ABSTRACT
Tilapia Lake Virus (TiLV) is associated with pathological changes in the brain of infected fish, but the mechanisms driving the virus’s neuropathogenesis remain poorly characterized. TiLV establishes a persistent infection in the brain of infected fish even when the virus is no longer detectable in the peripheral organs, rendering therapeutic interventions and disease management challenging. Moreover, the persistence of the virus in the brain may pose a risk for viral reinfection and spread and contribute to ongoing tissue damage and neuroinflammatory processes. In this review, we explore TiLV-associated neurological disease. We discuss the possible mechanism(s) used by TiLV to enter the central nervous system (CNS) and examine TiLV-induced neuroinflammation and brain immune responses. Lastly, we discuss future research questions and knowledge gaps to be addressed to significantly advance this field.
Introduction
Although the central nervous system (CNS) is protected by a complex barrier system, a wide variety of viruses are capable of causing CNS-related diseases in humans and animals. Neurotropic viruses can enter the central nervous system (CNS) by several routes leading to inflammation in distinct anatomical regions such as the meninges (meningitis) and the brain (encephalitis), or simultaneously in multiple regions of the brain (meningoencephalitis) [Citation1]. This may result in irreversible changes leading to the destruction of brain structure and function. Fish are the least evolved animals with a nervous system similar to higher animals, with a well-differentiated brain protected by meninges. As with mammals, neurotropic pathogens affecting the fish CNS represent a significant burden to animal health worldwide. Although several viruses have been documented to target the fish brain, a better understanding of the mechanisms by which these viruses enter the CNS, infect target cells, and induce local immune responses in the brain is still very much needed.
Multiple neurotropic viruses specifically affecting tilapia fish brain have been reported. These include nervous necrosis virus (NNV), tilapia larvae encephalitis virus (TLEV), and tilapia parvovirus (TiPV). NNV is the most studied viral agent causing neuroinfection called viral encephalopathy and retinopathy (VER) occurring in a wide range of fish species, including tilapia (reviewed in [Citation2]). VER is caused by several nervous necrosis viruses (NNVs), small, non-enveloped viruses belonging to the genus Betanodavirus of the family Nodaviridae. TLEV is a herpes-like DNA virus [Citation3, whereas TiPV belongs to the Parvoviridae family [Citation4]. Both TLEV and TiPV lead to mortality rates as high as 90% in affected tilapia populations [Citation3,Citation4]. Other “potentially” CNS penetrant fish viruses have been recently identified within the Flaviviridae, a family of viruses including well-known human neurotropic pathogens such as Zika, dengue, yellow fever, and Japanese encephalitis viruses, all associated with neuroinvasion [Citation5–8].
Tilapinevirus tilapiae (also known as tilapia lake virus or TiLV) has recently emerged as the causative agent of tilapia lake virus disease (TiLVD), which has the greatest potential to decimate tilapia populations. This disease has been associated with rapid global spread of outbreaks, resulting in massive tilapia losses, often with mortality rates as high as 90% [Citation10]. Since its first identification in Israel in 2014 [Citation9], outbreaks of TiLV have been recorded in several continents, and the presence of the virus has been confirmed in numerous countries around the world [Citation10]. Similar to NNV, TLEV and TiPV, TiLV is a neurotropic virus of tilapia (), with disease signs including multifocal haemorrhages with severe blood congestion in the brain [Citation11,Citation12], inflammation of meninges [Citation13], haemorrhages in the leptomeninges [Citation9,Citation14] and brain oedema [Citation9,Citation12,Citation15] .
Table 1. Reported major neurotropic viruses affecting tilapia.
Here, we review the current body of work describing the mechanism(s) of TiLV-associated CNS disease, with inferences drawn from well-studied neurotropic viruses. In addition, we identify important gaps in knowledge regarding TiLV neuroinvasion and neurovirulence and discuss TiLV-induced neuroinflammation and innate and adaptive immune responses in the brain. Neurotropic fish viruses, such as TiLV, pose a serious threat to aquaculture worldwide. Successful antiviral interventions are impossible without a clear understanding of the molecular mechanisms that govern the neuropathogenesis of these viruses.
General anatomy of the teleost fish brain and neurovascular unit
In teleosts, the brain consists of three major regions: the forebrain (or prosencephalon), the midbrain (or mesencephalon), and the hindbrain (also known as the rhombencephalon) [Citation16]. The forebrain is further divided into the telencephalon (which contains the olfactory bulbs), the telencephalic hemispheres, and the diencephalon (), the latter of which contains the pituitary gland, thalamus, hypothalamus, and pineal body. Whilst the telencephalon is associated with learning, appetitive behaviour, and attention, the diencephalon is linked to homoeostasis and appetitive coordination [Citation17,Citation18].
Figure 1. General anatomical comparison of teleost and mammalian brain. The general brain anatomy of teleost fish (left side) showing the different regions and their equivalent in the mammalian human brain (right side).
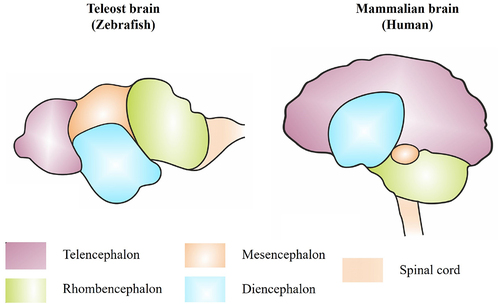
The midbrain includes the peduncles (stalks attaching the cerebrum to the brainstem and containing ascending sensory and descending motor nerve tracts), the tectum opticum (associated with the retina and the visual processes of the brain), and the tegmentum (including the substantia nigra). The hindbrain is divided into the myelencephalon (or medulla oblongata) and the metencephalon, which includes the cerebellum. Coordination of optic nerve inputs occurs in the midbrain tectum opticum, while locomotor activity and balance stimuli are regulated in the hindbrain [Citation17,Citation18]. The primitive meninx (or meninges, the unique meningeal layer of the teleost brain) surrounds the entire brain, while a large portion of motor and sensory cranial nerves reside in the hindbrain.
Two major barriers exist within the brain cavity: the blood-cerebrospinal fluid barrier (BCSFB) and the blood-brain barrier (BBB). The BBB tightly regulates the transport of ions, molecules, and cells between the systemic circulation and the CNS parenchyma, blocking the entry of harmful compounds or cells without restricting the transcerebral movement of essential molecules [Citation16]. In teleost fish (as in humans), the BBB is formed by vascular (or capillary) endothelial cells. These cells are connected by specialized tight junctions and form a functional brain neurovascular unit (NVU) through interactions with the pericytes (interspaced cells present along the walls of capillaries and post-capillary venules of the brain), neurons, radial glial cells (progenitor cells responsible for neurons and nervous system development) and nearby microglia ()[Citation19] .
Figure 2. The neurovascular unit (NVU) of the teleost BBB. The NVU telecosts primarily includes endothelial cells which express tight junction proteins restricting the paracellular flow of fluid to the brain parenchyma. The basement membrane, wh1cl1 helps in maintaining the BBB integrity, consists of extracellular matrix proteins secreted by both endothelial cells and pericytes. Pericytes which also play a role in the formation, integrity and maintenance of the BBB, interact with endothelial cells. Radial glial cells (including those which could potentially be the equivalent of mammalian stellate astrocytes) ensheath the CNS vasculature. Other cells such as microglia and oligodendrocyte precursor cell may also potentially participate in the regulation of the BBB, although their role in the function and regulation of the BBB of telcosts largely remain underappreciated.
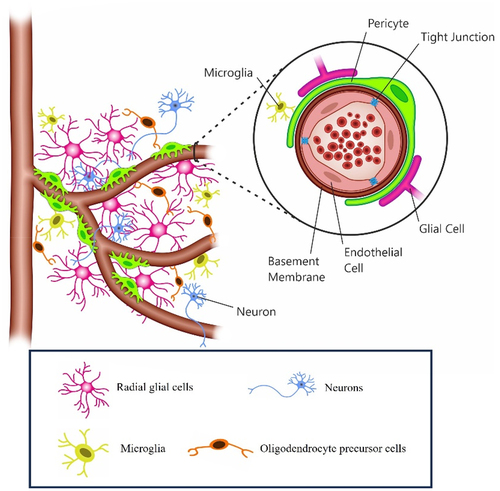
As a model for developmental and disease studies in vertebrates, the teleost zebrafish (Danio rerio), is widely and extensively used thus well annotated, especially for disease model studies. Zebrafish brain endothelial cells have many of the same adaptations as their mammalian counterparts. In addition, they possess homologs for the claudin family of transmembrane tight junction proteins found in mammals that contribute to BBB permeability [Citation20].
Zebrafish have radial glial cells that resemble the astrocytes found in mammals. The BBB is also structurally and functionally similar. Although the NVU of zebrafish lacks a direct equivalent to stellate mammalian astrocytes [Citation21]. A population of cells derived from radial glial cells that are remarkably similar to astrocytes was recently identified in zebrafish and could potentially be considered equivalent to human astrocytes [Citation22].
In both human and zebrafish BBB, pericytes are embedded in the vascular basement membrane close to endothelial cells, where they are thought to play an important role in the formation and maintenance of the BBB [Citation23]. Zebrafish pericytes also express brain pericyte marker genes similar to those found in mammals [Citation24]. However, zebrafish pericytes appear to have both a neural crest and mesoderm origin [Citation25], whereas mammalian pericytes develop only from the neural crest [Citation26].
Microglia are resident immune cells of the brain. They are rapidly activated in response to inflammation, infection or injury of the CNS, where they provide innate immune protection. Microglia play an important role in the clearance of both dead cells in the brain and pathogens invading the brain, in neurogenesis, gliogenesis and neuronal tissue repair [Citation27]. In adult zebrafish, two distinct populations of microglial cells have been described [Citation28]. Phagocytotic microglia constitute the predominant population and are characterized by their amoeboid shape, high mobility, and ccl34b.1 expression [Citation28]. The second subset, with ramified processes, does not express ccl34b.1, has limited phagocytic capacity and restricted mobility, and appears to be regulatory in function [Citation28]. Within the CNS, they patrol neurons and engulf damaged axons whilst secreting key factors involved in neuronal repair [Citation29].
Oligodendrocytes, the myelinating glial cells of the CNS, have been overlooked in teleosts. These cells generate and maintain myelin and participate in axonal signalling and the maintenance of the unsheathed axons. Their role in regulating the BBB, particularly in teleosts, needs to be more appreciated. In both teleosts and mammals, oligodendrocytes originate from a specific progenitor motor neuron (pMN) domain in the ventral spinal cord [Citation16]. These pMN domain oligodendrocyte progenitors form oligodendrocyte precursor cells (OPCs), which further differentiate into oligodendrocytes following their migration towards axonal tracts [Citation16].
Tilapia lake virus
TiLV is currently the only species in the genus Tilapinevirus of the Amnoonviridae family [Citation30]. TiLV is an enveloped virus with a linear, negative-sense single-stranded RNA genome of approximately 10.3 kb in total length [Citation31]. TiLV virions are round to oval with a diameter ranging from 55 nm to 100 nm () [Citation9,Citation11][,,Citation32,Citation33]. The genome of TiLV consists of 10 RNA segments (), each encoding at least one open reading frame (ORF) [Citation31] []. The first and largest segment shows homology with the influenza C virus polymerase basic (PB)-1 subunit, whilst the protein derived from segment 4 is the virus nucleoprotein (NP) [Citation31][,Citation34]. The functions of the proteins derived from the ORFs of the remaining eight segments remain to be determined as the predicted ORF deriving from these segments show no significant homology with other known viral sequences despite the similar genomic organization of TiLV to orthomyxoviruses [Citation31] [].
