ABSTRACT
Poplar stands as one of the primary afforestation trees globally. We successfully generated transgenic poplar trees characterized by enhanced biomass under identical nutrient conditions, through the overexpression of the pivotal nitrogen assimilation gene, pxAlaAT3. An environmental risk assessment was conducted for investigate the potential changes in rhizosphere soil associated with these overexpressing lines (OL). The results show that acid phosphatase activity was significantly altered under ammonium in OL compared to the wild-type control (WT), and a similar difference was observed for protease under nitrate. 16SrDNA sequencing indicated no significant divergence in rhizosphere soil microbial community diversity between WT and OL. Metabolomics analysis revealed that the OL caused minimal alterations in the metabolites of the rhizosphere soil, posing no potential harm to the environment. With these findings in mind, we anticipate that overexpressed plants will not adversely impact the surrounding soil environment.
1. Introduction
The widespread adoption of genetically modified (GM) crops has led to substantial economic and societal benefits, with the majority of cotton (79%) and soybean (74%) currently produced worldwide being genetically modified.Citation1,Citation2 The cultivation of GM crops is considered a vital strategy for addressing the challenges related to food scarcity, climate change, sustainable development, and environmental conservation. However, the biosafety of GM crop cultivation and application has sparked an ongoing debate.
The introduction of GM plants has raised biosafety concerns, both for human health and the environment. Early environmental risk assessments of transgenic plants primarily focused on their impact on the aboveground ecosystem components. More recently, the analysis of the effects of transgenic plant cultivation on soil has become an integral component of the comprehensive safety assessment of transgenic plants.Citation1 Likely due to the influence of variable biotic and abiotic factors, the impact of GM plants on soil microorganisms is still heavily debated among researchers and no consensus has been reachedCitation3; existing studies have yielded conflicting outcomes. Some studies suggest that transgenic plants cause a reduction of soil microbial diversity,Citation3,Citation4 whereas an increasing body of research has demonstrated that cultivating GM plants has either minimal or no impact on soil microbial communities.Citation3,Citation5,Citation6 Nevertheless, several reports have highlighted the negative effects of transgenic plants on soil physicochemical properties, enzymatic activity, and microbial diversity.Citation7–9 Given the absence of a universal standard for evaluating the influence of transgenic plants on soil parameters and microbial diversity, assessing the risk of planting transgenic plants in soil remains a challenging task. Currently, the most effective approach for assessing the outcomes of using GM crops is to employ plant- and gene-specific methodologies.Citation10
Poplar, a deciduous tree of the Salicaceae family, serves as a model woody plant and is one of the world’s most important forest tree species. Possessing attributes of rapid growth and robust regenerative capabilities, it occupies a pivotal role in global tree cultivation.Citation11 Since Parsons et al.. (1986)Citation12 first confirmed that poplar can undergo genetic transformation and express foreign genes, this technology has been used to enhance various important traits, including insect resistance, cold tolerance, drought resilience, salt tolerance, and wood quality.Citation13–16 Poplar became the most widely genetically modified woody plant globally, and multiple transgenic poplar lines have been created with outstanding traits. Studies have demonstrated the significant role of the AlaAT gene, a pivotal gene in nitrogen metabolism, in enhancing plant resistance and augmenting biomass production.Citation17,Citation18 Overexpression of the barley AlaAT gene led to a substantial increase in grain yield and biomass in both canolaCitation19 and rice.Citation20 Additionally, sugarcane exhibited improved nitrogen use efficiency under low nitrogen conditions upon overexpression of this gene.Citation21 Moreover, overexpression of AlaAT genes from other species in Arabidopsis thaliana has been shown to enhance nitrogen use efficiency.Citation22 However, it remains unclear whether overexpression of the AlaAT gene would induce changes in poplar biomass.
