Abstract
Titanium dioxide nanoparticles (nTiO2) are found in numerous manufactured products. While a few studies have been carried out to measure the efficiency of chemical protective clothing and gloves against nanoparticles (NPs), they have generally not considered the conditions prevailing in occupational settings. This study was designed to evaluate the resistance of protective clothing against NPs under conditions simulating occupational use. Nitrile and butyl rubber gloves, as well as cotton/polyester woven and polyolefin non-woven clothing samples were placed into contact with nTiO2 in the form of powders or colloidal solutions. Simultaneously, mechanical deformations were applied to the samples. Preliminary results showed that nTiO2 may penetrate some of the materials after prolonged dynamic deformations and/or when the NPs are in colloidal solutions. The effect was partly attributed to modifications in the physical and mechanical properties of protective materials that were induced by repetitive mechanical deformations.
1. Introduction
The National Science Foundation has estimated that the number of nano-products manufactured around the world will double every three years until 2020 Citation1. At the same time, the number of workers and researchers in the industry will attain six million people. Nanoparticles (NPs), particularly titanium dioxide nanoparticles (nTiO2), are increasingly present in commercial products such as paints, varnishes and sunscreens Citation2Citation3 and as such are a new potential source of hazard. Indeed, increasing numbers of studies are cautioning their likely harmful effects on health. For example, a small increase in the number of cancers among workers in contact with nTiO2 has been reported Citation4. Moreover, studies conducted on rats and mice, exposed to 250-nm TiO2 pigment particles and 20-nm nTiO2, observed a greater occurrence of pulmonary effects Citation5. In addition, the observed inflammatory response was higher with nTiO2 Citation6Citation7. Based on these results, the International Agency for Research on Cancer has classified nanosized titanium dioxide in the 2B-group, as being possibly carcinogenic to humans Citation8. In response to this classification, several agencies have recommended the application of the precautionary principle Citation9. While this implies the use of protective gloves and clothing, for the most part, they have not yet been validated for use with NPs.
With respect to the limited number of studies that have examined the efficiency of protective clothing and gloves exposed to NPs, most involved aerosols. Furthermore, conclusions reached on the efficiency of protective gloves appear to be conflicting. For example, while the penetration of 30 and 80 nm graphite NPs has been reported for nitrile, vinyl, latex and neoprene commercial glove samples Citation10, no penetration was observed for the same glove models for 40 nm graphite, 10 nm TiO2 or Pt NPs Citation11Citation12. In the case of air-permeable fabrics, tests have been performed with oleic acid, KCl, NaCl, graphite, TiO2 and Pt nano-aerosols with diameters as small as 10 nm Citation11Citation13. Several authors have determined the variation of NP penetration through fabrics to be function of the particle diameter and air flow rate, in agreement with the filtration theory Citation14Citation15. Nonetheless, a much higher efficiency against NP penetration was measured with a thin high-density polyethylene (PE) non-woven membrane than with other, thicker fabrics, both with and without air flow Citation11Citation12.
In occupational settings, exposure to NPs may also involve both colloidal solutions and powders. This situation is especially relevant to protective clothing and gloves. For example, Ahn et al. Citation16 exposed nitrile, latex and cotton glove samples to nanoclay and alumina nanopowder. Scanning electron microscope (SEM) observations revealed the accumulation of the NPs in micrometer-size pores at the surface of the nitrile and latex gloves. Furthermore, large quantities of NPs were found on and within the fibres of the cotton glove samples. Penetration through glove materials is likely to be facilitated by the presence of a liquid carrier. In addition, the polymers forming the gloves are often sensitive to solvents such that some of them may sustain significant swelling Citation17Citation18. Finally, when they are in service, protective clothing and gloves are subjected to mechanical constraints, for example, biaxial strains at interphalangeal joints, elbows and knees, which may also affect the penetration of NPs. Indeed, elastomer resistance to organic solvents has been shown to be sensitive to small elongations (less than 20%) Citation19. In addition, prehension forces of up to 500 N have been induced by adult hands Citation20. Such a compression of the membrane may induce an effect similar to the application of a high pressure with the NPs in an aerosol, i.e. reduction of the barrier properties.