Figure 3. (a) ultrastructure of TiLV obtained by EM-analyses (from fyngor et al. [Citation9] with permission). (b) The 10-genome segments of TiLV virus represented, from the longest segment (segment 1), presenting homologies to the PBI sub-unit of influenza viruses, to the smallest (segment 10) of a yet unknown function. Scale bar, 100 nm.
![Figure 3. (a) ultrastructure of TiLV obtained by EM-analyses (from fyngor et al. [Citation9] with permission). (b) The 10-genome segments of TiLV virus represented, from the longest segment (segment 1), presenting homologies to the PBI sub-unit of influenza viruses, to the smallest (segment 10) of a yet unknown function. Scale bar, 100 nm.](/cms/asset/7f50c768-290b-408b-a670-c9c65ebd7859/kvir_a_2329568_f0003_oc.jpg)
TiLV seems to infect primarily tilapia species (particularly Nile tilapia Oreochromis niloticus, Mozambique tilapia O. mossambicus, grey tilapia (Oreochromis niloticus x O. aureus) and red tilapia (Oreochromis spp.)). Other fish species, such as ornamental African Cichlids (Aulonocara spp.) [Citation35], tinfoil barbs (Barbonymus schwanenfeldii) [Citation36,Citation37], giant gourami Osphronemus goramy [Citation38], angelfish (Pterophyllum scalare), and firemouth cichlid (Thorichthys meeki) [Citation39] have also shown susceptibility to TiLV. In vivo infection has been experimentally recapitulated by intraperitoneal (IP) injection in adult zebrafish [Citation15],Citation40], zebrafish larvae [Citation41], juvenile rainbow trout (Oncorhynchus mykiss) and brown trout (Salmo trutta) [Citation42].
As previously mentioned, TiLVD results in mortalities between 20 and 90% [Citation10], although asymptomatic cases have also been reported [Citation43]. All the life stages of tilapia, including fertilized eggs, yolk-sac larvae, fry, fingerlings, and adults, appear to be susceptible to TiLV infection [Citation32,Citation44–46]. Several gross pathological signs of the disease have been reported, including ocular alterations and lesions, and skin erosions in both wild and farmed tilapia [Citation9], haemorrhagic patches, detached scales, open wounds, and skin discoloration [Citation47], abdominal distension, protrusion of the scales and gill pallor [Citation32]. Systemic infection leads to broad tissue tropism, including the liver, brain, kidney, gills, fins, spleen, intestines, heart, ovaries, testis, connective muscle, and optic tissue [Citation9],Citation40,Citation44,Citation48] of both infected tilapia and zebrafish, as well as circulating leukocytes [Citation48].
Viral particles have been detected in the brain of diseased tilapia and zebrafish [Citation11,Citation15], and infectious virions could be isolated from brain tissues of diseased tilapia [Citation11,Citation13]Citation49]. Moreover, several pathological signs in the brain have been reported, including multifocal haemorrhages with severe blood congestion in the brain [Citation12],Citation11,Citation46], inflammation of the meninges [Citation13], haemorrhages in the leptomeninges [Citation9,Citation14] , and brain oedema [Citation9,Citation12,Citation15] . Furthermore, using the zebrafish-TiLV infection model, it has been demonstrated that TiLV induces neuroinflammation, microglia activation, and behavioural changes in infected fish. Interestingly, TiLV persisted in the brain of infected zebrafish for at least 90 days even when the virus was not detectable in other peripheral organs [Citation15].
TiLV transmission and CNS-invasion
Viral transmission, possible routes of infection and dissemination
Both vertical and horizontal transmission of the virus have been documented during TiLV infection [Citation9],Citation50,Citation51]. This includes interspecies horizontal transmission from red hybrid tilapia to naïve giant gourami [Citation38], suggesting that horizontal waterborne transmission and faecal-oral infection [Citation52] represent the most important transmission routes. As such, the mucosal surfaces of the gills, buccal, and digestive cavity are the most likely ports of virus entry into the host [Citation53–55]. TiLV genomic RNA was strongly detected in the olfactory bulb of infected tilapia [Citation13]. As the nasal cavity is in direct contact with water, where the virus can be shed through faeces and mucus, the olfactory mucosa of the nasal cavity also represents a further potential conduit for virus dissemination to the brain. It is also possible that the virus gains access via epithelial cells covering the skin and/or the fins, which have all been reported as portals of entry for neurotropic betanodaviruses [Citation2],Citation56]. Whether TiLV enters at a specific entry site or multiple entry portals remains unknown.
Unlike exclusively neurotropic betanodaviruses, which have restricted replication in nervous tissue, typically the brain and retina [Citation2]Citation57,Citation58], TiLV can infect multiple organs of the host, implying that TiLV induces a systemic infection [Citation48,Citation59]. The entry receptor(s) for TiLV remain unknown. However, the virus enters TmB cells derived from tilapia heart via a dynamin-mediated endocytic pathway, which does not require endosomal acidification for endosomal escape but depends on cholesterol-rich lipid rafts [Citation60]. Shortly after entry, the genome of the virus can be detected in the nucleus [Citation31], the site of viral replication and transcription.
The blood (haematogenous spread) and peripheral nerves (neural spread) are generally regarded as the primary means of dissemination. During haematogenous spread, newly synthesized viral particles, produced at entry sites and released into the extracellular fluids, are taken up by the local lymphatic system, draining into the bloodstream (viraemia), thereby delivering virions to target organs. The systemic nature of TiLV infection [Citation59] and the detection of TiLV antigens in circulating leukocytes [Citation48] suggest that haematogenous spread is the main mechanism for disseminating the virus.
The possible involvement of the neural conduit in TiLV dissemination is supported by some reports of immunohistochemical detection of TiLV proteins in neuronal cells of naturally infected tilapia [Citation48]. Neural spread may be plausible if we consider that for some neurotropic viruses, viral replication occurs first in non-neuronal cells (often epithelial and/or endothelial cells) near the site(s) of infection, and virus spreads into afferent (sensory) or efferent (motor) nerve fibres that innervate the infected peripheral tissues [Citation61].
Disease pathology in neurons is a hallmark of active viral replication and disease during infections with neurotropic betanodaviruses like NNV. However, reports of TiLV pathology in neurons are rare, although neuronal degeneration within the optic lobes of the telencephalon has been reported [Citation9]. Spinal curving is another CNS-related pathological sign reported during TiLV infection in zebrafish larvae [Citation18], suggesting a focal activation of proinflammatory signals within the spinal cord [Citation62]. However, the absence of pathology in the neurons does not necessarily imply a lack of neuronal infection as it has been reported that neurons infected with some pathogenic strains of rabies virus can maintain normal size and biological functions [Citation63].
CNS invasion
Several pieces of evidence attest to the neurotropic nature of the TiLV. These include replication in primary brain cell cultures [Citation9] and in brain-derived cell lines [Citation49], visualization of virus particles in the brain [Citation11,Citation15], isolation of infectious virions from infected brain tissues [Citation9,Citation11]Citation49,Citation64], detection of viral genomic RNA in the brain [Citation9,Citation11,Citation13]Citation30,Citation38,Citation49,Citation65,Citation66], repeated demonstration of brain pathology [Citation9,Citation13,Citation15] and an increase over time of viral RNA in the brain [Citation66] denoting either an ongoing local viral replication or an ongoing CNS invasion. However, the mechanism(s) through which TiLV gains access to the brain during infection are yet to be elucidated. Neurotropic viruses can reach the CNS after dissemination from peripheral organs through mechanisms that include: (1) infection of brain microvascular endothelium; (2) infection of leukocytes; (3) axonal transport from peripheral neurons (4) free pathogen transcytosis through the BBB; (5) paracellular entry at the BBB.
Infection of brain microvascular endothelial cells
Viral particles that reach the CNS after dissemination from entry sites in peripheral organs can enter the brain through infection of brain microvascular endothelial cells (BMVECs), a central element of the microvasculature that forms the BBB ().
Under normal conditions, the endothelial cells lining the microvasculature within the CNS are connected by tight and adherent junctions that receive support from pericytes and astrocytes. Upon infection of BMVECs, neurotropic viruses can disrupt the BBB by altering tight junction expression and functionality and inducing inflammatory mediators’ expression by other cell types. This is often accompanied by the uncontrolled migration of immune cells into the brain parenchyma and the induction of neuronal inflammation thereafter [Citation67].
No direct in vitro and/or in vivo evidence of active TiLV infection and replication within BMVECs has been established. However, the detection of TiLV antigens within endothelial cells lining the blood vessels of the brain [Citation13],Citation48] supports the possibility that TiLV may infect BMVECs, as is the case for West Nile virus (WNV) [Citation68] and Epstein-Barr virus [Citation69]. Interestingly, using the zebrafish model, it has also been demonstrated that the human neurotropic chikungunya virus infects endothelial cells of the brain vasculature [Citation70].
Disruption of the BBB is a hallmark of WNV neuropathogenesis and often results in the uncontrolled entry of immune cells into the brain [Citation71]. Mechanistically, WNV stimulates the loss of tight junction proteins in both epithelial and endothelial cells [Citation72], leading to the release of proteinases that degrade the basement membrane. As a result, leukocytes migrate from the capillaries into the surrounding tissue where, upon recognition of WNV double-stranded RNA via toll-like receptor 3 (TLR3), they release pro-inflammatory cytokines [Citation68,Citation73], which in turn leads to inflammation and subsequent encephalitis.
Several studies have also reported perivascular cuffing of immune cells in the brain during TiLV infection, suggesting that similar to WNV, TiLV could induce disease in brain microvascular endothelium [Citation9,Citation11,Citation12]Citation65] as well as the leukocyte migration into the brain parenchyma.
Infection of leukocytes via “Trojan horse” strategy
Some neurotropic viruses infect circulating leukocytes to gain access to the brain, where these cells routinely act as immune sentinels. This is known as the “Trojan Horse” entry, whereby viruses hide within immune cells and are delivered undetected to the brain parenchyma. HIV infects the CNS through this strategy, whilst the human polyomavirus JC virus can infect B-cells and infiltrate the CNS of immunosuppressed patients, resulting in the infection of oligodendrocytes and astrocytes [Citation74]. There is currently no direct evidence that TiLV uses this strategy, although the ability of TiLV to infect circulating leukocytes has recently been confirmed [Citation48].
Infection of peripheral neurons and axonal transport
Sensory or motor neurons extend outside of the CNS and are connected by neurochemical synapses. During infection of peripheral neurons, neurotropic viruses can use anterograde transport and/or retrograde axonal transport to reach the CNS. Rabies virus uses dynein-motors to move via the retrograde route [Citation75–77], and WNV uses both retrograde and anterograde axonal transport [Citation78]. Penetration to the CNS can also occur following infection of olfactory nerves. Several viruses use this strategy, including influenza A virus (IAV), WNV, vesicular stomatitis, and Nipah [Citation79].
Multiple routes of CNS penetration have been proposed for neurotropic betanodaviruses (e.g. NNV) of fish. Necrosis and vacuolation of nerve cells are first observed in the spinal cord [Citation80], and subsequent spread to the CNS is thought to occur through axonal transport to the brain stem via cranial nerves, including the vagus nerve [Citation81]. Betanodavirus infection can also occur through the nasal cavity, where the virus penetrates the nasal epithelium and disseminates through the olfactory nerve and olfactory bulb to the brain tissue [Citation57,Citation81]. Moreover, studies on zebrafish larvae revealed that Sindbis virus (a human neurotropic virus) uses axonal transport, both from the periphery to the CNS and between neural tissues [Citation66].