Recently, we successfully cloned the full-length sequence of the Populus × xiaohei pivotal nitrogen assimilation gene pxAlaAT3, coding an alanine aminotransferase. Using Agrobacterium-mediated transformation, we effectively introduced pxAlaAT3 into P. × xiaohei and obtained plants with high expression levels of the transgene. In this study, we assessed the biomass of these transgenic plants. More importantly, we evaluated the ecological risks of overexpressing the pxAlaAT3 gene in P. × xiaohei by examining its impact on rhizosphere soil supplemented with two forms of nitrogen. We grew P. × xiaohei overexpressing pxAlaAT3 and wild-type plants under controlled greenhouse conditions and analyzed the changes in soil chemistry, enzyme activity, bacterial communities, and metabolites. The results of this study will establish a crucial theoretical foundation for subsequent field experiments with transgenic P. × xiaohei and also serve as a reference for future assessment of the ecological safety of GM poplar plant lines.
2. Materials and Methods
2.1. Experimental Material and Sample Collection
The seeds of P. × xiaohei wild-type (WT) and the pxAlaAT3 overexpressing line (OL) used in this study were obtained from the State Key Laboratory of Forest Tree Genetics and Breeding at Northeast Forestry University in Harbin, China. Seedlings were cultivated in pots with Farag ova a mixture of humus black soil:vermiculite:perlite (6:3:1), and their rhizosphere soil was collected and analyzed. The flower pots used were 30 cm in diameter and were placed in a growth chamber under controlled conditions (temperature of 25 ± 2°C, with a 16-hour light/8-hour dark cycle, and a relative humidity maintained at 65%–75%). The nitrogen-free Long-Ashton (LA) liquid medium nutrient solution (Hewitt, 1966)Citation23 was supplemented with 3 mM of either ammonium nitrogen (NH4Cl) or nitrate nitrogen (NaNO3) and applied to the pots every three days until day 180 of growth when sampling was conducted. For sample collection, after plants were retrieved from pots, large soil clumps were removed from the root systems, and roots were shaken to dislodge loosely attached soil; further soil was collected from roots using a sterile brush. Soil samples obtained from nine plants were combined in equal proportions, flash-frozen in liquid nitrogen, and stored at −80°C for further analysis.
P. × xiaohei saplings, cultivated for 180 days, were removed from the growth medium. Surrounding medium was carefully cleaned from the roots, followed by rinsing with running water. Surface water traces were wiped away using absorbent paper, and nitrogen is used to blow away the surface liquid (5 seconds at room temperature). The fresh weight was then measured. The weighed root system was placed in an oven at 105°C for 2 hours, followed by drying at 80°C until a constant weight was achieved. The dry weight of the sample was subsequently measured.
2.2. Analysis of Rhizosphere Soil Nutrient Contents
The methods used to assess the rhizosphere soil available nutrient contents were: the alkali diffusion method for the available nitrogen (AN), the molybdenum-antimony resistivity colorimetric method for the available phosphorus (AP), and flame photometry for the available potassium (AK). Additionally, total soil nutrient contents were determined using the Kjeldahl method for total nitrogen (TN), the molybdenum-antimony resistance colorimetric method for total phosphorus (TP), and flame photometry for total potassium (TK).Citation24 The method described by Walkley and Black method (1934) was used for the determination of soil organic carbon (SOC).Citation25
2.3. Analysis of Rhizosphere Soil Enzymatic Activity
The rhizosphere soil activities of acid phosphatase (S-ACP, BC0285), nitrate reductase (S-NR, BC3105), and sucrase (S-SC, BC0245) were quantified using the corresponding kits obtained from the Solarbio Corporation, China. Additionally, kits for measuring the activity of soil urease (S-UE, SUE-2-Y), catalase (S-CAT, SCAT-2-Y), neutral protease (S-NPT, SNPT-1-Y), amylase (SA, SDM-2-G), neutral phosphatase (S-NP, SNP-2-W), and dehydrogenase (SDHA, SDHA-2-G) were obtained from the Suzhou Kemin Biotechnology Co., Ltd., China.
2.4. Detection of Microbial Diversity in Rhizosphere Soil
DNA extraction from soil samples was performed using the FastPure Soil DNA Isolation Kit (Shanghai Major Yuhua Biomedical Technology joint stock company). The bacterial 16S rRNA genes were amplified following the method described in Huse et al. (2008).Citation26 The amplified sub-library was subsequently sequenced by Majorbio Biomedical Technology Co., Ltd. (Shanghai, China) using the Illumina MiSeq PE300 platform. Demultiplexing of the original FASTQ files obtained was done with an in-house perl script, followed by quality filtering using fastp (version 0.19.6Citation27 and merging using FLASH (version 1.2.1.Citation28 UPARSE (version 11Citation29 was used for performing operational taxonomic unit (OTU) clustering at a 97% similarity threshold. Taxonomic classification was performed using the bacterial SILVA reference database (v138). Bioinformatics analyzes, including α-diversity index analysis, NMDS, PLS-DA, average linkage clustering, Wilcoxon rank-sum test, and LefSe analysis, were conducted on the Majorbio cloud platform.
