cattle in Ranchi (India) and has been described elsewhere [Citation1].
Determination of LSDV neutralizing antibodies
Serum samples were heated at 56°C for 30 min to inactivate the complements. Vero cells were grown in 96 well tissue culture plates until they reached ~ 90 confluency. Two-fold serum dilutions (in 50 µL volume) were prepared in PBS and incubated with ~ 102 TCID50 of LSDV for 1 h at 37°C. The virus-antibody mixture was then incubated with Vero cells. At day 3–4 post-infection, cells were observed under a microscope for the appearance of CPE for the determination of antibody titres.
Stimulation of PBMCs
The PBMCs were isolated by using Histopaque®-1077 Hybri-MaxTM (Sigma, Steinheim, Germany) density gradient centrifugation according to the instructions of manufacturer and resuspended in RPMI 1640 (Sigma, St Louis, USA) supplemented with 10% heat-inactivated foetal calf serum. Cell density was adjusted to 5 × 106 cells/well in a 96 well plate. Live LSDV has toxic effect on PBMCs [Citation42]. Therefore, for stimulation, PBMCs were treated with UV-inactivated-LSDV antigen (2 µg/ml) at 37°C in 5% CO2 for 36 h.
miRNA inhibitor
BovinemiR-29ainhibitor(5’-mA/ZEN/mAmCmAmCmUmGmAmUmUmUmCmAmAmAmUmGmGmUmGmCmU/3ZEN–3’) was custom synthesized at Integrated DNA Technologies (IDT). miRNA inhibitors are single-stranded oligonucleotides that are perfectly complementary to the mature miRNA target and comprised of 2’-O-methyl residues that confer increased binding affinity to RNA targets and resistance to endonuclease degradation. ZEN modifications are included to block exonuclease degradation. miRNA inhibitors form a duplex with the miRNA guide strand that prevents the miRNA from binding to its intended target. Negative control (NC5 negative control inhibitor) was also procured from IDT. We used 100 nM final concentration of miRNA inhibitor, which was not found to be toxic as determined by the MTT [Citation6].
Evaluation of the impact of miR-29a inhibition on IFN-γ expression
Blood was collected from cattle at 6 months post-vaccination of a live-attenuated lumpy skin disease vaccine (Lumpi-ProVacInd). The PBMCs were isolated by using Histopaque®-1077 Hybri-MaxTM (Sigma, Steinheim, Germany) density gradient centrifugation. Cells were seeded into 6-well plates. The miR-29a inhibitor (100 nM final concentration) or negative control (Integrated DNA Technologies, Inc.) were transfected using the TransIT-siQUEST reagent (Mirus Bio, Madison, USA) according to the manufacturer’s instructions. After incubation for 24 h, cells were washed with PBS and stimulated with UV-inactivated LSDV antigen (2 µg/ml). The levels of IFN-γ released in the supernatant at 36 h post-stimulation were measured by the Bovine IFN-γ-ELISA kit (Invitrogen, Frederick, USA) according to the manufacturer’s instructions.
Cell proliferation assay
Fifty microlitres of MTT dye (5 mg/ml stock) were added to each well of 96-well plate having PBMCs and incubated at 37°C for 4 h. Finally, 100 µl of DMSO was added to dissolve the formazan crystals. The absorbance (OD) was measured at 570 nm, and the relative increase in cell number in LSDV-antigen stimulated over unstimulated cells was determined.
FACS analysis
The PBMCs were incubated at 4°C for 30 min with 50 μl of each of the corresponding monoclonal antibodies, which included Mouse anti-bovine-CD4:RPE (BIORAD, USA) (1:250 dilution) or Mouse anti-Bovine CD8:FITC (Invitrogen, Rockford, USA) (1:250 dilution). At the end of the incubation period, the cells were rinsed twice with pre-chilled FACS (PBS with 0.5% BSA) buffer and centrifuged for 3 min at 250xg. The cells were fixed with 200 μl of 1% paraformaldehyde. Flow Cytometry was performed using a CytoFLEX Flow Cytometer (Beckman Coulter Life Sciences). All results were analysed using FlowJoTM10 software. Prior to each experiment, compensation was conducted using CytoFLEX Daily QC Fluorospheres (Beckman Coulter Life Sciences). Gating was initially performed around the cell population of interest (lymphocytes) using forward and side scatter properties. The percentage of CD8+ or CD4+ cells was determined using the fluorescence intensity of the respective dyes on forward scatter (FSC). The relative increase in the fold-change in CD4+ or CD8+ cell counts in LSDV antigen-stimulated cells compared to un-stimulated cells was determined.
Measurement of IFN-γ
The serum levels of IFN-γ were determined using the Bovine IFN-γ-ELISA kit (Invitrogen, Frederick, USA) according to the manufacturer’s instructions.
Quantitation of miRNAs by qRT-PCR
RNA was isolated using TRIzol reagent. The total RNA concentration in each sample was normalized to 1 µg RNA. Poly (A) tailing of RNA was performed using E. Coli Poly (A) Polymerase (New England Biolabs, United States) according to the manufacturer’s instructions. Briefly, a 20 µl reaction volume contained 1 µg RNA, 2 µl of 10X E. coli Poly (A) Polymerase Reaction Buffer, 2 µL of ATP (10 mM), and 1 µL of E. Coli Poly (A) Polymerase. The reaction mixtures were incubated at 37°C for 1 h and at 70°C for 15 min. cDNA of poly (A)-tailed RNA was prepared using an oligo adaptor primer (OAP) () according to the manufacturer’s instructions (Invitrogen, MA, United States).
Table 1. List of primers used for quantitation of miRNAs, IFN-γ and endogenous control.
cDNA amplification was conducted by qRT-PCR using miRNA-specific forward primers and Universal Adaptor primers (). The thermal cycler conditions were as follows: initial denaturation at 95°C for 10 min, followed by 45 cycles of denaturation at 95°C for 15 s, and annealing at 60°C for 60 s. The miRNA expression levels were calculated using the 2−ΔΔCt method and normalized to the respective U6 (endogenous control) expression levels. Differences in the expression levels of miRNAs were compared using Student’s t-test.