The dorsal ends of fish gills possess an array of nerve trunks that connect the gills to the CNS. These nerve trunks form branches of cranial nerves VII (the facial), the glossopharyngeal IX nerve, and the vagus X nerve [Citation82]. A study of the kinetics of viral loads in various organs of tilapia during TiLV infection demonstrated that following either IP injection or cohabitation infections, the gills had significantly higher virus loads than the brain or liver [Citation66]. High levels of TiLV antigen have also been detected in the gill epithelia, basal cells, and primary laminae [Citation48]. Therefore, TiLV may invade the brain via cranial, vagus, and/or olfactory nerves, with the gills representing the entry point. Further support for this notion comes from reports of immunohistochemical detection of TiLV proteins in neuronal cells of naturally infected tilapia [Citation48], thus making neural spread a plausible route of CNS penetration during TiLV infection.
Transcytosis through the blood – brain barrier
Virus transcytosis (or transcellular invasion) across the BBB involves the unidirectional transport of free viruses from the apical to the basolateral membrane of the BBB. During this process, viruses can be trafficked across the BBB by endocytosis on the apical side and exocytosis on the basolateral side [Citation83] (). The endocytic phase involves either receptor-mediated internalization or adsorptive internalization [Citation84]. Initially thought to be attenuated in brain endothelial cells, receptor-mediated transcytosis occurs in almost all endothelial cells, including those of the brain [Citation85,Citation86]. Several receptors present in the BBB, including the insulin receptor, which shows high amino acid identity between teleosts (particularly rainbow trout) and their human homologs [Citation87], are capable of inducing receptor-mediated transcytosis [Citation88]. Transcytosis can occur through clathrin-coated pits during receptor-mediated transcytosis, caveolae during adsorptive-mediated endocytosis, or macropinocytosis involving macropinocytotic vesicles [Citation88]. The BBB expresses low clathrin levels, and caveolae-mediated transcytosis is limited [Citation89]. Macropinocytosis can be induced in the BBB in response to various growth factors [Citation90]. Fibroblasts, neurons, and microglia are also capable of macropinocytosis [Citation90].
Figure 4. Paracellular and transcytosis virus invasion routes across the BBB. At the BBB (a), viruses can gain access to the brain parenchyma (b) or the CNS either by passive diffusion following the disruption of tight junction integrity by proteases (paracellular) for instance, or by transcytosis, a process during which the virus is endocytosed by endothelial cells to be subsequently released in the brain parenchyma by exocytosis. It is important to note that most evidence of these two routes of brain invasion mainly come from in vitro stud ies. Adapted from Marshall et al [Citation84].
![Figure 4. Paracellular and transcytosis virus invasion routes across the BBB. At the BBB (a), viruses can gain access to the brain parenchyma (b) or the CNS either by passive diffusion following the disruption of tight junction integrity by proteases (paracellular) for instance, or by transcytosis, a process during which the virus is endocytosed by endothelial cells to be subsequently released in the brain parenchyma by exocytosis. It is important to note that most evidence of these two routes of brain invasion mainly come from in vitro stud ies. Adapted from Marshall et al [Citation84].](/cms/asset/bdebc0c5-d59a-49c4-bc0c-6f989e8f63a6/kvir_a_2329568_f0004_oc.jpg)
Replication-deficient WNV can transmigrate from the apical to the basolateral side of polarized human BMVECs via endocytosis mediated by lipid rafts [Citation91]. The basolateral release of Zika virus from brain endothelial cells is sensitive to nystatin, an inhibitor of caveolae-mediated transcytosis [Citation92]. HIV-1 enters primary BMVECs via macropinocytosis, requiring cholesterol and MAPK signalling [Citation92].
To date, TiLV transcytosis through the BBB has not been reported. However, TiLV entry into endothelial TmB cells is sensitive to latrunculin A (actin inhibitor), nocodazole (microtubule polymerization inhibitor), and methyl-β-cyclodextrin (cholesterol-dependent endocytosis) [Citation60]. This suggests that TiLV might cross the BBB in a process dependent on actin and microtubule polymerization, both known to be involved in the formation of macropinosome ruffles. Moreover, similar to HIV-1, ERK/MAPK signalling participates in TiLV propagation [Citation93].
Paracellular entry at the blood-brain barrier
Under normal conditions, tight junctions restrict the passive diffusion or paracellular entry of molecules from the blood into the CNS through the intercellular space between endothelial cells. However, some neurotropic viruses have evolved strategies to disrupt and increase the permeability of the BBB, thereby facilitating their entry into the CNS. These strategies include the secretion of tight junction-disrupting proteases () and the stimulation of inflammatory responses and endothelial cell death.
WNV is associated with enhanced BBB permeability through the increased production of matrix metalloproteinases capable of degrading the basement membrane. Such metalloproteinases disrupt BBB integrity by cleaving tight junction proteins and the adjacent extracellular matrix [Citation94,Citation95]. Viral proteins such as the non-structural protein 1 (NS1) of neurotropic flaviviruses can also promote the degradation of endothelial glycocalyx components by inducing the expression of cathepsin L and heparinase, resulting in increased BBB permeability. Likewise, HIV proteins such as tat, gp120, and nef can affect BBB changes [Citation96].
Features associated with BBB disruption, such as neuroinflammation [Citation15], neuronal necrosis [Citation9] ,Citation50], and gliosis [Citation9,Citation11,Citation12]Citation52], have been reported during pathological analyses of the brains of TiLV-infected fish. In mammals, immune-modulatory proteins such as interferon (IFN) -gamma-inducible protein 10 (IP-10 or CXCL10) can enhance the expression of tumour necrosis factor (TNF)-α in a JNK (c-Jun N-terminal kinase)-dependent manner, leading to BBB disruption [Citation97]. Inflammasome activation also triggers pyroptosis, a programmed cell death that increases BBB disruption [Citation98–100] and gliosis [Citation100]. The disruption of tight junction complexes is also associated with the enhanced generation of reactive oxygen species (ROS), as virus infection can induce mitochondrial damage or NADPH oxidase activation, resulting in ROS generation [Citation101]. As brain endothelial cells are highly susceptible to oxidative stress, releasing various cytokines and proteases due to ROS generation can damage BBB vasculature. This can be further exacerbated by their exposure to viral proteins [Citation96]. Interestingly, a recent study revealed that TiLV can cause structural and functional damage to mitochondria by reducing mitochondrial mass, ATP levels, and mitochondrial membrane potential [Citation102]. However, additional research is warranted to determine the role of ROS and NADPH oxidase activation during TiLV infection.
Some neurotropic viruses can also induce syncytium formation, resulting in extensive vascular damage associated with the influx of inflammatory cells [Citation103,Citation104] and haemorrhage [Citation105], as observed for Nipah and Hendra viruses. Interestingly, syncytial formation has been observed in the brain tissue of tilapia experimentally infected with TiLV [Citation64,Citation106,Citation107]. Moreover, inflammatory cell infiltration [Citation9,Citation11,Citation12]Citation52,Citation107,Citation108] and brain haemorrhage [Citation9,Citation11,Citation12,Citation15] are amongst the pathological signs associated with TiLV disease in the brain.
Neuroinvasion of the blood-cerebrospinal fluid barrier and meninges
The teleost fish brain comprises two lateral ventricular spaces: the diencephalon medial ventricular space and the hindbrain medial ventricular space [Citation109]. Cerebrospinal fluid (CSF) produced by the epithelial cells of the choroid plexus (CP) flows through this ventricular system [Citation109]. The diencephalic (dCP) and the myelencephalic (mCP) choroid plexus exist in the teleost brain [Citation110]. Unlike the mammalian brain, the CSF space in teleost fish lacks a subarachnoid space [Citation111]. The blood-cerebrospinal fluid barrier (BCSFB) is characterized by fenestrations that interrupt the endothelial lining of CP capillaries, and like the endothelial cells of the BBB, the CP epithelium is connected via tight junctions. These unique apical tight junctions of the BCSFB () prevent the paracellular diffusion of water-soluble molecules [Citation110]. The numerous transport systems expressed by epithelial cells of the CP allow direct transport of ions and nutrients into the CSF whilst removing toxic agents [Citation112].
Figure 5. Paracellular and transcytosis virus invasion routes across the BCSFB. Viruses can cross the meningeal blood cerebrospinal fluid barrier (BCSFD) (a) by a paracellular mechanism (b) in which virus-infected leukocytes attach lo the endothelium, transverse the fenestrated endothelial cells, and cross the choroid plexus epithelial cells into the CSF following the disruption of tight junction integrity (paracellular). Alternatively, virus-infected leukocytes or cell-free viruses present within blood vessels of the CP, transverse the endothelium and infect epithelial cells followed by an apical release of the virus across the CP epithelium into the CSF by transcytosis. Most evidence of these two routes of brain invasion mainly come from in-vitro studies, although experimental infection in rodents have shown that mumps vims can enter the CNS via the CSF through the CP. Adapted from Marshall et al. [84).
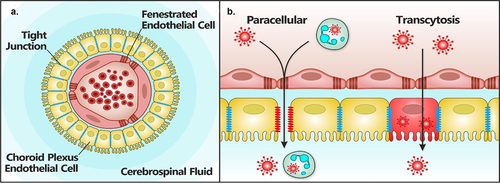
As in the mammalian brain, the primitive meninx (meninges) of teleosts contains immune cells [Citation113]. The CP connects these lymphatics and provides a potential mechanism for leukocyte egress from the CNS. Studies of haematogenous invasion via the CSF across the CP are lacking for viruses. Similarly, the transcellular passage of the BCSFB represents a poorly characterized route of viral neuroinvasion.
Crossing of the meningeal blood – CSF barrier by viruses involves virus-infected leukocytes in meningeal blood vessels attaching to the endothelium, transversing the fenestrated endothelial cells, and crossing the CP epithelial cells into the CSF. This can be facilitated by the disruption of tight junction integrity. Direct infection of endothelial cells may subsequently lead to the virus spreading into the CSF. Alternatively, for viruses to breach the BCSFB, virus-infected leukocytes or cell-free virus present within the blood vessels of the CP transverse the endothelium and infect the epithelial cells, followed by the apical release of virus or movement of virus-infected leukocytes across the CP epithelium into the CSF [Citation114] ().
In vitro studies of Zika virus have revealed its ability to cross human choroid plexus papilloma cells and human brain vascular pericytes without disrupting tight junctions or through enhanced barrier permeability, suggestive of a transcellular mode of invasion [Citation115]. These findings were supported by in vivo studies in which Zika virus was found to infect CP pericytes of Ifnar-/- mice, leading to the detection of the virus in the CSF prior to infection of the brain parenchyma [Citation115]. Experimental infection in rodents suggests that mumps virus can enter the CNS via the CSF through the CP and, once in the CSF, can spread through the ventricular system with virus replication in ependymal cells lining the ventricles. This is then followed by the invasion of the brain parenchyma [Citation116].
SARS-CoV-2 infection of CP epithelial cells and subsequent CNS invasion via the BCSFB have also been suggested [Citation117,Citation118]. In human-pluripotent-stem-cell-derived brain organoids, SARS-CoV-2 infection results in damage to the CP epithelium, leading to leakage and proinflammatory changes across the BCSFB [Citation118], potentiating CNS invasion and disease pathogenesis. Matrix metalloproteases have been implicated in damages to the BCSFB during infectious meningitis [Citation119] and systemic inflammatory disease [Citation120]. Viral proteins have also been detected in the CP of WNV-infected mice [Citation121].