2.5. Analysis of Rhizosphere Soil Metabolite Changes
Metabolite analyzes were conducted using 1 g of the collected rhizosphere soil following the sample pretreatment protocol described in Qi et al.. (2022).Citation30 LC-MS/MS analyses were performed on a UHPLC system (1290, Agilent Technologies, USA) coupled to a TripleTOF 6600 system (QTOF, AB Sciex) operating in EI mode; three biological replicates were performed. The settings and other experimental details were previously described.Citation31,Citation32 Significant differences in metabolites were investigated by calculating variable importance in projection (VIP) values in the orthogonal partial least squares discriminant analysis (OPLS-DA) model. The variables were evaluated for significance with the Student’s t-test, and significant differences between the two control groups (VIP>1 and p < 0.05) were retained.Citation33 Metabolites with substantial contributions to the rhizosphere soil were identified using the random forest approach; CCA and RDA analyzes were performed to explore the relationship between microorganisms present and changes in metabolite concentrations.
2.6. Statistical Analysis and Data Visualization
Quantitative data were presented as mean ± SD. Statistical differences between experimental groups were determined with Student’s t-tests with a significance threshold set at p < .05. Statistical analyzes were conducted using SPSS (version 19.0; SPSS Inc., Chicago, IL), and figures were generated using GraphPad Prism (version 8.0.1, GraphPad, USA). Circular heatmaps were produced using Chiplit online tools.
3. Results
3.1. Variations in Root Weight Among Treatment Groups
Root dry and fresh weights were assessed for four treatment groups: WT+NH4+, WT+NO3−, OL+NH4+, OL+NO3−. The results () reveal that under OL+NH4+ treatment, the average root fresh weight was 12.84 g, a significant 39.9% increase compared to WT+NH4+. Similarly, OL+ NO3− exhibited a root fresh weight of 11.53 g, marking a 40.9% increase over WT+ NO3−. Dry weights followed a similar trend, with OL+NH4+ showing a 20.6% increase compared to WT+ NH4+, and OL+ NO3− only a 9.95% increase compared to WT+ NO3−.
3.2. Analysis of Rhizosphere Soil Nutrients
The results of the rhizosphere soil nutrient analysis for WT and OL plants are shown in . The AP content was similar in the rhizosphere soil of WT (31.3 mg/kg) and OL (33.6 mg/kg) subjected to the ammonium nitrogen treatment, with the difference between the two groups not being statistically significant. Similarly, under nitrate nitrogen treatment, the AP content was not significantly different between OL (22.97 mg/kg) and WT (23.3 mg/kg) groups. The TN, TP, TK, AN, AK, SOC, C:N and PH were similar between the rhizosphere soil of the two lines when grown under the same nitrogen treatments. However, there were significant differences between the two groups for TN, AN, AP, SOC, C:N and PH assessed under different nitrogen treatments.
Figure 2. Chemical properties of rhizosphere soil of P. × xiaohei wild-type (WT) and the pxAlaat3 overexpressing line (OL) supplemented with nitrate nitrogen or ammonium nitrogen. (a) Available phosphorus (AP), (b) Total phosphorus (TP), (c) Available potassium (AK), (d) Total potassium (TK), (e) Available nitrogen (AN), (f) Total nitrogen (TN), (g) Soil organic carbon (SOC), (h) C:N, (i) PH.