Results
Downregulation of circulating miR-29a in LSDV-infected animals
Recently, we performed miRNA profiling of LSDV-infected primary lamb testicular cells and identified several LSDV-associated dysregulated miRNAs. This, together with a previous report on the association of some miRNAs in regulating immune response [Citation43–46], we selected three miRNAs viz: mi-R29a, mi-R221a, and mi-R27a, and evaluated their levels in LSDV-infected and uninfected cattle at 3 days post-infection (dpi). As shown in , mi-R29a levels (), but not miR-221a () or mi-R27a () levels were lower in infected animals. This confirmed the downregulation of mi-R29a in the LSDV-infected cattle.
Figure 1. Downregulation of circulating miR-29a in LSDV-infected cattle. Serum from LSDV-infected (n = 5) or uninfected animals (n = 5) was collected at 3 dpi (experimental infection) and subjected for RNA isolation. The RNA was subjected to poly(A) tailing and cDNA synthesis by using oligo-adaptor primer. The levels of miRNAs in serum were detected by qRT-PCR using a universal adaptor primer and miRNA-specific forward primers. The relative fold change in miR-29a (a), miR-221 (b) and miR-27a (c) levels was determined by qRT-PCR. The miRNA expression levels were calculated using the 2−ΔΔCt method and were normalized to the respective U6 (endogeneous control) expression levels. The differences in the levels of miRNAs were compared using student’s t-test. Values are means ± SD. p value indicates the level of statistically significant difference.
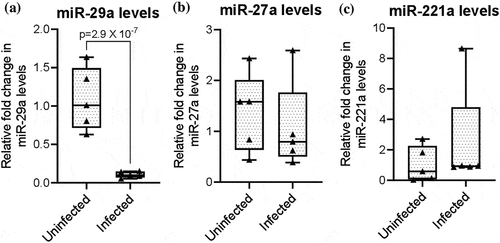
miR-29a negatively regulates the IFN-γ response in LSDV-infected cattle
IFN-γ is a major cytokine critical for innate and adaptive immunity against viral infections [Citation47]. We evaluated the levels of mi-R29a and IFN-γ at various times post-LSDV infection in experimentally challenged cattle. The miR-29a level was highest (basal level) at 0 dpi and progressively decreased at 3 and 9 dpi (). In contrast, the levels of IFN-γ, which were at basal levels at 0 dpi, progressively increased at 3 and 9 dpi in the PBMCs () and in the serum (). At 15 dpi, both miR-29a and IFN-γ levels tended to reach basal levels (). Bioinformatics analysis using TargetScan (v7.0; targetscan.org) predicted the miR-29a-binding site on the IFN-γ-inducing transcription factors T-bet (T-box transcription factor) and EOMES (Eomesodermin) at their 3’-untranslated region (UTR) (), suggesting an indirect action of miR-29a on IFN-γ production. Furthermore, we provided experimental evidence in support of the inhibition of IFN-γ by miR-29a. We used 100 nM of miR-29a inhibitor, which was not found to be toxic, as the cell viability of the PBMCs was comparable in untreated, negative control-treated, and miR-29a inhibitor-treated cells (). In order to evaluate the effect of miR-29a inhibition on IFN-γ, PBMCs were transfected with the negative control or miR-29a inhibitor, followed by stimulation with inactivated LSDV antigen. As shown in , the supernatant from miR-29a inhibitor-treated cells had significantly higher levels of IFN-γ as compared to the negative control-treated cells. Taken together, it was concluded that miR-29a negatively regulates IFN-γ production in LSDV-sensitized immune cells.
Figure 2. miR-29a negatively regulates IFN-γ response in LSDV-infected cattle. Blood/serum samples from cattle were collected at the indicated times post-LSDV infection. (a) miR-29a. RNA from PBMCs was subjected to poly(A) tailing, cDNA synthesis, and qRT-PCR using a universal adaptor primer and a miR-29a-specific forward primer (). The miRNA expression levels were calculated using the 2−ΔΔCt method and normalized to the respective U6 (endogenous control) expression levels. (b) IFN-γ in PBMCs. RNA from PBMCs was subjected to cDNA synthesis, and IFN-γ levels were determined by qRT-PCR. (c) IFN-γ in serum. The IFN-γ levels in serum were determined by the Bovine IFN-γ-ELISA kit. Differences in the levels of miR-29a/IFN-γ at various days post-infection were compared using Student’s t-test. Values are means ± SD. The p value indicates the level of statistically significant difference. (d) miR-29a binds to IFN-γ-inducing transcriptional factors EOMES and T-bet. The miR-29a binding sites on IFN-γ-inducing transcriptional factors EOMES and T-bet were identified by pairing the miRNA seed region and complementary sites within target mRNAs using TargetScan. The context score and position of miR-29a binding to EOMES and T-bet mRNA are shown. (e) Evaluation the cytotocity of miR-92a inhibitor. The PBMCs, in triplicates, were transfected with bovine miR-29a inhibitor or negative control and cultured for 72 h. The cytotoxicity was determined by the MTT assay. The % cell viability was measured by comparing it with the untransfected control. (f) Effect of miR-29a inhibition on IFN-γ expression. The PBMCs from sensitized cattle were transfected with bovine miR-29a inhibitor or a negative control. After incubation for 24 h, cells were either unstimulated or stimulated with UV-inactivated LSDV antigen. The level of IFN-γ released in the supernatant at 36 h post-stimulation was measured by the Bovine IFN-γ-ELISA kit. The IFN-γ levels were normalized with the respective unstimulated controls. The relative levels of IFN-γ secreted in miR-29a inhibitor-treated- and negative control-treated PBMCs are shown. Values are means ± SD. The “p” value indicates the level of a statistically significant difference.
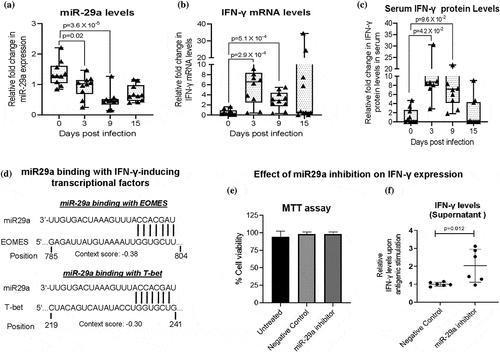
Among the numerous dysregulated miRNAs that were identified in LSDV-infected primary lamb testicle cells [Citation27], at least five more novel miRNAs were predicted for target sites on genes associated with regulation of interferon induction (Supplementary Figure S1).