Bacharach et al. [Citation31] reported the detection of TiLV genomic RNA (segments 1 and 5) at the leptomeninges and adjacent to blood vessels. Later studies by Dinh-Hung et al. [Citation13] detected TiLV genomic RNA (segments 1 and 3) in the primitive meninges and endothelial cells of blood vessels close to the CSF. The intensity of detection in the periventricular regions indicated a distinct gradient of infection, most intense in the ventricle, ependymal cells lining the ventricles, and in the CP of epithelial cells [Citation13]. Notably, TiLV can infect endothelial cells, choroidal epithelial cells, and ependymal cells of the BCSFB, highlighting the ventricles and CSF as important conduits of TiLV neuroinvasion. Whether CSF circulation contributes to pathogen dissemination within the CNS is unknown, although TiLV infection is also associated with pathological signs of meningitis, including the infiltration of lymphocytes in the meninges (lymphocytic meningitis [Citation14]), cerebral and meningeal haemorrhage, inflammation and haemorrhage in the leptomeninges [Citation12], infiltration of eosinophilic cells in meningeal areas of the brain [Citation66], inflammation of meninges [Citation13], and meningoencephalitis [Citation46], altogether supporting this route of invasion.
Kinetics of TiLV infection in the brain
The route of virus entry into the host influences its mode of dissemination. A paucity of studies have examined the kinetics of TiLV viral loads in the brain. When IP injection was used to model TiLV disease in tilapia or zebrafish, peripheral organs such as the liver, kidney, and spleen showed the highest viral loads during the early stages of the infection (1–6 days post-infection, dpi) [Citation15],Citation65]. In the brain viral load was at comparable levels between 6 and 7 dpi, and became significantly higher than in the peripheral organs by 12–14 dpi [Citation15],Citation65]. Interestingly, whilst viral loads in peripheral organs declined later in the infection (12–14 dpi in tilapia and 14–45 dpi in zebrafish), viral loads in the brain persisted at quite a high level, at least until 34 dpi (in Nile tilapia) [Citation65] and 90 dpi (in zebrafish) [Citation15].
During infection through cohabitation, which is more reflective of a natural infection, viral loads in the gills were higher than those in the liver at early time points (3 dpi), but virus was first detected in the brain only at 6 dpi, where viral load continuously increased up to 14 dpi in susceptible tilapia fish strains [Citation66].
The delay in the establishment of the infection within the brain of fish in both infection models may be indicative of the CNS as a secondary replication site for TiLV. In a previous study, changes in the brain of TiLV-infected fish were not observed prior to 30 dpi (in O. niloticus for instance), although viral loads in the brain peaked at 17 dpi and were significantly higher than in other organs [Citation65]. Differences related to fish strains and species have been reported with some strains of O. niloticus being for instance largely resistant to TiLV infection [Citation66]. Pathological changes in the CNS also seem to vary between adult and larvae stages [Citation15],Citation41].
Neurotropism
Once in the brain, TiLV spreads to the forebrain, midbrain, and hindbrain, with similar viral loads reported in all three regions both in tilapia and zebrafish [Citation13,Citation15] Citation66]. As the entry receptor for TiLV has not been identified, its expression and distribution cannot be used as a proxy for neuronal permissiveness.
Most studies have focused on detecting TiLV antigen and genomic RNA, primarily relying on hybridization techniques. There is a lack of direct evidence of viral infection and replication (tropism) within specific brain cells. However, in tilapia, hybridization signals are more intense in the olfactory bulbs, the hemispheres of the telencephalon, and the periventricular zone of the hypothalamus (in the diencephalon) [Citation13]. In the midbrain, virus detection was observed in the periventricular grey zone of the optic tectum and the torus longitudinalis. In the hindbrain, TiLV was densely localized in the medulla oblongata, more specifically, the vagal lobe [Citation13].
Current evidence suggests that specific neuronal cell types are differentially susceptible to TiLV infection. In tilapia, viral antigens have been detected in neurons and vascular endothelial cells of the brain and brain neuronal cells [Citation48]. Moreover, in a recent study, a newly established astrocyte-like cell line deriving from the brain of tilapia (O. niloticus) and used as an in vitro model for studies of the fish BBB was susceptible to TiLV infection [Citation122], suggesting that tilapia astrocyte-like cells may also be susceptible to TiLV infection. In situ hybridization has revealed the presence of TiLV genomic RNA in the primitive meninges, in the epithelium of the blood vessels, in ependymal cells lining the ventricles, and in CP epithelial cells [Citation13]. However, there is currently limited evidence that pericytes, microglia, and oligodendrocytes are susceptible to TiLV infection.
Overall, it is possible that within the brain, TiLV neurotropism includes neurons such as the motor neurons contained within the vagal lobe, neural structures such as the torus longitudinalis, the hypothalamus, vascular endothelial cells, ependymal cells, choroidal epithelial cells, and astrocytes (). Direct in vitro and in vivo evidence of viral replication within these cells and the susceptibility of other cell types, such as microglia, pericytes, and oligodendrocytes, still needs to be established.
Figure 6. Possible neurotropism of TiLV. Virus antigen or viral RNA (indicated by the red virus next to each cell type) has been detected in the different cell types here depicted. These are the neurons, the ependymal cells, the choroidal epithelial cells and endothelial cells. In addition, astrocyte-like cells deriving from the brain of tilapia and used in an organoid model of the tilapia BBB [Citation123] were also found to be susceptible to TiLV infection, suggesting that these cells may as well be infected by the virus.
![Figure 6. Possible neurotropism of TiLV. Virus antigen or viral RNA (indicated by the red virus next to each cell type) has been detected in the different cell types here depicted. These are the neurons, the ependymal cells, the choroidal epithelial cells and endothelial cells. In addition, astrocyte-like cells deriving from the brain of tilapia and used in an organoid model of the tilapia BBB [Citation123] were also found to be susceptible to TiLV infection, suggesting that these cells may as well be infected by the virus.](/cms/asset/d916234b-ac4c-4a67-bb92-f38c63fa4c52/kvir_a_2329568_f0006_oc.jpg)
Neurovirulence and CNS-associated clinical manifestations
The ability of a virus to cause CNS pathology independently from its ability to infect cells of the CNS is termed neurovirulence [Citation123]. Evidence that TiLV infection induces CNS inflammation exists. During TiLV infection, up-regulation of the expression of genes encoding activation markers of microglia/macrophages (csf1r and cd68) was observed in the brain of adult zebrafish, while up-regulation of the expression of apoeb, another microglia/macrophages activation marker, was demonstrated in the brain of zebrafish larvae [Citation15]. Moreover, TiLV infection of transgenic Tg(mpeg1.1:mCherryF)ump2 zebrafish larvae results in changes in microglia shape, from a resting ramified state in mock-infected to a highly ameboid active state in TiLV-infected larvae at 48 hpi [Citation15]. Regarding astrocytes, TiLV infection resulted in up-regulation of the expression of astrocytes marker (gfap) in the brain of zebrafish larvae but not in the brain of adult zebrafish [Citation15].
In tilapia, multifocal areas of gliosis have been reported during TiLV infection [Citation9,Citation11,Citation12][Citation52]. Perivascular infiltrations of lymphocytic cells were reported in the brain of both experimentally and naturally infected tilapia [Citation9,Citation11,Citation12][Citation65,Citation108], in addition to the presence of macrophage-like cells in basal regions of the brain [Citation65]. In some cases, infiltrating inflammatory cells were localized to white matter [Citation65].
Neuronal degeneration has been observed in the telencephalon (particularly in the optic lobes) of experimentally infected tilapia [Citation9]. Localized blood clotting, suggestive of blood vessel rupture, has been reported in the brain cortex [Citation108]. Multifocal cerebral, meningeal and leptomeningeal haemorrhages [Citation9,Citation11,Citation13,Citation14] and severe capillary congestion [Citation9,Citation12–14],Citation108] have also been observed, which are all suggestive of neurovascular injury and lesions in the CNS vasculature. Taken together, these pieces of evidence suggest that TiLV could exert its neurovirulence through acute haemorrhagic brain injury, in which neuronal lesions evolved to develop cerebral oedema, a pathological signature of TiLV infection [Citation9,Citation12,Citation15]Citation124].
Focal inflammation in the leptomeninges and meninges has also been described [Citation12,Citation13], in addition to the presence of an increased number of eosinophilic cells, mainly in meningeal areas (lymphocytic meningitis) of the brain of TiLV-infected tilapia [Citation66]. Interestingly, upregulation of apoptotic genes was not observed in the brains of three different strains of tilapia during TiLV infection [Citation66], although degeneration and signs of necrosis were reported in zebrafish larvae [Citation41]. This suggests that TiLV may not induce apoptosis like some pathogenic strains of rabies virus [Citation63]. In zebrafish, loosely packed neuropil and disintegration of the perikaryal have been described [Citation15],Citation41]. Vacuole formation in basal regions of the brain with pyknotic nuclei has also been reported in tilapia [Citation64,Citation65,Citation106,Citation107] in addition to TiLV-induced meningoencephalitis [Citation11,Citation14]Citation46].
The underlying neurovirulent pathogenesis of TiLV is therefore diverse and unlikely to be mediated by a single mechanism. Moreover, it is also likely that the mode of infection (IP injection, cohabitation, intracoelomic or intragastric inoculation), virus strain, host factors, developmental stage of the fish, species, or previous underlying disease (e.g. co-infections) can complicate TiLV-associated CNS pathology. This is supported by reports of no pathology and the absence of lesions in the brain in some TiLV infection models [Citation47,Citation108,Citation125].
The resulting clinical manifestations possibly associated with CNS impairment include lethargy [Citation9,Citation12,Citation14][Citation52,Citation108], loss of appetite [Citation12],Citation40], loss of balance [Citation11,Citation13,Citation65], and abnormal swimming behaviour [Citation14],Citation52]. In zebrafish, TiLV infection has also been shown to induce a sickness behaviour characterized by a decreased locomotor activity (significant reduction in the speed and distance swum). Moreover, TiLV-infected fish tend to spend less time moving and more time at the bottom part of the tank when compared with mock-infected fish [Citation15]. TiLV-infected zebrafish also show irregular swimming patterns [Citation40] and unusual spiral movements during swimming [Citation15].
Neuroinflammation, innate and adaptive immune responses in the brain
Neuropathogenesis is often associated with immune mediators produced in the CNS during antiviral responses. Several aspects of the brain immune response in teleosts remain unknown, as do the neuroimmune interactions that occur during TiLV infection. Recent studies have begun to address immunity to TiLV infection (reviewed in [Citation126]). Despite the prolonged persistence of the virus in the brain [Citation15],Citation65], local immune responses from both the innate and adaptive systems act in concert to attempt to clear the infection.
Innate immune response interface
TiLV infection induces upregulation of the expression of genes encoding proteins involved in type I IFN pathway such as the pattern recognition receptors (PRR): RIG-I and TLR3, transcription factors: IRF3 and IRF7, type I IFN, and the antiviral protein Mxa in the brain of zebrafish [Citation15]. Similarly, in the brain of Nile tilapia, TiLV induces upregulation of TLR3, TLR7, IPS-1, type I IFN and Mx [Citation65,Citation66,Citation108]. Moreover, TiLV-induced upregulation of genes encoding proinflammatory cytokines (IL-1β, IFNγ1–2, TNF, IL-8 (CXCL8A)), enzyme COX2b, and anti-inflammatory cytokine IL-10 was demonstrated in the brain of zebrafish [Citation15] while up-regulation of genes encoding IL-1β, and TNF-a was observed in the brain of tilapia [Citation66,Citation127], although no upregulation of apoptotic genes was observed [Citation66].