This paper reports some preliminary results on the penetration of NPs through protective clothing and gloves obtained with commercial 15 nm nTiO2 in powder and colloidal solutions. The tested protective clothing and glove materials consisted of nitrile and butyl rubber as well as of cotton/polyester weaves and polyolefin non-woven fabrics. Repeated biaxial deformations were applied to samples exposed to NPs in order to simulate typical use conditions of protective equipment under an occupational setting. Complementary analyses on the dynamic biaxial deformations and the contact of the protective materials with colloidal solutions of the NPs were also carried out.
2. Materials
2.1. Protective gloves and clothing
Two models of protective gloves, corresponding to two families of elastomers, were selected for this study: disposable nitrile rubber gloves (100 μm thick) and non-supported butyl rubber gloves (700 μ m thick). Samples were taken from the palm section of the gloves.
2.2. Nanoparticles
nTiO2 (Nanostructured & Amorphous Materials, Inc., Houston, TX) was been selected for this study due to its use in numerous applications (paints, cosmetics, electronic compounds, etc.) Citation3. nTiO2 is labelled as 99.7% pure anatase with an average particle size of 15 nm. Colloidal solutions of nTiO2 were also selected. Both were prepared from a 15 nm diameter anatase: a stock solution of 15 wt% in water (Nanostructured & Amorphous Materials, Inc., Houston, TX) and a stock solution of 20 wt% in 1,2-propanediol (MK Impex, Mississauga, ON).
2.3. Ultra-pure solvents
Solvents corresponding to the liquid carriers of the two colloidal solutions of nTiO2 were also tested for comparison purposes. Ultra-pure 1,2-propanediol (99.5 wt%) was obtained from Sigma Aldrich and high-performance liquid chromatography water was obtained from Acros Organics.
3. Methods
3.1. NP penetration experimental set-up
A test set-up has been developed for the purpose of this study Citation21 (). It includes an exposure chamber and a sampling chamber, which are separated from each other by the sample. Both chambers as well as all components in contact with NPs are made of ultrahigh molecular weight PE in order to limit the adsorption of nTiO2. The set-up has been designed to the exposure of glove samples to powders or colloidal solutions while simultaneously subjecting them to static or dynamic mechanical constraints. NPs are introduced in the exposure chamber and placed into contact with the external surface of the glove sample. In the case of powder NPs, a thin circular nitrile rubber membrane is placed on top of the sample to prevent the NP from being dispersed in the exposure chamber. As shown in , the test system is also equipped with a probe mounted on a pneumatic system for deforming the sample. The system is computer controlled and includes a 500 N load cell and a position detector. The whole set-up is enclosed in a glove box in order to ensure operator safety during assembly, dismounting and clean-up operations, as well as during tests.
The time profile of the applied sample deformations is illustrated in . They consist in the application of a 50% deformation every 5 min for the glove samples and a 6% deformation every 5 min for the textile materials. The probe head used in this study is shown in . It corresponds to a conical-spherical geometry that has been shown to successfully simulate biaxial deformations that are produced, for example, in gloves during hand flexion Citation22.
3.2. NP sampling protocol and detection methods
A sampling protocol was developed to collect NPs that crossed from the exposure chamber into the sampling chamber. Sample collection was facilitated by the use of a sampling solution, which was placed in the sampling chamber during the set-up. The sampling solution consisted of ultra-pure water acidified with 1% nitric acid Citation23. Before the beginning of each test, contamination of the sampling chamber by nTiO2 was verified by analysing a control sample that was produced by rinsing the sampling chamber. If a trace of contamination in the test control was measured, the result of the following test was discarded.
During the experiment, the maximum sample deformation height was such that no contact occurred between the sampling solution and the sample surface. Once the test was over and before dismounting the test setup, the chamber assembly was gently tilted and rotated so that the sampling solution could rinse the walls of the sampling chamber. The sampling solution was then transferred into a vial. Titanium concentrations in the sampling solution were analysed by inductively coupled plasma mass spectrometry (ICP–MS, Varian 820) for glove materials or by atomic absorption spectroscopy (Perkin Elmer AAnalyst 800) for protective clothing materials. Moreover, a small quantity of sampling solution was centrifuged on mica for qualitative analysis by Atomic Force Microscopy (Veeco-DI).