LSDV-specific CD8+ T cells play an important role in clearing LSDV infection
The antibody response following LSDV exposure in cattle is variable [Citation6,Citation48]. A few animals that do not develop detectable amounts of antibodies can also resist challenge infections [Citation6]. This highlights the importance of CMI responses in LSDV infection. However, the CMI response is poorly characterized in LSDV infection [Citation6]. We screened some apparently healthy cattle from the field, and based on the presence or absence of anti-LSDV antibodies in serum, categorized them as LSDV-sensitized (LSDV-exposed) and LSDV-unsensitized (unexposed or naive), respectively (Supplementary Figure S2a). We then conducted FACS analysis of PMBCs upon stimulation with UV-inactivated LSDV (antigen) (). CD8+ T cell counts were significantly higher (~3.5 fold) in sensitized cattle than in unsensitized cattle (). Furthermore, the IFN-γ level in the supernatant of stimulated PBMCs was significantly higher in sensitized than in unsensitized cattle (). This suggests that LSDV induces the activation and proliferation of CD8+ T cells in sensitized cattle. We also analysed the CD4+ T cell counts. However, no significant difference was observed in CD4+ T cell counts between the sensitized and unsensitized cattle ().
Figure 3. LSDV-specific CD8+ T cells play an important role in clearing virus infection. Equal numbers of PBMCs from LSDV-sensitized and unsensitized cattle were treated with UV-inactivated LSDV antigen and incubated for 36 h at 37°C. The cells were then stained with the respective antibodies. Flow cytometry was performed using a CytoFLEX flow Cytometer, and the results were analysed using FlowJoTM10 software. (a) The gating strategy for identification and quantification of CD8+ and CD4+ cells. Total lymphocytes were first gated in a forward scatter (Fsc)/side scatter (SSC) plot, and then a single-cell population was selected in an FSC-A/FSC-H plot. Single cells were subsequently gated for CD4 or CD8 expression. (b) Relative fold-change in CD8+ counts upon antigenic stimulation. (c) Relative IFN-γ levels in the supernatant following stimulation of PBMCs with the LSDV antigen. (d) Relative fold change in CD4+ counts upon antigenic stimulation. (e) Virus clearance: PBMCs were stimulated with LSDV antigen for 24 h. Thereafter, the stimulated PBMCs were incubated with LSDV-infected lamb testicular cells for 72 h. The LSDV particles in the resulting supernatant were quantified by determining the TCID50. values are means ± SD. The p value indicates the level of statistically significant difference.
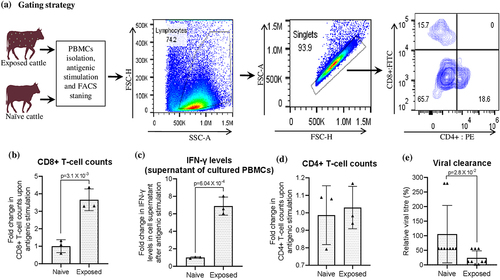
To further confirm the role of CD8+ T cells in clearing viral infection, we incubated LSDV-stimulated PBMCs with virus-infected lamb testicular cells, and the virus released in the infected cell culture supernatant at 72 hpi was quantified. As shown in , the virus titres in sensitized animals were ~ 90% lower than those in unsensitized animals, suggesting that LSDV-specific CD8+ T cells are involved in clearing viral infection. Taken together, these lines of evidence suggest that activated CD8+ T cells help clearing LSDV infection.
miR-29a antagonizes IFN-γ production in sensitized T cells
IFN-γ is produced by activated lymphocytes, mainly natural killer (NK) cells, NK T cells (NKT), CD4+ Th1 cells, and CD8+ T cells, and is a critical component of the CMI response to viral infection. Since miR-29a expression was negatively correlated with IFN-γ (), we further evaluated the correlation between miR-29a expression and lymphoproliferation, CD8+ T cell counts in sensitized and unsensitized cattle (Supplementary Fig. S2b). Similar to experimental infection, the level of miR-29a (in stimulated PBMCs) in field cattle was also lower in LSDV-sensitized cattle than in unsensitized cattle () and negatively correlated with the levels of IFN-γ (), lymphoproliferation (), and CD8+ T cell count (). This suggests that suppression of miR-29a in lymphocytes is essential for producing optimal levels of IFN-γ in LSDV-sensitized cattle.
Figure 4. miR-29a antagonizes IFN-γ production in sensitized T cells. Equal numbers of PBMCs from LSDV-sensitized and unsensitized cattle were treated with UV-inactivated LSDV antigen for 36 h. The half of the cells were subjected for RNA isolation while reaming cells were used for FACS analysis. The relative levels of miR-29a (a), IFN-γ (b), and Lymphorolifieration (c) as well as FACS plot (d) and CD8+ T cell counts (e) is shown. Values are means ± SD. p value indicates the level of statistically significant difference.
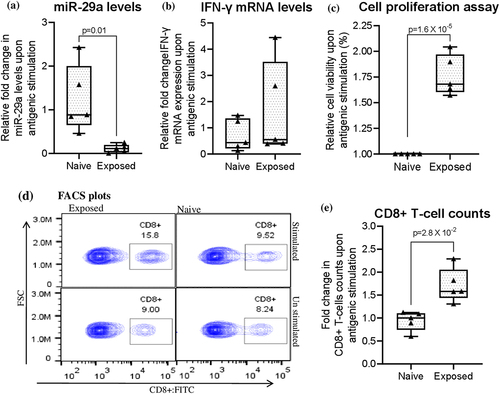
Furthermore, we analysed the correlation between miR-29a expression levels and the CMI response. Interestingly, a detectable CMI response (significant increase in virus-specific CD8+ T cell counts along with a significant increase in IFN-γ levels) was observed only in those animals that had > 60% reduction in miR-29a expression levels in the stimulated PBMCs ().