IL-1β and IL-8 are potent inflammatory cytokines [Citation128,Citation129] that disrupt BBB integrity. Both are upregulated during rabies virus infection [Citation130], facilitating both CNS invasion and leukocyte trafficking into the CNS [Citation131,Citation132]. Although essential for virus clearance, the latter can lead to increased brain inflammation and microglia activation.
Similarly, microglia are activated in the brains of adult zebrafish infected with TiLV [Citation15]. These cells represent the first line of innate immunity in the CNS and play an important role in controlling viral replication [Citation133].
Astrocytes/radial glial cells were activated only in zebrafish larvae (but not in adults) during TiLV infection [Citation15]. These cells perform various neuroprotective functions and are activated by proinflammatory cytokines, such as IL-1β, TNF, and IFNγ, which promote astrogliosis during viral infection [Citation134,Citation135]. An increased number of eosinophilic cells in meningeal areas of the infected brain of tilapia have been observed during TiLV infection [Citation66]. Eosinophils are a key arm of the innate immune response, but their role during viral infection is still unclear as these cells are mainly associated with protection against helminthic parasitic infections as well as with airway hyper-responsiveness to infectious disease. Most studies investigating the role of eosinophils in viral infections have focused on viral infections of the airway, as the recruitment of eosinophils to the airways is a prominent feature of the asthmatic inflammatory response. As such, studies on the role of eosinophils in viral infections of the brain are rare.
Reduced leukocyte infiltration due to the absence of eosinophils in the CNS has been shown to correlate with attenuated tissue damage and more prolonged survival in mice during helminth parasitic disease [Citation136]. Direct interaction between eosinophils and airway epithelial cells after IAV infection has been shown to prevent virus-induced cytopathology in vitro [Citation137]. Human eosinophils constitutively express TLR7 [Citation138], which recognizes virus-derived ssRNA [Citation126] and stimulates the release of eosinophil granule mediators through the adaptor protein myeloid differentiation primary response 88 (MyD88) [Citation129]. Through this TLR7-MyD88-axis, eosinophils mediate respiratory syncytial virus (RSV) clearance, suppressing RSV-induced pathology and airway hyperactivity [Citation129]. Whether such a similar mechanism occurs in the brain during TiLV infection is not known. However, the presence of eosinophilic cells in meningeal areas of the infected tilapia brain raises the question of whether these cells have a beneficial or detrimental role in the innate immune response to TiLV infection in the CNS.
Adaptive immune response
Activated T-cells routinely cross the BBB during immunological surveillance [Citation139,Citation140] whereas activated B cells can be recruited through the BBB, meningeal barriers and CP [Citation141]. This infiltration is partly mediated by the release of proinflammatory cytokines such as IL-1β and IL-8, which can disrupt both the BBB and tight junction integrity of brain endothelial cells, promoting leukocyte trafficking into the CNS [Citation131,Citation132]. The infiltration of mononuclear inflammatory cells has been reported in the brain of TiLV-infected tilapia. During TiLV infection, mononuclear inflammatory cells infiltration is characterized by an initial accumulation at perivascular areas [Citation9,Citation14], followed by infiltration in the regions of viral infection [Citation11],Citation65,Citation108]. Depending on the area of the brain infiltrated (such as the meninges), this can result in pathological conditions such as lymphocytic meningitis, often associated with brain inflammation, also described during TiLV infection [Citation14].
Activated microglia attract dendritic cells (DCs) in mammals [Citation142]. Whether this occurs in the teleost brain is undetermined. The role of DCs in the Trojan horse invasion strategy and neuroinflammation cannot be excluded as these cells also regulate antiviral responses and neuroinflammation [Citation143].
A significant increase in IgM mRNA expression was also described in the brain of TiLV-infected Nile tilapia at 7 dpi [Citation65]. Interestingly, IgM mRNA levels in the brain remain higher than in peripheral organs (kidney, liver, spleen) at 34 dpi, even when a decline of the virus was already observed in the peripheral organs [Citation65].
Intrathecal antibodies in the CSF, indicative of the presence of local antibody-secreting cells (ASCs), are hallmarks of measles virus, poliovirus, rubella virus, mumps virus, herpes simplex virus, and Japanese encephalitis virus (JEV) infections [Citation144]. ASCs can cross the BBB and are critical to the clearance of Sindbis virus [Citation145]. Similarly, during WNV infection in the CNS, an early antibody response is important to contain viraemia and limit dissemination [Citation146]. The increased and sustained production of IgM in the brain during TiLV infection may thus play a critical role in the local clearance of the virus.
Interestingly, gene transcripts encoding fish-specific IgT antibody, initially associated with gut mucosal anti-parasite immunity in teleost fish, are also upregulated in the brains of TiLV- susceptible strains of tilapia during the later stages of infection but downregulated in TiLV-resistant strains [Citation66]. This implicates IgT antibodies in the modulation of systemic immunity, which may affect neuronal immune responses, particularly considering that damage to the BBB due to severe chronic infection by some neurotropic viruses may lead to infiltration of antibodies into the brain. The contribution of both secreted antibodies and activated B-cells to viral clearance in the brain during TiLV infection, therefore, requires further attention. As TiLV persistence in the brain may provide a reservoir for continued virus replication and reinfection, the contribution of secreted antibodies and activated B-cells to viral clearance in the brain may have important implications for disease management and therapy.
Concluding remarks
To date, the sequential stages of TiLV infection progression such as virus penetration at portals of entry and local replication followed by dispersal to target organs, have not been elucidated, and a comprehensive understanding of the neuroanatomy of teleosts, particularly tilapia, is lacking. Studies on TiLV pathogenesis are also limited by the absence of well-developed and annotated in vivo models that recapitulate natural disease pathogenesis. Zebrafish represent an attractive in vivo model, but they are not naturally infected by TiLV and IP challenge models bypass the natural entry and dissemination routes of the virus. It is currently not known to what degree neuropathology is dependent upon host factors (host factors contributing to CNS pathogenesis) as well as which viral factors drive neuroinvasion and brain pathogenesis. This is partly due to the lack of knowledge of the function of most viral genes and proteins and to the lack of knowledge of mechanisms at the interplay between TiLV and host responses.
Although neuronal degeneration has been reported during TiLV infection, the specific infection of neurons by the virus is still to be confirmed. It will also be of interest to determine if TiLV can infect circulating leukocytes, as this would help to elucidate the mechanistic invasion pathways at the BBB or at the BCSFB. Given the potential feco-oral transmission of TiLV, the contribution of alimentary infection and transneural invasion via the gut – brain neural circuit should also be investigated. Likewise, neuroinvasion via the retinal ganglions (eye-brain axis often referred to as blood-ocular barrier) should also be addressed, especially when considering the numerous reports of eye pathology and ocular lesions, particularly exophthalmia [Citation9,Citation11,Citation13,Citation14],Citation52,Citation65,Citation108,Citation147,Citation148].
The blood-CSF interface may play an important role in TiLV dissemination and infection in the brain, particularly when considering that an important neuropathological signature of TiLV infection is leptomeningitis [Citation12,Citation14]. The CP at necropsy and histopathological examinations may provide further insight into its role during TiLV infection, particularly when considering that intrathecal antibodies in the CSF are a hallmark of human CNS infections. Moreover, identification of specific TiLV-infected monocyte subsets in the CP stroma and the clustering of different viral species with specific systemic compartments by viral sequencing may help elucidate the possible dissemination routes of the virus and the contribution of the blood-CSF interface in TiLV disease pathogenesis.
From a more translational perspective, 3D and organoid-based models derived from the natural host may be advantageous to study TiLV-associated neuropathogenesis. In vitro 3D models of the BBB using tilapia brain-derived astrocytes have shown susceptibility to TiLV infection [Citation123]. This model may be a valuable tool for studies of virus-associated neuropathogenesis at the BBB interface. However, the extent to which brain organoids and 3D systems recapitulate the cellular diversity, regional complexity, and circuit functionality of the brain, particularly for tilapia, remains poorly addressed.
The local brain microenvironment may also influence neuropathogenesis. This hypothesis stems from the observation that although all regions of the brain are infected by TiLV, areas of increased permissiveness, notably the forebrain telencephalon (olfactory bulb, hemisphere and periventricular zone) and diencephalon (periventricular zone of the hypothalamus) and hindbrain medulla oblongata (vagal lobe), exist [Citation13]. As such, the appreciation of such neuroanatomical heterogeneity or local brain microenvironments might benefit from high-resolution analyses and exhaustive sampling. Another open question that remains is whether TiLV is an opportunistic pathogen of the CNS or has TiLV evolved to invade the CNS to establish a persistent infection in the brain.
The existence of multiple knowledge gaps in TiLV-related neuropathogenesis provides an opportunity for further research directions, which should follow the clues provided by other neurotropic virus infections presented in this review. This is of particular importance as neurotropic fish viruses such as TiLV pose a serious threat to aquaculture worldwide. Achieving successful antiviral interventions are impossible without a clear understanding of the molecular mechanisms that govern the neuropathogenesis of these viruses.
Author contributions
J.E.K.R., F.N.H., D.S., K.D.T., W.S., K.R., J.M.D., N.G., and M.A. contributed to the conception and design; J.E.K.R. and F.N.H. drafted the initial version of the manuscript, J.E.K.R., F.N.H., D.S., K.D.T., W.S., K.R., J.M.D., N.G., and M.A. revised the manuscript critically for intellectual content; All Authors approved the final version of the manuscript; All Authors agree to be accountable for all aspects of the work.
Acknowledgements
We sincerely thank Dr. John Readman (Department of Infection, Immunity & Inflammation, GOS Institute of Child Health (ICH), University College London) for his wonderful assistance in the conceptualization of the images of the present manuscript.
Disclosure statement
No potential conflict of interest was reported by the author(s).
Data Availability statement
Data sharing does not apply to this article, as no new data were generated or analyzed in this study.