3.3. Quantification of characteristic features on the gloves surface
Some characteristic features can be observed on the gloves surface: micrometer-size pores for nitrile rubber and platelets for butyl rubber gloves Citation16. The surface morphology of five samples, for each elastomer, was analysed by SEM (Hitachi S3600N – Vacc=15 kV – magnification×300) and the quantification of the surface area of these features was performed using the software ImageJ (image processing).
3.4. Swelling measurements
Gravimetric measurements were performed on glove samples in order to evaluate the effect of the nTiO2 solutions and especially their liquid carriers on the glove materials. Indeed, elastomers may be sensitive to swelling in some solvents. Rectangular samples (4×50 mm) were taken from the palm section of the gloves. To obtain statistically significant data, triplicate measurements were performed for each solution. The measurements were performed by immersing the samples in the commercial nTiO2 colloidal solutions and in the ultra-pure solvents corresponding to the colloidal carriers. At regular intervals, samples were removed from the liquid, their surface gently blotted with a paper towel and their mass determined using a precision balance (±0.1 mg). The gain in mass was computed using Equation (1):
with Mt being the mass at time t and M0 the corresponding value before immersion.
4. Results and discussion
4.1. Characterization of the nTiO2
The size distribution of the nTiO2 was verified by statistical analysis of 174 particles imaged by transmission electron microscopy (TEM, JEM-2100F). Two allotropic forms of TiO2 were observed: a spherical anatase and a rod-like rutile (). Analysis by X-ray diffraction (Philips X'PERT) confirmed the presence of 3–6% rutile in the nTiO2. Moreover, these NPs seldom behaved as individual particles when in air Citation2. In fact, only two particles with a diameter lower than 20 nm were counted among the 174 particles. displays the size distribution of the analysed sample in terms of circular diameter, which is the diameter of a circle having the same surface as the particle Citation24. It can be seen that the average dimension of the particles/aggregates was situated around 100 nm, although even some micrometric-size agglomerates were recorded.
Analysis of the aqueous nTiO2 stock solution (following dilution to 10 mg/L) by fluorescence correlation spectroscopy gave a hydrodynamic diameter for the particles of 21±2 nm. The same analysis was not possible for the nTiO2 in 1,2-propanediol due to an incompatibility between the cell material and the liquid carrier. In addition, no characterization of the colloidal solutions was achievable by microscopy due to the formation of a μm-thick viscous film on the NPs following solvent evaporation. This film was attributed to the presence of additives in the colloidal solutions, which are used as stabilizing agents Citation25.
4.2. Characterization of the gloves and protective clothing
shows the micrometer-size pores which can be observed on the outer surface of the nitrile rubber glove. For its part, the outer surface of butyl rubber gloves shows platelets (). The same features were observed on the inner surface of both types of gloves. Two types of protective clothing were also studied: a 35/65 cotton/polyester plain woven fabric used to produce laboratory coats and a polyethylene–polypropylene (PE–PP) non-woven used for coveralls. and show, respectively, the outer surface of the PE–PP non-woven and the cotton/polyester woven materials. In both cases, the surfaces displayed porosities ranging from 1 to 10 μm for the PE–PP non-woven and from 10 to 100 μm for the cotton/polyester woven.
4.3. Protective glove materials and nTiO2 powder
Glove samples were exposed to the nTiO2 powder using a dynamic exposure (one biaxial deformation every 5 min for up to 7 h). Control experiments were carried out under identical conditions except that no NP was introduced into the exposure chamber. Controls ensured that the presence of titanium in the sampling solutions was related to the passing of NPs through the materials rather than simply a contamination of the sampling solution by the materials. No significant differences were observed for measurements performed with and without NPs when the butyl rubber was tested (). This result indicated that the butyl rubber was not permeable to the nTiO2 powder, even after 7 h of 50% deformations to the glove material.