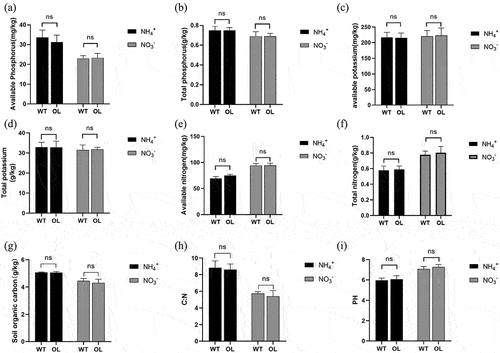
3.3. Rhizosphere Soil Enzymatic Activity
The activities of nine rhizosphere soil enzymes of WT and OL plants are reported in . Notably, under ammonium nitrogen treatments, the activity of acid phosphatase was significantly different between OL and WT plants, while protease activity was changed under nitrate nitrogen treatments. It is important to note that the activities of dehydrogenase, catalase, sucrase, amylase, neutral phosphatase, nitrate reductase, and urease all remained largely unchanged between the two experimental lines under the same nitrogen treatments.
Figure 3. Changes in enzymatic activity in rhizosphere soil of P. × xiaohei wild-type (WT) and the pxAlaat3 overexpressing line (OL) supplemented with nitrate nitrogen or ammonium nitrogen. (a) catalase, (B) acid phosphatase, (C) soil urease, (D) sucrase, (E) neutral protease, (F) amylase, (G) neutral phosphatase, (H) dehydrogenase, (I) nitrate reductase.
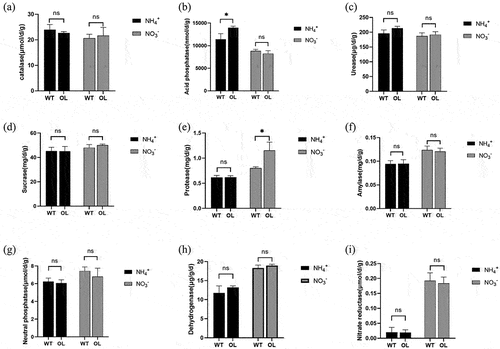
3.4. Rhizosphere Soil Microbial Diversity
shows the results of the α-diversity index analysis. The Chao index indicated no significant differences between the rhizosphere soil microbial diversity of WT and OL groups under either ammonium or nitrate nitrogen treatments (). The same results were obtained with analysis of the Coverage index (). The coverage of the measured microorganisms exceeded 0.986 in all cases, indicating that the sequencing results from all four experimental groups accurately represent the real conditions of the samples. The Shannon and Simpson indices also indicated no significant differences in microbial community diversity between the two lines under the same form of nitrogen treatment. However, we observed a significant difference in the microbial community of OL rhizosphere soil between the two nitrogen treatment samples.
Figure 4. α-diversity index on OUT level measurement of P. × xiaohei wild-type (WT) and the pxAlaat3 overexpressing line (OL) supplemented with nitrate nitrogen or ammonium nitrogen. (a) Chao index, (b) Coverage index, (c) Shannon index, (d) Simpson index.
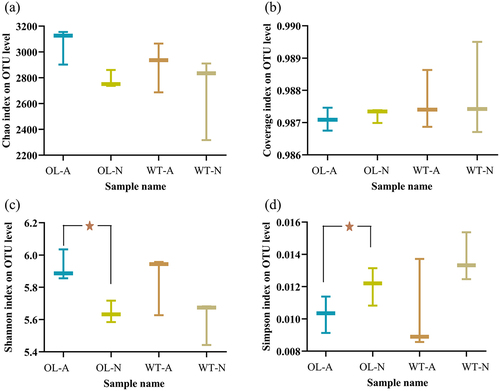
The OTU results for the four experimental groups are presented in . Specifically, 4364 OTUs were identified in OL-A (ammonium nitrogen), 3749 OTUs in OL-N (nitrate nitrogen), 4157 OTUs in WT-A, and 3695 OTUs in WT-N. Notably, 1000 OTUs were unique to the OL-A group, while 892 were unique to WT-A. OL-N and WT-N had 664 and 594 unique OTUs, respectively (). shows the outcomes of the average-linkage clustering analysis. Notably, the samples from the WT-N closely clustered with those from the OL-N, while WT-A samples clustered closely to those of the OL-A group. This finding aligns with the NMDS result (), where a stress value of 0.06 indicates a reliable outcome. Furthermore, the PLS-DA results indicate that the samples from the two nitrate nitrogen groups, OL-N and WT-N, are closely associated and cannot be distinguished. On the horizontal coordinate, the difference between OL-A and WT-A is not substantial, with a noticeable separation primarily evident on the vertical coordinate ().