Table 2. miR-29a expression in sensitized PBMCs and its correlation with cell-mediated immune response.
miR-29a expression levels in immunocompetent and immunocompromized cattle
Natural LSDV infection in cattle induces long-lasting immunity that may persist for up to two years [Citation3,Citation49]. However, we observed that some (1.4%; 6 out of 428) of the recovered animals from the 2022 outbreak succumbed to a secondary LSDV infection at ~7 months following the primary infection. Secondary infection was confirmed by the presence of fresh nodular skin lesions (Supplementary Fig S3a) in the affected cattle, virus isolation (Supplementary Fig S3b) and detection of the LSDV genome (Supplementary Fig S3c) in the skin nodules. The animals that developed secondary infection were termed immunocompromised animals, whereas those in contact with animals that resisted secondary infection were termed as immunocompetent. The immunocompetent animals had a history of LSDV infection (~7 months ago), skin patches (remnant of previous infection) (Supplementary Fig S3d) and presence of anti-LSDV antibodies in serum (Supplementary Fig S3e). At the time of sample collection (secondary infection), the immunocompromised and immunocompetent animals had anti-LSDV antibody titres in the range of < 4–8 and 16–64, respectively (Supplementary Fig S3e).
When PBMCs were stimulated with the LSDV antigen, as compared to the immunocompromised animals, immunocompetent animals showed higher levels of IFN-γ () and an increase in CD8+ T cell count (), in addition to exhibiting a significant lymphoproliferative effect (). Interestingly, miR-29a levels were higher in immunocompromised animals than in immunocompetent ones (). This suggests that miR-29a may be involved in the suppression of CD8+ T cell function and, consequently, reduced IFN-γ production in immunocompromised animals.
Figure 5. miR-29a expression levels and immune response in immunocompetent and immunocompromized cattle. Equal amount of PBMCs from immunocompetent (n = 5) and immunocompromized (n = 5) cattle were stimulated with UV-inactivated LSDV antigen for 36 h. Half of cells were subjected for RNA isolation and reaming cells were subjected for lymphoproliferation and FACS analysis. (a) Relative miR-29a levels in PBMCs. (b) FACS plot depicting CD8+ T cell count in stimulated and unstimulated PBMCs. (c) Relative fold change in CD8+ T cells counts upon antigenic stimulation. Ratio of CD8+ T cells counts in stimulated over unstimulated cells is shown. (d) Relative lymphoproliferation. (e) Relative IFN-γ levels in PBMCs. Values are means ± SD. p value indicates the level of statistically significant difference.
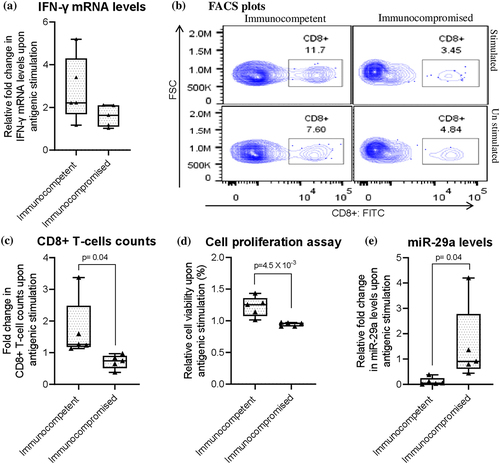
The Rathi breed of cattle mounts a more potent CMI response than crossbred cattle
The 2022 LSD outbreaks in India had a devastating impact on animal health and the livelihoods of farmers. However, susceptibility to the disease was shown to be much higher in certain cattle breeds than in other breeds. A small survey in Nohar (a district in the western part of India), the morbidity rates in Rathi, Sahiwal, and crossbred cattle was observed to be 13.79%, 33.33%, and 87.77%, respectively (). Similarly, the case fatality rates was 27.27%, 29.17%, and 69.51%, respectively, in Rathi, Sahiwal and crossbred cattle ().
Figure 6. The Rathi breed of cattle mounts a more potent CMI response than crossbred cattle. (a) Morbidity and case fatality rate in Rathi, Sahiwal and crossbred cattle. in 2022, there was a severe LSD outbreak in India, particularly the Western part of the country. Morbidity and case fatality rate in Rathi, Sahiwal and crossbred cattle in Nohar (a town in Western part of India), is shown. (b) Analysis of the miR-29a and CMI response in sensitized Rathi, Sahiwal and crossbred cattle. equal amount of PBMCs from Rathi, Sahiwal and crossbred cattle (n = 5 each) were treated with UV-inactivated LSDV antigen and incubated for 36 h. Half of the cells were subjected for RNA isolation and reaming cells were subjected for lymphoproliferation and FACS analysis. (b1) Relative miR-29a levels in PBMCs. (b2) Relative IFN-γ levels in PBMCs. (b3) FACS plot depicting CD8+ T cell count in stimulated and unstimulated PBMCs. (b4) relative fold change in CD8+ T cells counts upon antigenic stimulation. Ratio of CD8+ T cells counts in stimulated over unstimulated cells is shown. (b5) relative lymphoproliferation. Values are means ± SD. p value indicates the level of statistically significant difference.
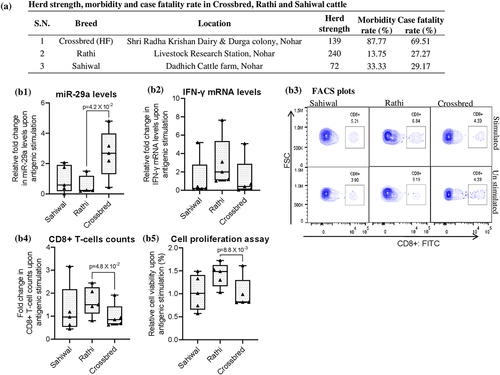
In order to provide insights into the association between miR-29a and breed susceptibility to LSDV infection, Rathi, Sahiwal, and crossbred cattle that had LSD history (~7 months ago) and had a virus neutralizing antibody titre of ≥ 32 were included in the study (Supplementary Fig S2c). PBMCs were stimulated with UV-inactivated LSD antigen and analysed for miR-29a expression, lymphoproliferation, and CD8+ T cell counts. Upon antigenic stimulation, compared to the crossbred animals, the PBMCs from sensitized Rathi animals expressed lower levels of miR-29a () but had higher levels of IFN-γ (), increased CD8+ T cell counts (), and exhibited a significant lymphoproliferative effect (). Sahiwal animals appear to express an intermediate phenotype (as compared to Rathi and crossbred cattle), but without any significant difference in the magnitude of the immune response against LSDV. Taken together, it was concluded that compared to crossbred cattle, the Rathi breed of cattle mounts a more potent CMI response by antagonizing the miR-29a response more effectively.