Figure 6. Variation in concentration of titanium in the sampling solutions as a function of the duration of the 50% biaxial deformations for nitrile (nitrile butadiene rubber (NBR)) and butyl (isobutylene isoprene rubber (IIR)) rubber samples exposed to nTiO2 powder.
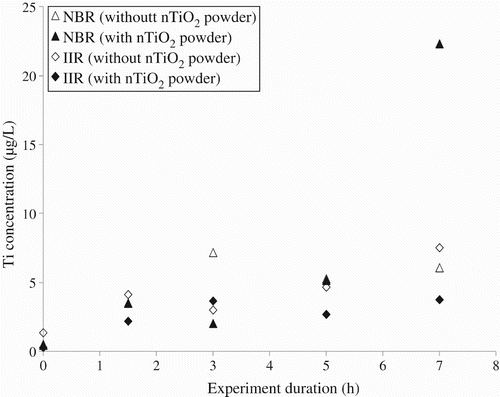
On the other hand, a much higher concentration in titanium was recorded in the sampling solution after 7 h of dynamic deformations for nitrile rubber gloves. Based upon its thermodynamic constants, TiO2 is unlikely to dissolve significantly and thus the ICP–MS results suggest that nTiO2 was penetrating the nitrile rubber gloves. The presence of NPs nTiO2was confirmed by atomic force microscopy (). These results indicated the passage of nTiO2 through the nitrile gloves after dynamic deformations of more than 5 h.
Figure 7. (a) Atomic force microscopy pictures of native mica substrate and (b) after centrifugation of the sampling solution for the 7 h dynamic biaxial deformations/nTiO2 powder/nitrile rubber condition.
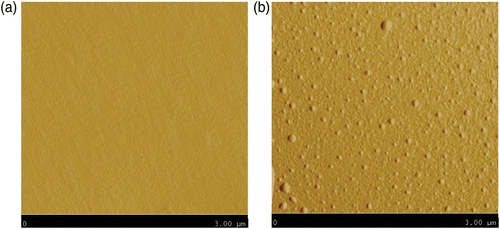
In order to find explanations for the possible passage of the NPs through the nitrile rubber gloves, an analysis of the effect of repeated mechanical deformations on the glove surface morphology was carried out. For that purpose, the variation in the surface features identified for each elastomer (pores for nitrile rubber and platelets for the butyl rubber, see ) was determined as a function of time. Dynamic biaxial deformations were applied to the glove samples for up to 7 h using the test set-up described in Section 3.1. The surfaces of the nitrile and butyl rubber samples after 7 h of dynamic biaxial deformations are given in . In comparison with corresponding pictures for the non-deformed samples (), a significant change in the surface was apparent in the nitrile rubber samples following 7 h of dynamic deformations. In particular, the number, diameter and depth of the pores appear to be largely increased, possibly indicating a weakening of the membrane. It is possible to speculate that NPs may have accumulated in the deeper imperfections.
Figure 8. (a) SEM images of the nitrile rubber and (b) butyl rubber external glove surface after 7 h of dynamic biaxial deformations.
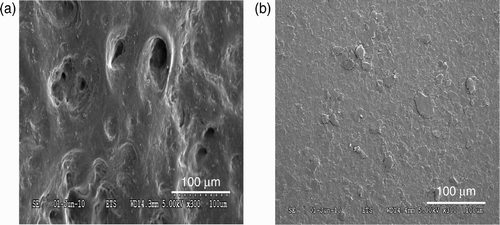
displays the variation in these surface features as a function of the duration of the applied deformations. A very slow increase can be seen for both elastomers during the first 5 h of deformations. The deterioration of the materials could be attributed to abrasion of the sample surface by the probe. This abrasion phenomenon might possibly be amplified in the presence of NPs. Indeed, TiO2 particles, which are much harder than the elastomer, could act as an abrasive powder. It is especially interesting to note that the nitrile rubber samples experienced a sharp increase in the surface area of pores after 7 h of repeated deformations. Such an observation could explain the jump in the Ti concentration in the sampling solution that was measured for the nitrile rubber after these longer times.
Figure 9. Variation of the surface features as a function of the duration of dynamic biaxial deformations for the nitrile and butyl rubber (outer surface).