Additional information
Funding
References
- Swanson PA 2nd, McGavern DB. Viral diseases of the central nervous system. Curr Opin Virol. 2015;11:44–20. doi: 10.1016/j.coviro.2014.12.009
- Bandín I, Souto S. Betanodavirus and VER disease: a 30-year research review. Pathogens. 2020;9(2):106. doi: 10.3390/pathogens9020106
- Shlapobersky M, Sinyakov MS, Katzenellenbogen M, et al. Viral encephalitis of tilapia larvae: primary characterization of a novel herpes-like virus. Virology. 2010;399(2):239–47. doi: 10.1016/j.virol.2010.01.001
- Liu W, Zhang Y, Ma J, et al. Determination of a novel parvovirus pathogen associated with massive mortality in adult tilapia. PLoS Pathog. 2020;16(9):e1008765. doi: 10.1371/journal.ppat.1008765
- Lensink MJ, Li Y, Lequime S, et al. Aquatic Flaviviruses. J Virol. 2022;96(17):e00439–22. doi: 10.1128/jvi.00439-22
- Geoghegan JL, Di Giallonardo F, Cousins K, et al. Hidden diversity and evolution of viruses in market fish. Virus Evol. 2018;4(2):vey031. doi: 10.1093/ve/vey031
- Skoge RH, Brattespe J, Økland AL, et al. New virus of the family Flaviviridae detected in lumpfish (cyclopterus lumpus). Arch Virol. 2018;163(3):679–685. doi: 10.1007/s00705-017-3643-3
- Soto E, Camus A, Yun S, et al. First isolation of a novel aquatic flavivirus from chinook salmon (Oncorhynchus tshawytscha) and its in vivo replication in a piscine animal model. J Virol. 2020;94(15):e00337–20. doi: 10.1128/JVI.00337-20
- Eyngor M, Zamostiano R, Kembou Tsofack JE, et al. Identification of a novel RNA virus lethal to tilapia. J Clin Microbiol. 2014;52(12):4137–4146. doi: 10.1128/JCM.00827-14
- Kembou-Ringert JE, Steinhagen D, Readman J, et al. Tilapia lake virus vaccine development: a review on the recent advances. Vaccines. 2023;11(2):251. doi: 10.3390/vaccines11020251
- Tattiyapong P, Dachavichitlead W, Surachetpong W. Experimental infection of Tilapia Lake Virus (TiLV) in Nile tilapia (Oreochromis niloticus) and red tilapia (Oreochromis spp. Vet Microbiol. 2017;207:170–177. doi: 10.1016/j.vetmic.2017.06.014
- Amal MNA, Koh CB, Nurliyana M, et al. A case of natural co-infection of tilapia lake virus and Aeromonas veronii in a Malaysian red hybrid tilapia (Oreochromis niloticus× O. mossambicus) farm experiencing high mortality. Aquaculture. 2018;485:12–16. doi: 10.1016/j.aquaculture.2017.11.019
- Dinh-Hung N, Sangpo P, Kruangkum T, et al. Dissecting the localization of tilapia tilapinevirus in the brain of the experimentally infected Nile tilapia, Oreochromis niloticus (L.). J Fish Dis. 2021;44(8):1053–1064. doi: 10.1111/jfd.13367
- Basri L, Nor RM, Salleh A, et al. Co-infections of Tilapia Lake Virus, Aeromonas hydrophila and streptococcus agalactiae in farmed red hybrid Tilapia. Animals (Basel). 2020;10(11):2141. doi: 10.3390/ani10112141
- Mojzesz M, Widziolek M, Adamek M, et al. Tilapia lake virus-induced neuroinflammation in zebrafish: microglia activation and sickness behavior. Front Immunol. 2021;12:760882. doi: 10.3389/fimmu.2021.760882
- Mani A, Salinas I. The knowns and many unknowns of CNS immunity in teleost fish. Fish Shellfish Immunol. 2022;131:431–440. doi: 10.1016/j.fsi.2022.10.013
- Roberts RJ, Ellis AE. Chapter 2 - the anatomy and physiology of teleosts. In: Roberts RJ, editor. Fish pathol. 4th ed. Wiley Blackwell. 2012. p. 17–61.
- Sado RY, de Souza FC, Behr ER, et al. Chapter 2 - anatomy of teleosts and elasmobranchs. In: Baldisserotto B, EC Urbinati JEP Cyrino, editors. Biology and physiology of freshwater neotropical fish. Academic Press; 2020. p. 21–47. doi:10.1016/B978-0-12-815872-2.00002-6
- Vernier P. “1.04 – the brains of teleost fishes.” Evolution Nerv Sys 1. 2017;1:59–75.
- Hotz JM, Thomas JR, Katz EN, et al. ATP-binding cassette transporters at the zebrafish blood-brain barrier and the potential utility of the zebrafish as an in vivo model. Cancer Drug Resist. 2021;4(3):620–633. doi: 10.20517/cdr.2021.35
- O’Brown NM, Pfau SJ, Gu C. Bridging barriers: a comparative look at the blood-brain barrier across organisms. Genes Dev. 2018;32:466–478. doi: 10.1101/gad.309823.117
- Chen J, Poskanzer KE, Freeman MR, et al. Live-imaging of astrocyte morphogenesis and function in zebrafish neural circuits. Nat Neurosci. 2020;23(10):1297–306. doi: 10.1038/s41593-020-0703-x
- Daneman R, Prat A The blood–brain barrier. Cold Spring Harb Perspect Biol. 2015;7:a020412. doi: 10.1101/cshperspect.a020412 1
- Wang Y, Pan L, Moens CB, et al. Notch3 establishes brain vascular integrity by regulating pericyte number. Development. 2014;141(2):307–17. doi: 10.1242/dev.096107
- Ando K, Fukuhara S, Izumi N, et al. Clarification of mural cell coverage of vascular endothelial cells by live imaging of zebrafish. Development. 2016;143:1328–39. doi: 10.1242/dev.132654
- Korn J, Christ B, Kurz H. Neuroectodermal origin of brain pericytes and vascular smooth muscle cells. J Comp Neurol. 2002;442(1):78–88. doi: 10.1002/cne.1423
- Cuoghi B, Mola L. Macroglial cells of the teleost central nervous system: a survey of the main types. Cell Tissue Res. 2009;338(3):319–332. doi: 10.1007/s00441-009-0870-2
- Wu S, Nguyen LTM, Pan H, et al. Two phenotypically and functionally distinct microglial populations in adult zebrafish. Sci Adv. 2020;6(47):eabd1160. doi: 10.1126/sciadv.abd1160
- Zupanc GKH. Adult neurogenesis in the central nervous system of teleost fish: from stem cells to function and evolution. J Exp Biol. 2021;224(8):jeb226357. doi: 10.1242/jeb.226357
- Koonin EV, Krupovic M, Surachetpong W, et al. Ictv virus taxonomy profile: amnoonviridae 2023. J Gen Virol. 2023 Oct;104(10). doi: 10.1099/jgv.0.001903
- Bacharach E, Mishra N, Briese T, et al. Characterization of a novel orthomyxo-like virus causing mass die-offs of tilapia. MBio. 2016;7(2):e00431–16. doi: 10.3390/pathogens9020106
- Ferguson HW, Kabuusu R, Beltran S, et al. Syncytial hepatitis of farmed tilapia, Oreochromis niloticus (L.): a case report. J Fish Dis. 2014;37(6):583–589. doi: 10.1111/jfd.12142
- Del-Pozo J, Mishra N, Kabuusu R, et al. Syncytial Hepatitis of Tilapia (Oreochromis niloticus L.) is associated with orthomyxovirus-like virions in hepatocytes. Vet Pathol. 2017;54(1):164–170. doi: 10.1177/0300985816658100
- Abu Rass R, Kustin T, Zamostiano R, et al. Inferring protein function in an emerging virus: detection of the nucleoprotein in tilapia lake virus. J Virol. 2022;96(6):e0175721. doi: 10.1128/JVI.01757-21
- Yamkasem J, Piewbang C, Techangamsuwan S, et al. Susceptibility of ornamental African cichlids Aulonocara spp. to experimental infection with Tilapia lake virus. Aquaculture. 2021;542:736920. doi: 10.1016/j.aquaculture.2021.736920
- Abdullah A, Pazai AMM, Ridzuan MSM, et al. Persistent detection of tilapia lake virus in wild tilapia and tinfoil barbs. Vet World. 2022;15(4):1097–106. doi: 10.14202/vetworld.2022.1097-1106
- Abdullah A, Ramly R, Mohammad Ridzwan MS, et al. First detection of tilapia lake virus (TiLV) in wild river carp (Barbonymus schwanenfeldii) at Timah Tasoh lake, Malaysia. J Fish Dis. 2018;41(9):1459–62. doi: 10.1111/jfd.12843
- Jaemwimol P, Rawiwan P, Tattiyapong P, et al. Susceptibility of important warm water fish species to tilapia lake virus (TiLV) infection. Aquaculture. 2018;497:462–8. doi: 10.1016/j.aquaculture.2018.08.028
- Paria A, Yadav SC, Verma DK, et al. Susceptibility of selected tropical non-tilapine ornamental cichlids to Tilapia tilapinevirus following experimental infection. Aquaculture. 2023;567:739224. doi: 10.1016/j.aquaculture.2022.739224
- Rakus K, Mojzesz M, Widziolek M, et al. Antiviral response of adult zebrafish (Danio rerio) during tilapia lake virus (TiLV) infection. Fish Shellfish Immunol. 2020;101:1–8. doi: 10.1016/j.fsi.2020.03.040
- Widziolek M, Janik K, Mojzesz M, et al. Type I interferon-dependent response of zebrafish larvae during tilapia lake virus (TiLV) infection. Dev Comp Immunol. 2021;116:103936. doi: 10.1016/j.dci.2020.103936
- Adamek M, Matras M, Surachetpong W, et al. How susceptible are rainbow trout and brown trout to infection with tilapia lake virus at increased water temperature – is there any potential for climate change driven host jump? Aquaculture. 2023;571:739469. doi: 10.1016/j.aquaculture.2023.739469
- Senapin S, Shyam KU, Meemetta W, et al. Inapparent infection cases of tilapia lake virus (TiLV) in farmed tilapia. Aquaculture. 2018;487(6):51–55. doi: 10.1016/j.aquaculture.2018.01.007
- Dong HT, Siriroob S, Meemetta W, et al. Emergence of tilapia lake virus in Thailand and an alternative semi-nested RT-PCR for detection. Aquaculture. 2017;476:111–8. doi: 10.1016/j.aquaculture.2017.04.019
- Dong HT, Ataguba GA, Khunrae P, et al. Evidence of TiLV infection in tilapia hatcheries in Thailand from 2012 to 2017 reveals probable global spread of the disease. Aquaculture. 2017;479:579–583. doi: 10.1016/j.aquaculture.2017.06.035
- Surachetpong W, Janetanakit T, Nonthabenjawan N, et al. Outbreaks of Tilapia Lake Virus Infection, Thailand, 2015-2016. Emerg Infect Dis. 2017;23(6):1031–1033. doi:
- Nicholson P, Fathi MA, Fischer A, et al. Detection of Tilapia Lake Virus in Egyptian fish farms experiencing high mortalities in 2015. J Fish Dis. 2017;40(12):1925–1928. doi: 10.1111/jfd.12650
- Piewbang C, Tattiyapong P, Techangamsuwan S, et al. Tilapia lake virus immunoglobulin G (TiLV IgG) antibody: Immunohistochemistry application reveals cellular tropism of TiLV infection. Fish Shellfish Immunol. 2021;116:115–23. doi: 10.1016/j.fsi.2021.06.017
- Kembou Tsofack JE, Zamostiano R, Watted S, et al. Detection of tilapia lake virus in clinical samples by culturing and Nested Reverse Transcription-PCR. J Clin Microbiol. 2017;55(3):759–767. doi: 10.1128/JCM.01808-16
- Yamkasem J, Tattiyapong P, Kamlangdee A, et al. Evidence of potential vertical transmission of tilapia lake virus. J Fish Dis. 2019;42(9):1293–1300. doi: 10.1111/jfd.13050