Discussion
In addition to regulating various physiological, metabolic, and developmental processes, miRNAs have been shown to regulate virus replication and immune responses [Citation50,Citation51]. We selected virus-associated dysregulated miRNAs [Citation27,Citation52,Citation53] and confirmed the downregulation of circulating miR-29a levels in LSDV-infected cattle. miR-29a expression in NK cells, CD4+ T cells, and CD8+ T cells from Mycobacterium tuberculosis-infected mice was previously shown to suppress IFN-γ-production [Citation52,Citation54]. In our study, miR-29a expression progressively decreased from 0 to 9 dpi in LSDV-infected cattle. In contrast, IFN-γ levels progressively increased from 0 to 9 dpi in infected cattle. Nevertheless, IFN-γ-inducing transcriptional factors, such as T-bet and EOMES, share a conserved miR-29a target site at their 3’ untranslated region (UTR) [Citation54–56]. This was further confirmed by transfection of miR-29a inhibitor, wherein sensitized PBMCs produced enhanced levels of IFN-γ. Taken together, it was concluded that miR-29a expression is indirectly involved in antagonizing the IFN-γ response in LSDV-infected cattle, and that reduced miR-29a expression may serve as a novel biomarker during the acute phase of LSDV infection. Among the hundreds of dysregulated miRNAs that have been identified in cells infected with LSDV [Citation27], at least five more novel miRNAs were predicted to have target sites in genes that are associated with interferon regulation. Their further validation, along with elucidating their role in LSDV infection, is essential and needs further investigation.
Next, we hypothesized a link between miR-29a expression and CD8+ T cell activation and proliferation following LSDV exposure in cattle. There are few publications on the CMI response to LSDV infection [Citation42,Citation57–59] and the information pertaining to the CMI response to LSDV is very limited. Therefore, we first provided insights into CMI by analysing the PBMCs of cattle recovered from LSDV infection in the field. Antigenic stimulation of sensitized PBMCs resulted in an increased CD8+ T cell count, but without any significant effect on the CD4+ T cell count. The stimulated cells (PBMCs) from LSDV-sensitized cattle effectively cleared the LSDV infection compared to the unsensitized cells. Since the increased CD8+ T cell count was also associated with increased IFN-γ levels in the supernatant of antigen-stimulated cells, it was concluded that CD8+ T cells play a major role in CMI response and hence in clearing LSDV infection [Citation60–65].
In addition to directly evaluating the miR-29a response in experimentally LSDV-challenged cattle, we screened field cattle that were previously (~7 months ago) naturally exposed to LSDV infection. Upon antigenic stimulation, PBMCs from sensitized cattle expressed significantly lower levels of miR-29a compared to naïve cattle, but exhibited higher levels of IFN-γ, a significant lymphoproliferative effect, and an increased CD8+ T cell count. miR-29a is expressed at a basal level in naïve T cell. It is downregulated when naïve T cells convert into effector T cells and returns to basal levels when they convert into memory T cells [Citation66,Citation67]. This is essential to ensure the persistence of effector T-cells in the form of memory T-cells, and to prevent exhaustion and apoptosis [Citation68]. Therefore, besides serving as an immunological marker for T cell functionality, miR-29a may serve as a target for immunotherapy [Citation66–68]. Because the levels of antibody and IFN-γ responses in LSD vaccinated animals are variable, the immunogenicity/efficacy of the LSD vaccine is ideally evaluated by the challenge of vaccinated animals with a virulent virus [Citation6,Citation9–11]. However, this is cumbersome and requires a containment facility. However, a suitable biomarker that can be used to correlate the immunogenicity of vaccines in cattle is lacking. We observed that upon antigenic stimulation, >60% reduction in miR-29a expression levels in the PBMCs of the sensitized cattle correlates with a potent CMI response (significant lymphoproliferation as well as, increased CD8+ T cell count, and enhanced levels of IFN-γ). This may supplement the prediction of immune protection in sensitized cattle, particularly in animals that do not develop detectable levels of antibodies and IFN-γ. However, this requires additional data from a large number of controlled experimental trials.
Being a poxvirus, LSDV also induces long-lasting immunity in animals that either recover from natural infection (~2 years) [Citation3] or receive a live-attenuated vaccine (1–1.5 years) [Citation69]. The LSD has devastated the Indian livestock economy. During the 2022 outbreaks of LSDV, over 200,000 cattle died. The recovered animals had a drastic reduction in milk yield. In addition, it induces abortions in pregnant cattle and sterility in bulls. Approximately seven months after the first cycle of the outbreak, we confirmed the secondary cycle of LSDV infection in some cattle that recovered from the 2022 outbreak, as evidenced by the presence of skin nodules in the affected cattle and the detection of the virus/viral genome in the skin nodules. This was intriguing and led us to investigate the immune status of these animals. Five animals that succumbed to secondary LSDV infection (termed immunocompromised animals) and five apparently healthy animals from the same herd but had a history of clinical LSD (termed immunocompetent) were examined. miR-29a has previously been shown to be associated with immunosuppression in HIV-1 infected individuals [Citation70]. Compared to immunocompetent cattle, immunocompromised cattle had minimal or no detectable amount of anti-LSDV antibodies in their serum, and there was no significant increase in CD8+ T cell count upon antigenic stimulation of their PBMCs with LSDV antigen. Immunocompromised cattle were also not able to sufficiently antagonize miR-29a levels compared to immunocompetent cattle. It appears that T cells of immunocompromised animals undergo exhaustion and/or attrition. However, this requires further investigation.
One more interesting observation made by us during the 2022 outbreak of LSD in India was that indigenous cattle, particularly the Rathi breed of cattle showed lower morbidity (13.75%) and case fatality rate (27.27%) as compared to the crossbred cattle (87.77% morbidity and 69.51% case fatality). Upon antigenic stimulation, PBMCs from the Rathi breed showed a higher CD8+ T cell count and reduced miR-29a expression levels. This again indicates that miR-29a downregulation is essential to achieve an optimal CMI response, and that the indigenous Rathi breed of cattle mounts a more potent CMI response than crossbred cattle. The results from this and several other studies indicated that herds with native African and Indian cattle were at a lower risk of LSD than those with exotic breeds or crossbred animals [Citation32–34]. However, this may be partly influenced by differences in management practices, comorbidities, and altered susceptibility to the arthropod vectors that transmit the disease. Indigenous breeds of cattle may have the additional advantage of prolonged periods of natural selection, favouring individuals with greater resistance to local diseases. However, this may not be relevant for LSD in Kenyan (Africa) cattle breeds, as LSD was first recognized in Kenya in 1957 [Citation71], which is not far from the time exotic breeds were introduced into Kenya in 1902 [Citation72]. Likewise, in India, the crossbreeding program was initiated in 1910, and LSD was first reported in 2019 [Citation73]. In addition, subclinical infection may be more common in indigenous breeds, which requires further study in experimental and/or field settings. While this topic still remains open for further discussion and in-depth investigation, we shed some light on the scientific basis of breed susceptibility to LSDV infection in cattle for the first time.