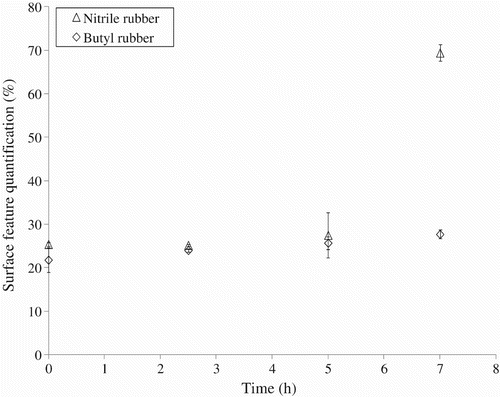
The effect of the repeated deformations on the agglomeration of the nTiO2 powder was investigated. In this case, the sample surface was analysed by a field emission gun SEM (FEG–SEM, JEOL JSM-7600F). A reduction in the agglomeration state of the NPs was observed. For example, as shown in , agglomerates (about 500 nm diameter) can be seen after 1.5 h of dynamic biaxial deformations. On the other hand, only aggregates in the range of 100 nm can be observed after 7 h of deformations (). This reduction in the agglomeration state can be attributed to shearing induced by the probe on the sample and may potentially contribute to increased penetration of the nTiO2 particles through the protective gloves.
4.4. Protective glove materials and nTiO2 in colloidal solutions
Tests were also carried out by exposing the glove samples to a solution of nTiO2 for up to 7 h of 50% dynamic biaxial deformations. As above, the sampling solutions were analysed by ICP–MS () and as above, no differences were observed for measurements made with and without the NPs for the samples composed of the butyl rubber. On the other hand, for the nitrile gloves, a clear increase in the concentration of titanium was observed in the sampling solutions after 5 and 7 h of deformations nTiO2. These results suggest the penetration of the nTiO2 solutions nTiO2 through the nitrile gloves when they were subjected to 50% dynamic biaxial deformations for periods of 5 h or more.
Figure 11. Variation in the concentration of titanium in the sampling solutions as a function of the duration of 50% biaxial deformations for nitrile (NBR) and butyl (IIR) rubber samples exposed to colloidal solutions of nTiO2.
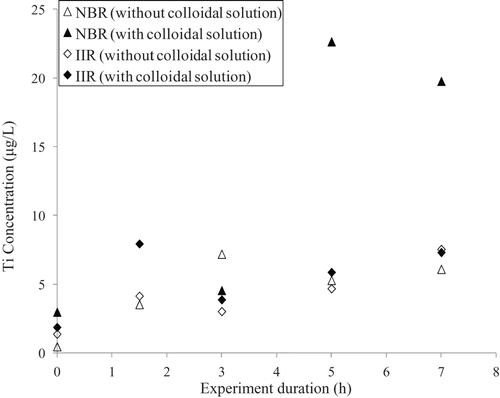
In order to investigate the cause of the apparent NP penetration through the nitrile gloves, the glove surface morphology was characterized following the mechanical deformations and contact with the solutions of nTiO2. Dynamic biaxial deformations were applied to the glove samples over 7 h and in combination with the nTiO2 solvents. The surface morphology of the glove samples following exposure to the solvents, to the mechanical deformations or to a combination of the two was imaged by SEM and analysed by Image J. Contact with 1,2-propanediol alone did not appear to cause any modification to the nitrile rubber surface while a small effect was produced on the butyl rubber (). In contrast, when combined with mechanical deformations, the presence of 1,2-propanediol reduced the number of glove deformations, probably due to its role as a lubricating agent. These data did not provide any insight into the nTiO2 large increase in titanium that was observed in the nitrile rubber sampling solutions after 5 and 7 h.
Figure 12. Effect of 7 h of exposure to 1,2-propanediol (propylene glycol (PG)), dynamic biaxial deformations and combination of both on the material-specific glove surface features for the nitrile and butyl rubber.