Figure 5. Number of operational taxonomic units (OTUs) and comparison between experimental groups. (a) Number of OTUs; (B) Sample level clustering results; (C) NMDS scatter plot; (D) orthogonal partial least squares discriminant analysis (OPLS-DA) results.
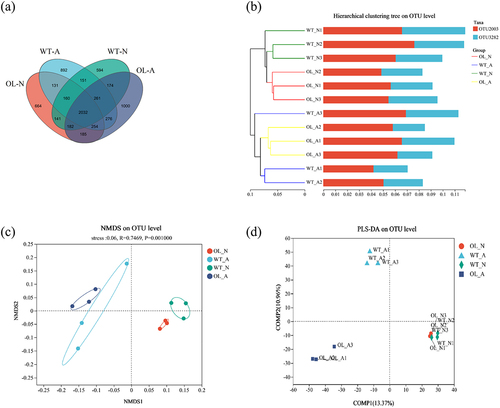
The results of the community composition analysis are shown in , with 6A illustrating the gate level composition. The top 10 dominant bacterial groups identified at the phylum level were Proteobacteria, Actinobacteriota, Acidobacteriota, Gemmatimonadota, Chloroflexi, Patescibcteria, Chloroflexi, Acidobacteriota, Firmicutes, Bacteroidota, Myxococcota, and Cyanobacteria. Notably, the proportion of these 10 dominant phyla varied among the four experimental groups. At the genus level, the top 10 dominant bacterial groups identified, labeled 1–10, were norank_f__norank_o__Gaiellales, Sphingomonas, Pseudolabrys, norank_f__Gemmatimonadaceae, Rhodanobacter, Gemmatimonas, norank_f__WWH38, norank_f__Micropepsaceae, Arthrobacter, and norank_f__norank_o__norank_c__KD4–96 (). The proportion of these 10 dominant bacterial genera across the four groups also varied. Furthermore, the Wilcoxon rank-sum test () indicated that the differences between OL-N and WT-N, as well as between WT-A and OL-A, were not statistically significant. This suggests that under identical nitrogen conditions, differences in the root microbial flora at the phylum and genus levels between OL and WT plants were not significant.
Figure 6. Community composition and inter-group species differences. Results of the proportion of dominant bacterial communities at the phylum (A) and genus (B) levels. Genus level Wilcoxon Rank-Sum Test analysis results under ammonium (C) or nitrate (D) treatment.
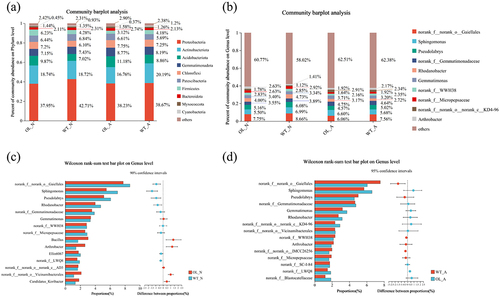
LefSe analysis revealed differences in the abundance and quantity of rhizosphere microorganisms between OL and WT plants at various taxonomic levels under the same nitrogen treatment ( and S1–S4). The red and blue nodes in and S1 illustrate population differences between OL-N and WT-N in 112 taxa; for WT-A and OL-A, 196 taxa were changed ( and Fig S2). At the genus level, 42 and 82 taxa were significantly different between OL-N and WT-N and WT-A and OL-A, respectively. The linear discriminant analysis (LDA) scores for microbial groups with significantly different numbers in OL-N vs. WT-N ( and S3) and WT-A vs. OL-A ( and S4) show that p_Acidobacteriota had the most pronounced differential effect in OL-N, while p_Proteobacteria and c_Alphaproteobacteria had the largest differential effect in WT-N. Furthermore, c_Thermoleophilia and p_Actinobacteriota had the highest differential effect in WT-A. Notably, the LDA SCORE(LOG10) of the OL-A group was lower than that of other groups.