This study has numerous limitations that should be highlighted. The numbers of native and crossbred cattle available for analysing morbidity and mortality/case fatality rates were limited and belonged to a particular geographical region in India. In addition, only a limited number of immunocompromised animals were available for analysing the anamnestic immune response to LSDV. Furthermore, a large number of controlled experimental trials are required to establish miR-29a as a biomarker for negatively correlating it with the immunogenicity of the LSD vaccine.
Conclusion
Our study in experimentally challenged and naturally infected cattle suggests that miR-29a expression is involved in antagonizing the IFN-γ response in LSDV-infected cattle and may serve as a novel biomarker for the acute phase of LSDV infection, as well as predicting the functionality of T cells in sensitized cattle. The indigenous Rathi breed of cattle mounts a more potent CMI response against the LSDV than crossbred cattle.
Author contributions
N.K., B.N.T., and S.S. conceived of and designed the study. R.K., N.K., A.V., S.S., S.B., Y.C., S.D., H.K., K.N, A.B. and R.K.D. collected the samples and performed laboratory experiments. S.S., R.K., and N.K. analysed the data. N.K. wrote the primary draft of the manuscript. All authors have reviewed the manuscript.
Supplemental Material
Download Zip (1.2 MB)Disclosure statement
No potential conflict of interest was reported by the author(s).
Data Availability statement
All the data are available within this manuscript.
Supplemental data
Supplemental data for this article can be accessed online at https://doi.org/10.1080/21505594.2024.2324711
Additional information
Funding
References
- Kumar N, Chander Y, Kumar R, et al. Isolation and characterization of lumpy skin disease virus from cattle in India. PLoS One. 2021;16(1):e0241022. doi: 10.1371/journal.pone.0241022
- Kumar N, Tripathi BN. A serious skin virus epidemic sweeping through the Indian subcontinent is a threat to the livelihood of farmers. Virulence. 2022;13(1):1943–14. doi: 10.1080/21505594.2022.2141971
- Tuppurainen E, Dietze K, Wolff J, et al. Review: vaccines and vaccination against lumpy skin disease. Vaccines (Basel). 2021;9(10):1136. doi: 10.3390/vaccines9101136
- Tuppurainen ES, Lubinga JC, Stoltsz WH, et al. Mechanical transmission of lumpy skin disease virus by Rhipicephalus appendiculatus male ticks. Epidemiol Infect. 2013;141(2):425–30. doi: 10.1017/S0950268812000805
- Tuppurainen ES, Oura CA. Review: lumpy skin disease: an emerging threat to Europe, the Middle East and Asia. Transbound Emerg Dis. 2012;59(1):40–8. doi: 10.1111/j.1865-1682.2011.01242.x
- Kumar N, Barua S, Kumar R, et al. Evaluation of the safety, immunogenicity and efficacy of a new live-attenuated lumpy skin disease vaccine in India. Virulence. 2023;14(1):2190647. doi: 10.1080/21505594.2023.2190647
- Kumar R, Chander Y, Verma A, et al. A novel HRM-based gap-qRT-PCR for identification and quantitation of the vaccine and field strain(s) of lumpy skin disease virus. J Immunol Methods. 2023;519:113521. doi: 10.1016/j.jim.2023.113521
- WOAH. The first South Asia transboundary animal diseases coordination meeting for PPR, FMD and LSD. Organized by, World Organization for animal Health (WOAH) and Food and Agricultural Organization (FAO) of the United Nations from May 7-12, 2023. 2023;1–35.
- Tuppurainen E, Oura C. Lumpy skin disease: an African cattle disease getting closer to the EU. Vet Rec. 2014;175(12):300–301. doi: 10.1136/vr.g5808
- Tuppurainen ES, Pearson CR, Bachanek-Bankowska K, et al. Characterization of sheep pox virus vaccine for cattle against lumpy skin disease virus. Antiviral Res. 2014;109:1–6. doi: 10.1016/j.antiviral.2014.06.009
- Zhugunissov K, Bulatov Y, Orynbayev M, et al. Goatpox virus (G20-LKV) vaccine strain elicits a protective response in cattle against lumpy skin disease at challenge with lumpy skin disease virulent field strain in a comparative study. Vet Microbiol. 2020;245:108695. doi: 10.1016/j.vetmic.2020.108695
- Zeng Y. Principles of micro-RNA production and maturation. Oncogene. 2006;25(46):6156–6162. doi: 10.1038/sj.onc.1209908
- VA PS, Kumar N, Kumar N. Role of miRNA in regulating virus replication. Animal Gene. 2023;30:200162. doi: 10.1016/j.angen.2023.200162
- Wang G, Cheng T, Yuan H, et al. Tracing cellular interaction of circRNA-miRNA axis with Cu metal-organic framework supported DNA cascade assembly. Biosens Bioelectron. 2023;228:115226. doi: 10.1016/j.bios.2023.115226
- Liao Z, Zheng R, Shao G. Mechanisms and application strategies of miRNA‑146a regulating inflammation and fibrosis at molecular and cellular levels (review). Int J Mol Med. 2023;51(1):51. doi: 10.3892/ijmm.2022.5210
- Girigoswami K, Girigoswami A. A review on the role of nanosensors in detecting cellular miRNA expression in colorectal cancer. Endocrine, metabolic & immune disorders drug targets. Endocr Metab Immune Disord Drug Targets. 2021;21(1):12–26. doi: 10.2174/1871530320666200515115723
- Zeng G, Wang Z, Huang Y, et al. Cellular and viral miRNA expression in polyomavirus BK infection. Transpl Infect Dis. 2019;21(5):e13159. doi: 10.1111/tid.13159
- Li J, Li X, Kong X, et al. MiRNA-26b inhibits cellular proliferation by targeting CDK8 in breast cancer. Int J Clin Exp Med. 2014;7:558–565.