Swelling measurements were performed by recording the mass of the samples after immersion in the nTiO2 solutions. The results obtained for the nitrile rubber immersed in the solutions of nTiO2 are presented in . A gradual weight increase is observed for the two colloidal solutions, indicating significant penetration of both solutions of nTiO2. Nonetheless, a larger swelling ratio was recorded for the aqueous solution of nTiO2. For example, for an immersion time of 8 h, the swelling ratio for the nitrile rubber was 35% for the nTiO2 in water and 15% when they were in nTiO2 1,2-propanediol. It should be noted that maximum was not attained for either of the solutions of nTiO2 after 8 h of immersion.
Figure 13. Mass gain of the nitrile rubber gloves as a function of immersion time in the nTiO2 solution in water and 1,2-propanediol (PG).
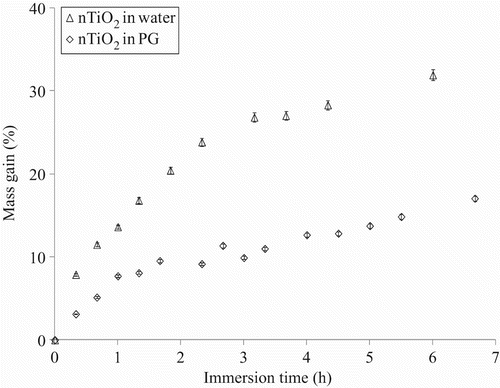
A comparison of the swelling behaviour of the two glove materials nTiO2 was therefore performed over a longer period (). A large difference in the swelling behaviour was observed for the two elastomers. A 79% gain in mass was observed for the nitrile rubber after three days of immersion with a plateau after about 40 h of immersion. For the butyl rubber, no significant weight gain was recorded. This difference in behaviour between the two elastomers can be attributed to their respective affinity to the liquid carriers. In particular, the butyl rubber displays an excellent resistance to most polar solvents Citation26. The difference in swelling behaviour between the nitrile and butyl rubber may well be one of the primary reasons that the nTiO2 was able to more easily penetrate the nitrile rubber gloves, especially when the NPs were in water.
4.5. Protective clothing materials and nTiO2 in powder
Some preliminary measurements were carried out to investigate the penetration of nTiO2 powder through protective clothing following a slight adjustment of the test conditions. In particular, since the maximum deformation of the PE–PP non-woven is 10% and the cotton/polyester plain woven fabric is 25%, and since these materials are usually only subjected to small deformations when they are used in protective clothing, dynamic biaxial deformations were limited to 6%.
As illustrated in , the presence of NPs in the sampling solutions was detected after 7 h of deformations for the non-woven coverall material. In this case, the concentration of titanium that was detected reached 2400 μg/L. For the laboratory coat fabric, a gradual increase in the concentration of titanium was observed with a large jump after 5 h and a maximum concentration in titanium in the sampling solution was 220 μg/L (). By comparison, no titanium was detected in the sampling solution when NPs were not present in the exposure chamber. These results suggest the passage of nTiO2 powder through the protective clothing textiles in the presence of dynamic deformations. The higher level of protection that was measured for the non-woven material as compared with the woven fabric is in agreement with what has been reported by Golanski et al. Citation10 when studying graphite nanoaerosols. The sharp increase in the concentration of titanium in the sampling solutions that was observed for both materials after 7 h of deformations might have been caused by the mechanical failure of the membranes at the micron or submicron scale. In this case, no observations of the sample surface were performed and more work is clearly required in order to identify the underlying phenomena responsible for the loss of integrity of the protective materials.
5. Conclusion
This paper has investigated the penetration of nTiO2 powder and two commercially obtained nTiO2 solutions through protective clothing and gloves that were subjected to dynamic biaxial deformations, corresponding to conditions typical of occupational use. In the case of the protective glove materials, no penetration of NPs was measured through the butyl rubber, even after 7 h of deformations. On the other hand, penetration of nTiO2 was recorded for the disposable nitrile gloves when exposed to both nTiO2 powder and colloidal solutions of nTiO2 (after 7 and 5 h, respectively). Similarly, penetration of the nTiO2 powder was detected for two typical fabrics of laboratory coats, when mechanical deformations simulating occupational use were included in the simulation.