Figure 7. Results of the LefSe analysis. The cladogram shows the microbial species present in significantly different numbers in the WT-N (wild type with nitrate nitrogen) vs. OL-N (pxAlaat3 overexpressing line with nitrate nitrogen) group (a) and the WT-A (wild type and ammonium) vs. OL-A group (pxAlaat3 overexpressing lines with ammonium) (b), (c) WT-N vs. OL-N LDA scores (d) WT-A vs. OL-A LDA scores.
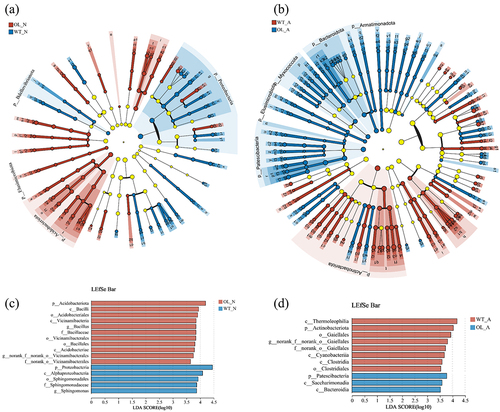
3.5. Rhizosphere Soil Metabolite Analysis
Changes in rhizosphere soil metabolites were evaluated using a broad metabolome analysis to compare differences between WT and OL plants (). OPLS-DA of differential metabolites showed that OL-N and WT-N were relatively close, while there were slight differences in the transverse and longitudinal coordinates between OL-A and WT-A (). The analysis also revealed that only a small number of metabolites were differently changed between the OL and WT experimental groups. Specifically, there were 14 metabolites significantly increased and 7 significantly decreased in OL-A compared to WT-A. For WT-N and OL-N, 15 metabolites were significantly increased and 11 significantly decreased in the WT group (). The expression patterns of all differential metabolites are depicted in , while their positions within biological pathways are shown in . Notably, these differential metabolites mostly consist of primary metabolites, such as organic acids and amino acids.
Figure 8. Changes in rhizosphere soil metabolites between the pxAlaat3 overexpressing line (OL) and wild-type (WT) plants. (a) OPLS-DA results; (b) Number of differential metabolites; Heat map of metabolite changes in ammonium treatment (c) and nitrate treatment (d); (e) Display of differential metabolites in pathways. Red background: significant differences under ammonium treatment; Blue background: significant differences under nitrate treatment. Yellow background: significant differences under ammonium and nitrate treatments.
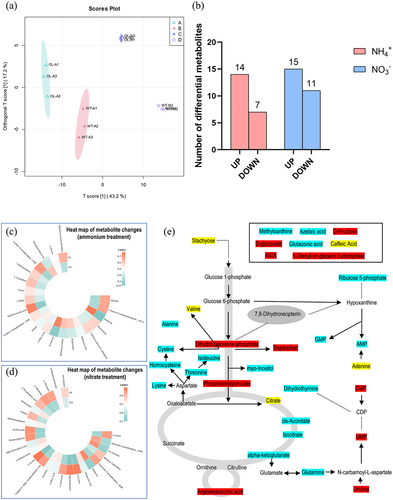
3.6. Joint Analysis of Microorganisms and Metabolites
A joint analysis of microorganisms and metabolites identified in the experimental groups was conducted using Random Forest to assess the contribution of differential metabolites to the microorganisms present (). Under the ammonium nitrogen treatment, L-Glutamate, Stachyose, Uridine, L-Tryptophan, Argininosuccinic acid, and others displayed relatively significant contribution rates. However, the metabolites that had significant contribution rates under the nitrate nitrogen treatment were AMP, cis-Aconitate, Succinate, L-Cystine, and Dihydrothymine, among others. RDA analysis for these substances and the microbial communities identified individually revealed that L-Glutamate and Uridine were closely related to WT-A, while Stachyose, L-Tryptophan, and Argininosuccinic acid had a notable impact on the microbial community of OL-A. Similarly, AMP, cis-Aconitate, and Succinate had a greater influence on WT-A, while L-Cystine and Dihydrothymine had a more pronounced impact on the microbial community of OL-N.