- Bernier A, Sagan SM. The diverse roles of microRnas at the host–virus interface. Viruses. 2018;10(8):440. doi: 10.3390/v10080440
- Condrat CE, Thompson DC, Barbu MG, et al. miRnas as biomarkers in disease: latest findings regarding their role in diagnosis and prognosis. Cells. 2020;9(2):276. doi: 10.3390/cells9020276
- Babar IA, Slack FJ, Weidhaas JB. miRNA modulation of the cellular stress response. Future Oncol. 2008;4:289–298. doi: 10.2217/14796694.4.2.289
- Boss IW, Renne R. Viral miRNAs: tools for immune evasion. Curr Opin Microbiol. 2010;13(4):540–545. doi: 10.1016/j.mib.2010.05.017
- Momen‐Heravi F, Bala S. miRNA regulation of innate immunity. J Leukoc Biol. 2018;103:1205–1217. doi: 10.1002/JLB.3MIR1117-459R
- Aslani M, Mortazavi-Jahromi SS, Mirshafiey A. Cytokine storm in the pathophysiology of COVID-19: possible functional disturbances of miRnas. Int Immunopharmacol. 2021;101:108172. doi: 10.1016/j.intimp.2021.108172
- Sullivan CS, Grundhoff AT, Tevethia S, et al. SV40-encoded microRNAs regulate viral gene expression and reduce susceptibility to cytotoxic T cells. Nature. 2005;435(7042):682–686. doi: 10.1038/nature03576
- Kumar N, Barua S, Thachamvally R, et al. Systems perspective of morbillivirus replication. J Mol Microbiol Biotechnol. 2016;26(6):389–400. doi: 10.1159/000448842
- Pandita S, Verma A, Kamboj H, et al. miRNA profiling of primary lamb testicle cells infected with lumpy skin disease virus. Arch Virol. 2023;168(12):290. doi: 10.1007/s00705-023-05917-0
- Dao TD, Tran LH, Nguyen HD, et al. Characterization of lumpy skin disease virus isolated from a giraffe in Vietnam. Transbound Emerg Dis. 2022;69(5):e3268–e3272. doi: 10.1111/tbed.14583
- Greth A, Gourreau JM, Vassart M, et al. Capripoxvirus disease in an Arabian oryx (Oryx leucoryx) from Saudi Arabia. J Wildl Dis. 1992;28(2):295–300. doi: 10.7589/0090-3558-28.2.295
- Hedger RS, Hamblin C. Neutralising antibodies to lumpy skin disease virus in African wildlife. Comp Immunol Microbiol Infect Dis. 1983;6(3):209–213. doi: 10.1016/0147-9571(83)90012-7
- Fagbo S, Coetzer JA, Venter EH. Seroprevalence of rift valley fever and lumpy skin disease in African buffalo (syncerus caffer) in the Kruger National Park and Hluhluwe-IMfolozi park, South Africa. J S Afr Vet Assoc. 2014;85:e1–e7. doi: 10.4102/jsava.v85i1.1075
- Kiplagat SK, Kitala PM, Onono JO, et al. Risk factors for outbreaks of lumpy skin disease and the Economic Impact in Cattle Farms of Nakuru County, Kenya. Front Vet Sci. 2020;7:259. doi: 10.3389/fvets.2020.00259
- Abera Z, Degefu H, Gari G, et al. Sero-prevalence of lumpy skin disease in selected districts of West Wollega zone, Ethiopia. BMC Vet Res. 2015;11(1):135. doi: 10.1186/s12917-015-0432-7
- Khalafalla AI, Gaffar Elamin MA, Abbas Z. Récentes épidémies de dermatose nodulaire contagieuse bovine observées au Soudan. Rev Elev Med Vet Pays Trop. 1993;46(4):548–550. doi: 10.19182/remvt.9408
- Tang Q, Y-Q W, Chen D-S, et al. Bovine herpesvirus 5 encodes a unique pattern of microRNAs compared with bovine herpesvirus 1. J Gen Virol. 2014;95(3):671–678. doi: 10.1099/vir.0.061093-0
- Tian F, Luo J, Zhang H, et al. MiRNA expression signatures induced by Marek’s disease virus infection in chickens. Genomics. 2012;99:152–915. doi: 10.1016/j.ygeno.2011.11.004
- Bao S, Jia L, Zhou X, et al. Integrated analysis of mRNA-seq and miRNA-seq for host susceptibilities to influenza a (H7N9) infection in inbred mouse lines. Funct Integr Genomics. 2018;18(4):411–424. doi: 10.1007/s10142-018-0602-3
- Kaufmann SH, Dorhoi A, Hotchkiss RS, et al. Host-directed therapies for bacterial and viral infections. Nat Rev Drug Discov. 2018;17:35–56. doi: 10.1038/nrd.2017.162
- Gari G, Abie G, Gizaw D, et al. Evaluation of the safety, immunogenicity and efficacy of three capripoxvirus vaccine strains against lumpy skin disease virus. Vaccine. 2015;33(28):3256–3261. doi: 10.1016/j.vaccine.2015.01.035
- Kumar N, Wadhwa A, Chaubey KK, et al. Isolation and phylogenetic analysis of an orf virus from sheep in Makhdoom, India. Vir Gen. 2014;48(2):312–319. doi: 10.1007/s11262-013-1025-9
- Khandelwal N, Chander Y, Rawat KD, et al. Emetine inhibits replication of RNA and DNA viruses without generating drug-resistant virus variants. Antiviral Res. 2017;144:196–204. doi: 10.1016/j.antiviral.2017.06.006
- ND W The role of cell-mediated immunity in the pathogenesis of experimental lumpy skin disease virus Ph D Thesis submitted to School of Veterinary Medicine and Sciences, University of Surrey 2022.