This penetration of the NPs through the protective materials was attributed in part to a degradation of their physical and mechanical properties following repetitive mechanical deformations and contact with nTiO2 liquid carriers. A possible reduction in the agglomeration state of the nTiO2 powder as a result of probe shearing was also observed. These results show that great care must be exercised when selecting protective clothing and gloves for the handling of NPs. In particular, these results suggest that exposure may be reduced through the frequent replacement of disposable gloves, especially when exposure of the NPs occurs in the liquid phase. In addition, while non-woven coveralls appear to be preferable to woven fabric laboratory coats, their prolonged use or reuse should be avoided.
Acknowledgements
This project was conducted within the context of the Research Chair in Protective Materials and Equipment for Occupational Safety and Health (École de technologie supérieure). It has been supported by the Institut Robert-Sauvé en santé et en sécurité du travail (IRSST), NanoQuébec, the Natural Sciences and Engineering Research Council of Canada (NSERC), the Agence nationale de sécurité sanitaire de l'alimentation, de l'environnement et du travail (ANSES) and the Agence de l'Environnement et de la Maıtrise de l’Énergie (ADEME). The authors would like to acknowledge the contribution of S. Mahé, A. Jambou and F. Deltombe (École de technologie supérieure) to the project as well as the collaboration of P. Plamondon and G. L'Espérance (École Polytechnique de Montréal).
References
- Roco M CMirkin C A, Hersam M C. Nanotechnology research directions for societal needs in 2020 – retrospective and outlook Berlin and Boston: WTEC Study on Nanotechnology Research Directions; 2010
- Hervé-Bazin B. Les nanoparticules: un enjeu majeur pour la santé au travail? Les Ulis Cedex A France: EDP Science20071936
- Robichaud C O, Uyar A E, Darby M R, Zucker L G, Wiesner M R. Estimates of upper bounds and trends in nano-TiO2 production as a basis for exposure assessmentEnviron Sci Technol. 2009;43:4227–4233 (doi:10.1021/es8032549) doi: 10.1021/es8032549
- CCHST. Information de base sur le dioxyde de titane. Centre canadien d'hygiène et de sécurité au travail; 2007. Available from: http://www.cchst.ca/
- Höhr D, Steinfartz Y, Schins RP F, Knaapen A M, Martra G, Fubini B, Borm PJ A. The surface area rather than the surface coating determines the acute inflammatory response after instillation of fine and ultrafine TiO2 in the ratInt J Hyg Environ Health. 2002;205(3):239–244. doi: 10.1078/1438-4639-00123
- Warheit D B, Webb T R, Reed K L, Frerichs S, Sayes C M. Pulmonary toxicity study in rats with three forms of ultrafine-TiO2 particles: differential responses related to surface propertiesToxicology. 2007;230(1):90–104. doi: 10.1016/j.tox.2006.11.002
- Warheit D B, Webb T R, Sayes C M, Colvin V L, Reed K L. Pulmonary instillation studies with nanoscale TiO2 rods and dots in rats: toxicity is not dependent upon particle size and surface areaToxicol Sci. 2006;91(1):227–236. doi: 10.1093/toxsci/kfj140
- IARC. Monographs on the evaluation of carcinogenic risks to humans – carbon black, titanium dioxide and talc Lyon: World health organization; 2010
- pOECD. Comparison of guidance on selection of ski protective equipment and respirators for use in the workplace: manufactured nanomaterials. Series on the safety of manufactured nanomaterials. 2009; 12. (Report number: JT03267010)
- Golanski L, Guiot A, Tardif F. Are conventional protective devices such as fibrous filter media, respirator cartridges, protective clothing and gloves also efficient for nanoaerosols? NanoSafe: European Strategy for nanosafety; 2008. Available from: http://www.nanosafe.org/home/liblocal/docs/Dissemination%20report/DR1_s.pdf
- Golanski L, Guiot A, Rouillon F, Pocachard J, Tardif F. Experimental evaluation of personal protection devices against graphite nanoaerosols: fibrous filter media, masks, protective clothing, and glovesHum Exp Toxicol. 2009;28(6–7):353–359. doi: 10.1177/0960327109105157
- Golanski L, Guoit A, Tardif F. Experimental evaluation of individual protection devices against different types of nanoaerosols: graphite, TiO2 and PtJ Phys Conf Ser. 2009;170(1):012025. doi: 10.1088/1742-6596/170/1/012025
- Hanley J T (Vêtements de protection) Aerosol system and swatch testing of chemical protective garments Elevated Wind Studies International Conference Arlington, VA;2006151
- Huang S-H, Chen C-W, Chang C-P, Lai C-Y, Chen C-C. Penetration of 4.5 nm to 10 nm aerosol particles through fibrous filtersJ Aerosol Sci. 2007;38(7):719–727. doi: 10.1016/j.jaerosci.2007.05.007
- Hofacre K C. (Textile) Aerosol penetration of fabric swatchesElevated Wind Studies International Conference Arlington, VA;: 2006150
- Ahn K, Ellenbecker MJ. Dermal and respiratory protection in handling nanomaterials at the center for high-rate nanomanufacturing (CHN). Paper presented at: The AIHce Conference, Chicago, IL, 2006 May 13–18.