- Dong HT, Senapin S, Gangnonngiw W, et al. Experimental infection reveals transmission of tilapia lake virus (TiLV) from tilapia broodstock to their reproductive organs and fertilized eggs. Aquaculture. 2020;515:734541. doi: 10.1016/j.aquaculture.2019.734541
- Pierezan F, Yun S, Surachetpong W, et al. Intragastric and intracoelomic injection challenge models of tilapia lake virus infection in Nile tilapia (Oreochromis niloticus L.) fingerlings. J Fish Dis. 2019;42(9):1301–1307. doi: 10.1111/jfd.13052
- Surachetpong W, Roy SRK, Nicholson P Tilapia lake virus: The story so far. J Fish Dis. 2020;43:1115–1132. doi: 10.1111/jfd.13237 10
- Taengphu S, Kayansamruaj P, Kawato Y, et al. Concentration and quantification of tilapia tilapinevirus from water using a simple iron flocculation coupled with probe-based RT-qPCR. PeerJ. 2022;10:e13157. doi: 10.7717/peerj.13157
- Yamkasem J, Prasartset T, Tattiyapong P, et al. Persistence of tilapia tilapinevirus in fish rearing and environmental water and its ability to infect cell line. J Fish Dis. 2022;45(5):679–685. doi: 10.1111/jfd.13593
- Souto S, Olveira JG, Alonso MC, et al. Betanodavirus infection in bath-challenged solea senegalensis juveniles: a comparative analysis of RGNNV, SJNNV and reassortant strains. J Fish Dis. 2018;41(10):1571–1578. doi: 10.1111/jfd.12865
- Tanaka S, Takagi M, Miyazaki T. Histopathological studies on viral nervous necrosis of sevenband grouper, Epinephelus septemfasciatus Thunberg, at the grow-out stage. J Fish Dis. 2004;27(7):385–399. doi: 10.1111/j.1365-2761.2004.00559.x
- Skliris GP, Richards RH. Induction of nodavirus disease in seabass, dicentrarchus labrax, using different infection models. Virus Res. 1999;63(1–2):85–93. doi: 10.1016/s0168-1702(99)00061-1
- Turner JK, Sakulpolwat S, Sukdanon S, et al. Tilapia lake virus (TiLV) causes severe anaemia and systemic disease in tilapia. J Fish Dis. 2023;46(6):643–651. doi: 10.1111/jfd.13775
- Abu Rass R, Kembou-Ringert JE, Zamostiano R, et al. Mapping of tilapia lake virus entry pathways with inhibitors reveals dependence on dynamin activity and cholesterol but not endosomal acidification. Front Cell Develop Biol. 2022;10. doi: 10.3389/fcell.2022.1075364
- Schweighardt B, Atwood WJ. Virus receptors in the human central nervous system. J Neurovirol. 2001;7(3):187–195. doi: 10.1080/13550280152403236
- Van Gennip JLM, Boswell CW, Ciruna B. Neuroinflammatory signals drive spinal curve formation in zebrafish models of idiopathic scoliosis. Sci Adv. 2018 Dec;4(12):eaav1781. doi: 10.1126/sciadv.aav1781
- Schnell MJ, McGettigan JP, Wirblich C, et al. The cell biology of rabies virus: using stealth to reach the brain. Nat Rev Microbiol. 2010;8(1):51–61. doi: 10.1038/nrmicro2260
- Behera BK, Pradhan PK, Swaminathan TR, et al. Emergence of Tilapia Lake virus associated with mortalities of farmed Nile tilapia Oreochromis niloticus (Linnaeus,1758) in India. Aquaculture. 2018;484:168–174. doi: 10.1016/j.aquaculture.2017.11.025
- Mugimba KK, Lamkhannat M, Dubey S, et al. Tilapia lake virus downplays innate immune responses during early stage of infection in Nile tilapia (Oreochromis niloticus). Sci Rep. 2020;10(1):20364. doi: 10.1038/s41598-020-73781-y
- Adamek M, Rebl A, Matras M, et al. Immunological insights into the resistance of Nile tilapia strains to an infection with tilapia lake virus. Fish Shellfish Immunol. 2022;124:118–33. doi: 10.1016/j.fsi.2022.03.027
- Cain MD, Salimi H, Diamond MS, et al. Mechanisms of pathogen invasion into the central nervous system. Neuron. 2019;103(5):771–783. doi: 10.1016/j.neuron.2019.07.015
- Verma S, Kumar M, Gurjav U, et al. Reversal of West Nile virus-induced blood-brain barrier disruption and tight junction proteins degradation by matrix metalloproteinases inhibitor. Virology. 2010;397(1):130–138. doi: 10.1016/j.virol.2009.10.036
- Casiraghi C, Dorovini-Zis K, Horwitz MS. Epstein-Barr virus infection of human brain microvessel endothelial cells: a novel role in multiple sclerosis. J Neuroimmunol. 2011;230(1–2):173–177. doi: 10.1016/j.jneuroim.2010.08.003
- Passoni G, Langevin C, Palha N, et al. Imaging of viral neuroinvasion in the zebrafish reveals That Sindbis and Chikungunya viruses favour different entry routes. Dis Model Mech. 2017;10(7):847–857. doi: 10.1242/dmm.029231
- Koyuncu OO, Hogue IB, Enquist LW. Virus infections in the nervous system. Cell Host Microbe. 2013;13(4):379–93. doi: 10.1016/j.chom.2013.03.010
- Xu Z, Waeckerlin R, Urbanowski MD, et al. West Nile virus infection causes endocytosis of a specific subset of tight junction membrane proteins. PLoS One. 2012;7(5):e37886. doi: 10.1371/journal.pone.0037886
- Wang T, Town T, Alexopoulou L, et al. Toll-like receptor 3 mediates West Nile virus entry into the brain causing lethal encephalitis. Nat Med. 2004;10(12):1366–1373. doi: 10.1038/nm1140
- Boothpur R, Brennan DC. Human polyoma viruses and disease with emphasis on clinical BK and JC. J Clin Virol. 2010;47(4):306–312. doi: 10.1016/j.jcv.2009.12.006
- Ugolini G. Rabies virus as a transneuronal tracer of neuronal connections. Adv Virus Res. 2011;79:165–202. doi: 10.1016/B978-0-12-387040-7.00010-X
- Zampieri N, Jessell TM, Murray AJ. Mapping sensory circuits by anterograde transsynaptic transfer of recombinant rabies virus. Neuron. 2014;81(4):766–778. doi: 10.1016/j.neuron.2013.12.033
- Bauer A, Nolden T, Schröter J, et al. Anterograde glycoprotein-dependent transport of newly generated rabies virus in dorsal root ganglion neurons. J Virol. 2014;88(24):14172–14183. doi: 10.1128/JVI.02254-14
- Samuel MA, Wang H, Siddharthan V, et al. Axonal transport mediates West Nile virus entry into the central nervous system and induces acute flaccid paralysis. Proc Natl Acad Sci, USA. 2007;104(43):17140–17145. doi: 10.1073/pnas.0705837104
- Munster V, Prescott JB, Bushmaker T, et al. Rapid Nipah virus entry into the central nervous system of hamsters via the olfactory route. Nat Sci Rep. 2012;2(1):736. doi: 10.1038/srep00736
- Nguyen HD, Nakai T, Muroga K. Progression of striped jack nervous necrosis virus (SJNNV) infection in naturally and experimentally infected striped jack pseudocaranx dentex larvae. Dis Aquat Organ. 1996;24:99–105. doi: 10.3354/dao024099
- Mladineo I. The immunohistochemical study of nodavirus changes in larval, juvenile and adult sea bass tissue. J Appl Ichthyol. 2003;19(6):366–370. doi: 10.1111/j.1439-0426.2003.00489.x
- Sundin L, Nilsson S. Branchial innervation. J Exp Zool. 2002;293(3):232–48. doi: 10.1002/jez.10130
- Marshall EM, Koopmans MPG, Rockx B. A journey to the central nervous system: routes of flaviviral neuroinvasion in human disease. Viruses. 2022;14(10):2096. doi: 10.3390/v14102096
- Pulgar VM. Transcytosis to cross the blood brain barrier, new advancements and challenges. Front Neurosci. 2019;12:1019. doi: 10.3389/fnins.2018.01019
- Stewart PA. Endothelial vesicles in the blood-brain barrier: are they related to permeability? Cell Mol Neurobiol. 2000;20(2):149–163. doi: 10.1023/a:1007026504843
- Villasenor R, Collin L High-resolution confocal imaging of the blood-brain barrier: imaging, 3D reconstruction, and quantification of transcytosis. J Vis Exp. 2017;129:e59407. doi: 10.3791/56407 129
- Greene MW, Chen TT. Characterization of teleost insulin receptor family members. Gen Comp Endocrinol. 1999;115(2):254–69. doi: 10.1006/gcen.1999.7293
- Mayor S, Pagano RE. Pathways of clathrin-independent endocytosis. Nat Rev Mol Cell Biol. 2007;8(8):603–612. doi: 10.1038/nrm2216
- Preston JE, Abbott NJ, Begley DJ. Transcytosis of macromolecules at the blood-brain barrier. Adv Pharmacol. 2014;71:147–163. doi: 10.1016/bs.apha.2014.06.001
- Lin XP, Mintern JD, Gleeson PA. Macropinocytosis in different cell types: similarities and differences. Membranes. 2020;10(8):177. doi: 10.3390/membranes10080177
- Hasebe R, Suzuki T, Makino Y, et al. Transcellular transport of West Nile virus-like particles across human endothelial cells depends on residues 156 and 159 of envelope protein. BMC Microbiol. 2010;10(1):165. doi: 10.1186/1471-2180-10-165
- Liu NQ, Lossinsky AS, Popik W, et al. Human immunodeficiency virus type 1 enters brain microvascular endothelia by macropinocytosis dependent on lipid rafts and the mitogen-activated protein kinase signaling pathway. J Virol. 2002;76(13):6689–6700. doi: 10.1128/JVI.76.13.6689-6700.2002
- Lertwanakarn T, Khemthong M, Tattiyapong P, et al. The modulation of immune responses in Tilapinevirus tilapiae-infected fish cells through MAPK/ERK signalling. Viruses. 2023;15(4):900. doi: 10.3390/v15040900
- Daniels BP, Holman DW, Cruz-Orengo L, et al. Viral pathogen-associated molecular patterns regulate blood-brain barrier integrity via competing innate cytokine signals. MBio. 2014;5(5):e01476–14. doi: 10.1128/mBio.01476-14
- Rempe RG, Hartz AMS, Bauer B Matrix metalloproteinases in the brain and blood–brain barrier: versatile breakers and makers. J Cereb Blood Flow Metab. 2016;36:1481–1507. doi: 10.1177/0271678X16655551 9
- Strazza M, Pirrone V, Wigdahl B, et al. Breaking down the barrier: the effects of HIV-1 on the blood–brain barrier. Brain Res. 2011;1399:96–115. doi: 10.1016/j.brainres.2011.05.015
- Wang K, Wang H, Lou W, et al. IP-10 promotes blood-brain barrier damage by inducing tumor necrosis factor alpha production in Japanese encephalitis. Front Immunol. 2018;9:1148. doi: 10.3389/fimmu.2018.01148
- Man SM, Karki R, Kanneganti TD. Molecular mechanisms and functions of pyroptosis, inflammatory caspases and inflammasomes in infectious diseases. Immunol Rev. 2017;277(1):61–75. doi: 10.1111/imr.12534
- Yogarajah T, Ong KC, Perera D, et al. AIM2 inflammasome-mediated pyroptosis in enterovirus A71-infected neuronal cells restricts viral replication. Sci Rep. 2017;7(1):5845. doi: 10.1038/s41598-017-05589-2