- Abu-Izneid T, AlHajri N, Ibrahim AM, et al. Micro-RNAs in the regulation of immune response against SARS CoV-2 and other viral infections. J Adv Res. 2021;30:133–45. doi: 10.1016/j.jare.2020.11.013
- Ostrycharz E, Hukowska-Szematowicz B. Micro-players of great significance—Host microRNA signature in Viral infections in Humans and animals. Int J Mol Sci. 2022;23(18):23. doi: 10.3390/ijms231810536
- Yang X, Liang Y, Bamunuarachchi G, et al. miR-29a is a negative regulator of influenza virus infection through targeting of the frizzled 5 receptor. Arch Virol. 2021;166(2):363–73. doi: 10.1007/s00705-020-04877-z
- Monteleone K, Selvaggi C, Cacciotti G, et al. MicroRNA-29 family expression and its relation to antiviral immune response and viro-immunological markers in HIV-1-infected patients. BMC Infect Dis. 2015;15(1):51. doi: 10.1186/s12879-015-0768-4
- Kumar N, Sharma S, Kumar R, et al. Host-directed antiviral therapy. Clin Microbiol Rev. 2020;33(3). doi: 10.1128/CMR.00168-19
- Kumar R, Godara B, Chander Y, et al. Evidence of lumpy skin disease virus infection in camels. Acta Trop. 2023;242:106922. doi: 10.1016/j.actatropica.2023.106922
- Woods JA. Lumpy skin disease—A review. Trop Anim Health Prod. 1988;20(1):11–17. doi: 10.1007/BF02239636
- Haralambieva IH, Kennedy RB, Simon WL, et al. Differential miRNA expression in B cells is associated with inter-individual differences in humoral immune response to measles vaccination. PloS One. 2018;13(1):e0191812. doi: 10.1371/journal.pone.0191812
- Coenen M, Hinze AV, Mengel M, et al. Immune- and miRNA-response to recombinant interferon beta-1a: a biomarker evaluation study to guide the development of novel type I interferon- based therapies. BMC Pharmacol Toxicol. 2015;16(1):25. doi: 10.1186/s40360-015-0025-x
- Kim JK, Kim TS, Basu J, et al. MicroRNA in innate immunity and autophagy during mycobacterial infection. Cell Microbiol. 2017;19(1):e12687. doi: 10.1111/cmi.12687
- Ndzi EN, Nkenfou CN, Mekue LM, et al. MicroRNA hsa-miR-29a-3p is a plasma biomarker for the differential diagnosis and monitoring of tuberculosis. Tuberculosis. 2019;114:69–76. doi: 10.1016/j.tube.2018.12.001
- Ma F, Xu S, Liu X, et al. The microRNA miR-29 controls innate and adaptive immune responses to intracellular bacterial infection by targeting interferon-γ. Nat Immunol. 2011;12(9):861–869. doi: 10.1038/ni.2073
- Steiner DF, Thomas MF, Hu JK, et al. MicroRNA-29 regulates T-box transcription factors and interferon-γ production in helper T cells. Immunity. 2011;35(2):169–181. doi: 10.1016/j.immuni.2011.07.009
- Savan R. Post-transcriptional regulation of interferons and their signaling pathways. J Interferon Cytokine Res. 2014;34(5):318–329. doi: 10.1089/jir.2013.0117
- Fay PC, Wijesiriwardana N, Munyanduki H, et al. The immune response to lumpy skin disease virus in cattle is influenced by inoculation route. Front Immunol. 2022;13:1051008. doi: 10.3389/fimmu.2022.1051008
- HI WN, Sanz-Bernardo B, Graham S, et al. Characterising the cell-mediated immune response to lumpy skin disease virus. Access Microbiol. 2019;1(1A):1. doi: 10.1099/acmi.ac2019.po0289
- KH AM, Moustafa A, Saad MA. Evaluation of humoral and Cell-mediated immunity of lumpy skin disease vaccine prepared from local strainin calves and its related to maternal immunity. J Am Sci. 2016;12:38–45.
- Schmidt ME, Varga SM. The CD8 T cell response to respiratory virus infections. Front Immunol. 2018;9:678. doi: 10.3389/fimmu.2018.00678
- Wherry EJ, Ahmed R. Memory CD8 T-cell differentiation during viral infection. J Virol. 2004;78(11):5535–5545. doi: 10.1128/JVI.78.11.5535-5545.2004
- Doherty PC, Topham DJ, Tripp RA, et al. Effector CD4 + and CD8 + T-cell mechanisms in the control of respiratory virus infections. Immunol Rev. 1997;159(1):105–117. doi: 10.1111/j.1600-065X.1997.tb01010.x
- Goulding J, Abboud G, Tahiliani V, et al. CD8 T cells use IFN-γ to protect against the lethal effects of a respiratory poxvirus infection. J Immunol. 2014;192(11):5415–5425. doi: 10.4049/jimmunol.1400256
- Andersen MH, Schrama D, Thor Straten P, et al. Cytotoxic T cells. J Invest Dermatol. 2006;126(1):32–41. doi: 10.1038/sj.jid.5700001
- Chisari FV. Cytotoxic T cells and viral hepatitis. J Clin Investig. 1997;99(7):1472–1477. doi: 10.1172/JCI119308
- Tokić S, Štefanić M, Glavaš-Obrovac L, et al. miR-29a-3p/T-bet regulatory circuit is altered in T cells of patients with Hashimoto’s thyroiditis. Front Endocrinol (Lausanne). 2018;9:264. doi: 10.3389/fendo.2018.00264
- Wu H, Neilson JR, Kumar P, et al. miRNA profiling of Naïve, effector and memory CD8 T cells. PloS One. 2007;2(10):e1020. doi: 10.1371/journal.pone.0001020
- Stelekati E, Cai Z, Manne S, et al. MicroRNA-29a attenuates CD8 T cell exhaustion and induces memory-like CD8 T cells during chronic infection. Proc Natl Acad Sci, USA. 2022;119(17):e2106083119. doi: 10.1073/pnas.2106083119
- Haegeman A, De Leeuw I, Mostin L, et al. Duration of immunity induced after Vaccination of Cattle with a live attenuated or inactivated lumpy skin disease virus vaccine. Microorganisms. 2023;11(1):11. doi: 10.3390/microorganisms11010210
- Rosca A, Anton G, Botezatu A, et al. miR‐29a associates with viro‐immunological markers of HIV infection in treatment experienced patients. J Med Virol. 2016;88(12):2132–7. doi: 10.1002/jmv.24586
- MacOwan K. Observations on the epizootiology of lumpy skin disease during the first year of its occurrence in Kenya. Bull Epizootic Dis Afr. 1959;7:7–20.
- BM FM, Buchanan DS, Theunissen B, et al. On the history of cattle genetic resources. Diversity. 2014;6(4):705–750. doi: 10.3390/d6040705
- Wakchaure RG, Ahmad P, Kumar P, et al. Development of crossbred cattle in India: a review. Int J Emerging Technol Adv Eng. 2015;5:75.