- Nohile C, Dolez P I, Vu-Khanh T. Parameters controlling the swelling of butyl rubber by solventsJ Appl Polym Sci. 2008;110(6):3926–3933 (doi:10.1002/app.29004) doi: 10.1002/app.29004
- Vinches L, Dolez P, Vu-Khanh T. Study on the penetration of TiO2 nanoparticles through nitrile and butyl protective gloves. Paper presented at: The International Conference on Nanotechnology: Fundamentals and Applications, Ottawa; 2011 juillet 27–29.
- Li Y, De Kee D, Fong C FCM, Pintauro P, Burczyk A.. Influence of external stress on the barrier properties of rubbersJ Appl Polym Sci. 1999;74(6):1584–1595. doi:10.1002 doi: 10.1002/(SICI)1097-4628(19991107)74:6<1584::AID-APP34>3.0.CO;2-L
- Nicolay C W, Walker A L. Grip strength and endurance: influences of anthropometric variation, hand dominance, and genderInt J Ind Ergonomics. 2005;35(7):605–618. doi: 10.1016/j.ergon.2005.01.007
- Dolez P, Vinches L, Wilkinson K, Plamondon P, Vu-Khanh T. Development of a test method for protective gloves against nanoparticles in conditions simulating occupational useJ Phy Conf Ser. 2011;304(1):012066 (doi:10.1088/1742-6596/304/1/012066) doi: 10.1088/1742-6596/304/1/012066
- Harrabi L, Dolez P I, Vu-Khanh T, Lara J. Evaluation of the flexibility of protective glovesInt J Occup Saf Ergonomics. 2008;14(1):61–68
- Kaegi R, Ulrich A, Sinnet B, Vonbank R, Wichser A, Zuleeg S, Simmler H, Brunner S, Vonmont H, Burkhardt M, Boller M. Synthetic TiO2 nanoparticle emission from exterior facades into the aquatic environmentEnviron Pollut. 2008;156:233–239 (doi:10.1016/j.envpol.2008.08.004) doi: 10.1016/j.envpol.2008.08.004
- Zayed J, L'Espérance G, Truchon G, Cloutier Y, Kennedy G. Contribution de la nanoscopie à l’échantillonnage et caractérisation physico-chimique des nanoparticules. Montréal: Institut de recherche Robert-Sauvé en santé et sécurité du travail; 2009.
- Dolez P, Vinches L, Perron G, Vu-Khanh T, Plamondon P, L'Espérance G, Wilkinson KJ, Cloutier Y, Dion C, Truchon G. Développement d'une méthode de mesure de la pénétration des nanoparticules à travers les matériaux de gants de protection dans des conditions simulant l'utilisation en milieu de travail. Montréal: Institut de recherche Robert-Sauvé en Santé et Sécurité du Travail; 2012.
- Jin J Z, Nguyen V, Gu W Q, Lu X Y, Elliott B J, Gin D L. Cross-linked lyotropic liquid crystal-butyl rubber composites: promising ‘breathable’ barrier materials for chemical protection applicationsChem Mater. 2005;17(2):224–226. doi: 10.1021/cm040342u