- de Sousa JR, Azevedo R, Martins Filho AJ, et al. In situ inflammasome activation results in severe damage to the central nervous system in fatal Zika virus microcephaly cases. Cytokine. 2018;111:255–264. doi: 10.1016/j.cyto.2018.08.008
- Keck F, Brooks-Faulconer T, Lark T, et al. Altered mitochondrial dynamics as a consequence of Venezuelan Equine encephalitis virus infection. Virulence. 2017;8(8):1849–1866. doi: 10.1080/21505594.2016.1276690
- Raksaseri P, Lertwanakarn T, Tattiyapong P, et al. Tilapia lake virus causes mitochondrial damage: a proposed mechanism that leads to extensive death in fish cells. PeerJ. 2023;11:e16190. doi: 10.7717/peerj.16190
- Erbar S, Maisner A. Nipah virus infection and glycoprotein targeting in endothelial cells. Virol J. 2010;7(1):305. doi: 10.1186/1743-422X-7-305
- Rockx B, Brining D, Kramer J, et al. Clinical outcome of henipavirus infection in hamsters is determined by the route and dose of infection. J Virol. 2011;85(15):7658–7671. doi: 10.1128/JVI.00473-11
- Park BH, Lavi E, Blank KJ, et al. Intracerebral hemorrhages and syncytium formation induced by endothelial cell infection with a murine leukemia virus. J Virol. 1993;67(10):6015–6024. doi: 10.1128/JVI.67.10.6015-6024.1993
- Jansen MD, Dong HT, Mohan CV. Tilapia lake virus: a threat to the global tilapia industry? Rev Aquacult. 2019;11(3):725–739. doi: 10.1111/raq.12254
- Debnath PP, Delamare-Deboutteville J, Jansen MD, et al. Two-year surveillance of tilapia lake virus (TiLV) reveals its wide circulation in tilapia farms and hatcheries from multiple districts of Bangladesh. J Fish Dis. 2020;43(11):1381–1389. doi: 10.1111/jfd.13235
- Haridas L, George MR, John KR, et al. Early innate immune responses of Nile tilapia (Oreochromis niloticus) during tilapia lake virus (TiLV) infection. Aquaculture. 2023;563(1):738962. doi: 10.1016/j.aquaculture.2022.738962
- Butler AB, Hodos W. Comparative Vertebrate Neuroanatomy: Evolution and Adaptation. John Wiley & Sons 2005; doi: 10.1002/0471733849
- Engelhardt B, Sorokin L. The blood–brain and the blood–cerebrospinal fluid barriers: function and dysfunction. Semin Immunopathol. 2009;31(4):497–511. doi: 10.1007/s00281-009-0177-0
- Sakka L, Coll G, Chazal J. Anatomy and physiology of cerebrospinal fluid. Eur Ann Otorhinolaryngol Head Neck Dis. 2011;128(6):309–16. doi: 10.1016/j.anorl.2011.03.002
- Johanson CE, Stopa EG, McMillan PN. The blood–cerebrospinal fluid barrier: structure and functional significance. Methods Mol Biol. 2011;686:101–131. doi: 10.1007/978-1-60761-938-3_4
- Castranova D, Samasa B, Venero Galanternik M, et al. Live imaging of intracranial lymphatics in the zebrafish. Circ Res. 2021;128(1):42–58. doi: 10.1161/CIRCRESAHA.120.317372
- Ludlow M, Kortekaas J, Herden C, et al. Neurotropic virus infections as the cause of immediate and delayed neuropathology. Acta Neuropathol. 2016;131(2):159–184. doi: 10.1007/s00401-015-1511-3
- Kim J, Alejandro B, Hetman M, et al. Zika virus infects pericytes in the choroid plexus and enters the central nervous system through the blood-cerebrospinal fluid barrier. PLoS Pathog. 2020;16(5):841437. doi: 10.1371/journal.ppat.1008204
- Rubin S, Eckhaus M, Rennick LJ, et al. Molecular biology, pathogenesis and pathology of mumps virus. J Pathol. 2015;235(2):242–52. doi: 10.1002/path.4445
- Gomes I, Karmirian K, Oliveira JT, et al. SARS-CoV-2 infection of the central nervous system in a 14-month-old child: a case report of a complete autopsy. Lancet Reg Health Am. 2021;2:100046. doi: 10.1016/j.lana.2021.100046
- Pellegrini L, Albecka A, Mallery DL, et al. Sars-cov-2 infects the brain choroid plexus and disrupts the blood-CSF barrier in human brain organoids. Cell Stem Cell. 2020;27(6):951–961.e5. doi: 10.1016/j.stem.2020.10.001
- Kanoh Y, Ohara T, Kanoh M, et al. Serum matrix metalloproteinase-2 levels indicate blood–CSF barrier damage in patients with infectious meningitis. Inflammation. 2008;31(2):99–104. doi: 10.1007/s10753-007-9054-y
- Vandenbroucke R, Dejonckheere E, Van Lint P, et al. Matrix metalloprotease 8-dependent extracellular matrix cleavage at the blood-CSF barrier contributes to lethality during systemic inflammatory diseases. J Neurosci. 2012;32(29):9805–9816. doi: 10.1523/JNEUROSCI.0967-12.2012
- Hunsperger EA, Roehrig JT. Nocodazole delays viral entry into the brain following footpad inoculation with West Nile virus in mice. J Neurovirol. 2009;15(3):211–218. doi: 10.1080/13550280902913255
- Bauer L, Laksono BM, de Vrij FMS, et al. The neuroinvasiveness, neurotropism, and neurovirulence of SARS-CoV-2. Trends Neurosci. 2022;45(5):358–368. doi: 10.1016/j.tins.2022.02.006
- Li Y, Huang Y, Cai J, et al. Establishment of an astrocyte-like cell line from the brain of tilapia (Oreochromis niloticus) for virus pathogenesis and a vitro model of the blood-brain barrier. J Fish Dis. 2022;45(10):1451–1462. doi:
- Aich N, Paul A, Choudhury TC, et al. Tilapia Lake Virus (TiLV) disease: Current status of understanding. Aqua Fish. 2022;7(1):7–17. doi:
- Pierezan F, Yun S, Piewbang C, et al. Pathogenesis and immune response of Nile tilapia (Oreochromis niloticus) exposed to tilapia lake virus by intragastric route. Fish Shellfish Immunol. 2020;107(Pt A):289–300. doi: 10.1016/j.fsi.2020.10.019
- Kembou-Ringert JE, Steinhagen D, Thompson KD, et al. Immune responses to tilapia lake virus infection: what we know and what we don’t know. Front Immunol. 2023;14:1240094. doi: 10.3389/fimmu.2023.1240094
- Mugimba KK, Tal S, Dubey S, et al. Gray (Oreochromis niloticus x O. aureus) and Red (Oreochromis spp.) tilapia show equal susceptibility and proinflammatory cytokine responses to experimental tilapia lake virus infection. Viruses. 2019;11(10):893. doi: 10.3390/v11100893
- Diebold SS, Kaisho T, Hemmi H, et al. Innate antiviral responses by means of TLR7-mediated recognition of single-stranded RNA. Science. 2004;303(5663):1529–1531. doi: 10.1126/science.1093616
- Phipps S, Lam CE, Mahalingam S, et al. Eosinophils contribute to innate antiviral immunity and promote clearance of respiratory syncytial virus. Blood. 2007;110(5):1578–86. doi: 10.1182/blood-2007-01-071340
- Chai Q, He WQ, Zhou M, et al. Enhancement of blood-brain barrier permeability and reduction of tight junction protein expression are modulated by chemokines/cytokines induced by rabies virus infection. J Virol. 2014;88(9):4698–710. doi: 10.1128/jvi.03149-13
- McCandless EE, Zhang B, Diamond MS, et al. CXCR4 antagonism increases T cell trafficking in the central nervous system and improves survival from West Nile virus encephalitis. Proc Natl Acad Sci, USA. 2008;105(32):11270–5. doi: 10.1073/pnas.0800898105
- Zhao L, Toriumi H, Kuang Y, et al. The roles of chemokines in rabies virus infection: overexpression may not always be beneficial. J Virol. 2009;83(22):11808–18. doi: 10.1128/jvi.01346-09
- Chen Z, Zhong D, Li G. The role of microglia in viral encephalitis: a review. J Neuroinflammation. 2019;16(1):76. doi: 10.1186/s12974-019-1443-2
- Liddelow SA, Barres BA. Reactive astrocytes: production, function, and therapeutic potential. Immunity. 2017;46(6):957–967. doi: 10.1016/j.immuni.2017.06.006
- Soung A, Klein RS. Viral encephalitis and neurologic diseases: focus on astrocytes. Trends Mol Med. 2018;24(11):950–962. doi: 10.1016/j.molmed.2018.09.001
- Mishra PK, Li Q, Munoz LE, et al. Reduced leukocyte infiltration in absence of eosinophils correlates with decreased tissue damage and disease susceptibility in ΔdblGATA mice during murine neurocysticercosis. PLoS Negl Trop Dis. 2016;10(6):e0004787. doi: 10.1371/journal.pntd.0004787
- Tiwary M, Rooney RJ, Liedmann S, et al. Eosinophil responses at the airway epithelial barrier during the early phase of influenza a virus infection in C57BL/6 mice. Cells. 2021;10(3):509. doi: 10.3390/cells10030509
- Nagase H, Okugawa S, Ota Y, et al. Expression and function of Toll-like receptors in eosinophils: activation by Toll-like receptor 7 ligand. J Immunol. 2003;171(8):3977–3982. doi: 10.4049/jimmunol.171.8.3977
- Irani DN, Griffin DE. Regulation of lymphocyte homing into the brain during viral encephalitis at various stages of infection. J Immunol. 1996;156(10):3850–7. doi: 10.4049/jimmunol.156.10.3850
- Hickey WF, Hsu BL, Kimura H. T-lymphocyte entry into the central nervous system. J Neurosci Res. 1991;28(2):254–60. doi: 10.1002/jnr.490280213
- Jain RW, Yong VW. B cells in central nervous system disease: diversity, locations and pathophysiology. Nat Rev Immunol. 2022;22(8):513–524. doi: 10.1038/s41577-021-00652-6
- Gallizioli M, Miró-Mur F, Otxoa-de-Amezaga A, et al. Dendritic cells and microglia have non-redundant functions in the inflamed brain with protective effects of type 1 cDcs. Cell Rep. 2020;33(3):108291. doi: 10.1016/j.celrep.2020.108291
- Constant O, Maarifi G, Blanchet FP, et al. Role of dendritic cells in viral brain infections. Front Immunol. 2022;13:862053. doi: 10.3389/fimmu.2022.862053
- Phares TW, Stohlman SA, Bergmann CC. Intrathecal humoral immunity to encephalitic RNA viruses. Viruses. 2013;5(2):732–752. doi: 10.3390/v5020732
- Nilaratanakul V, Chen J, Tran O, et al. Germ line IgM is sufficient, but not required, for antibody-mediated Alphavirus Clearance from the central nervous system. J Virol. 2018;92(7):e02081–17. doi: 10.1128/JVI.02081-17
- Diamond M, Sitati EM, Friend LD, et al. A critical role for induced IgM in the protection against west Nile virus infection. J Exp Med. 2003;198(12):1853–1862. doi: 10.1084/jem.20031223
- Sood N, Verma DK, Paria A, et al. Transcriptome analysis of liver elucidates key immune-related pathways in Nile tilapia Oreochromis niloticus following infection with tilapia lake virus. Fish Shellfish Immunol. 2021;111:208–19. doi: 10.1016/j.fsi.2021.02.005
- Hossain M, Uddin I, Hossain M, et al. Molecular detection of tilapia lake virus (TiLV) in farmed mono-sex Nile tilapia (Tilapia niloticus) in Bangladesh. Asian J Sci Res. 2020;13(1):67–78. doi: 10.3923/ajsr.2020.67.78