4. Discussion
The majority of the world’s food crops consist of herbaceous plants. In contrast to these food crops, woody cash crops are perennial and have slow growth rates. These distinctions introduce novel factors to be considered in the safety assessment of GM crops. Firstly, the fact that woody plants, including poplar, typically have significantly longer flowering periods than most herbaceous crops, mitigates concerns regarding foreign gene transfer from transgenic plants. Nevertheless, cultivation of transgenic poplar may still lead to unpredictable changes to the soil, therefore requiring comprehensive scrutiny during biosafety evaluation. Secondly, woody plants have different lifecycles than the previous GM crops developed. For instance, while there is no analogous process of straw return as in GM corn,Citation34 woody plants lead to the accumulation and decomposition of fallen leaves. Consequently, safety assessments of GM woody plants should consider these lifecycle differences. Additionally, unlike food crops, poplar is cultivated across diverse environments and can thrive in soils supplied with either ammonium or nitrate nitrogen as nitrogen sources.Citation35 However, variations in the physical and chemical properties of the soil arise when different nitrogen sources are present.Citation36 In the experiments described here, we conducted safety assessments of a transgenic poplar line using either ammonium or nitrate nitrogen sources, laying a crucial experimental foundation for subsequent field trials of transgenic poplar.
The chemical properties of soil affect soil quality and are critical indicators for assessing soil fertility.Citation36 However, as the cultivation of genetically modified crops expands, concerns about whether these crops might impact the surrounding soil ecology have become increasingly prominent, as changes in soil properties can affect crop growth. In our study, we found evidence that the chemical properties of rhizosphere soil can be influenced by the form of nitrogen supplied for plant growth but remain unaffected by the presence of the AlaAT3 transgene. This finding is in line with previous studies of rhizosphere soil properties in transgenic Bt cottonCitation37 and maizeCitation38 cultivation. These results collectively indicate that the introduction of the AlaAT3 gene into P. × xiaohei has no discernible impact on the chemical properties of rhizosphere soil.
Soil enzymes play a crucial role in various biochemical reactions involving the transformation of nitrogen, phosphorus, organic matter, and carbon within the soil. Their activities serve as valuable indicators for assessing the extent and direction of soil biotransformation.Citation39 These enzymes primarily originate from soil microorganism decomposition, plant root exudates, and plant and animal residues.Citation29 Their activity is highly susceptible to environmental factors, like climate, and specific cultivation conditions, including temperature and humidity.Citation40,Citation41 In this study, we investigated the changes in the activities of nine enzymes within the rhizosphere soil of OL and WT greenhouse-grown plants. We observed that the activities of seven major soil functional enzymes were not significantly different between the two lines. However, when subjected to the ammonium nitrogen treatment, the activity of acid phosphatase was notably higher in the rhizosphere soil of OL than in the WT. This is of particular significance as acid phosphatase is closely associated with rhizosphere phosphorus content and phosphorus cycling efficiency, thereby promoting the mineralization of soil organophosphorus.Citation41,Citation42 These changes in acid phosphatase activity might be attributed to alterations in the root microbial community. Conversely, soil protease activity, which plays a critical role in nitrogen mineralization and transformation,Citation43 was also significantly altered, but only under nitrate nitrogen supplementation, although it remains unclear why.
Microorganisms play an integral role in metabolic activities involving the use of carbon and nitrogen sources. The microbial functional diversity constitutes a crucial characteristic within biological communities in soil ecosystems.Citation44 However, previous studies examining soil microbial communities in the roots of GM plants compared to control plants had conflicting results. For instance, bacterial community structure, abundance, and diversity were markedly different in transgenic rice and maize.Citation17,Citation45,Citation46 Additionally, a sugarcane transgenic line engineered with Ea-DREB2B led to a significantly different rhizosphere microbial community diversity compared to the WT.Citation47 In contrast, studies on transgenic maize expressing Hahb-4 and transgenic rice expressing CaMSRB2 only observed minor effects on root-associated bacterial communities.Citation48,Citation49 In this study, we found no significant difference in microbial diversity indices between transgenic and non-transgenic P. × xiaohei plants. This suggests that OL plants did not have an impact on the rhizosphere soil microbial community species richness, dominance, or evenness. It is worth noting that Colombo et al.Citation50 suggested that plant genotype could be one of the contributing factors to changes in the root microbiome.Citation51 Furthermore, considering that soil properties are easily influenced by environmental factors, it is plausible that differences in environmental control between field experiments and controlled laboratory conditions may account for the varying results for the analysis of transgenic root microbiomes.
The comparative analysis of the rhizosphere soil metabolome between the OL and WT revealed significant differences in 21 metabolites under ammonium treatment and 26 metabolites under nitrate treatment. It is important to note that these significantly different metabolites accounted for 7.4% and 9.1% of all detected metabolites, respectively. The majority of the detected metabolites did not have significant changes in expression. Furthermore, the classification of these significantly different metabolites showed they primarily belong to organic acids, amino acids, and other organic compound groups (). In line with previous research, these metabolites, including L-Glutamate, Uridine, Stachyose, L-Tryptophan, Argininosuccinic acid, AMP, cis-Aconitate, Succinate, L-Cystine, and Dihydrothymine, have been associated with metabolism in living organisms.Citation4,Citation52 Importantly, these substances have no demonstrated toxic effects on environmental microorganisms or plants. Therefore, our findings suggest that transgenic P. × xiaohei have a minimal impact on metabolite composition in the rhizosphere soil. The RDA results offer some insight into which metabolites may influence the microbial population, but it remains unclear whether these metabolic differences are due to changes in microbial communities or in substances secreted by the plant roots. This topic will be a focal point for future research.
This study explored the impact of transgenic P × xiaohei on rhizosphere soil, encompassing soil chemistry, soil enzymatic activity, microbial populations, and metabolites. In conclusion, we found that OT plants have limited to negligible effects on the soil environment when cultivated in controlled greenhouse conditions. The influence of transgenic plants on the rhizosphere likely depends on various additional factors.Citation3,Citation5,Citation41,Citation53 Furthermore, recent studies suggest that the specific developmental stage of the tested transgenic plants may impact the soil microbial community differently. As a next step, we plan to perform extended field trials, taking into account the impact of the transgene on the soil during different seasons and plant developmental stages.
5. Conclusion
This study comprehensively assessed the impact of transgenic P. × xiaohei on rhizosphere soil, examining soil chemical properties, enzymatic activity, microbial communities, and metabolites. Our findings show that the rhizosphere soil remained largely unchanged between OL and WT plants under either nitrate nitrogen or ammonium nitrogen supplementation. Comparative analysis of the inter-root metabolome revealed changes in 21 metabolites under ammonium nitrogen and 26 metabolites under nitrate nitrogen. These consisted of primary metabolites, such as organic acids and amino acids, and no harmful substances were identified. Notably, transgenic P. × xiaohei had a slight effect on the enzymatic activity of acid phosphatase under ammonium nitrogen and protease under nitrate nitrogen. In summary, transgenic P. × xiaohei had no significant impact on the chemical properties, microbial communities, or metabolites and enzymes of rhizosphere soil, and did not cause any identifiable harmful effects. However, it is crucial to further investigate whether GM P. × xiaohei in field trials yield different results and whether the ongoing growth and development of GM P. × xiaohei will have environmental repercussions.
Author Contributions
S. Yang and G. Wang performed the experiments. M. Niu, H. Zhang, J. Ma, and G. Liu performed the data analysis and prepared the figures. M. Niu and G. Liu prepared the manuscript, C. Qu revised the manuscript. All authors read and approved the final manuscript.
Supplemental Material
Download Zip (13.1 MB)Acknowledgments
We thank Haijiao Huang (Instrument Sharing Platform, State Key Laboratory of Tree Genetics and Breeding) for providing technical assistance.
Disclosure statement
No potential conflict of interest was reported by the author(s).
Data availability statement
The raw sequence data reported in this paper have been deposited in the Genome Sequence Archive (Genomics, Proteomics & Bioinformatics 2021) in National Genomics Data Center (Nucleic Acids Res 2022), China National Center for Bioinformation/Beijing Institute of Genomics, Chinese Academy of Sciences (GSA: CRA013799) that are publicly accessible at https://ngdc.cncb.ac.cn/gsa.
Supplementary material
Supplemental data for this article can be accessed online at https://doi.org/10.1080/21645698.2024.2339568
Additional information
Funding
References
- Lebedev V, Lebedeva T, Tikhonova E, Shestibratov K. Assessing impacts of transgenic plants on soil using functional indicators: twenty years of research and perspectives. Plants. 2022;11(18):2439. doi:10.3390/plants11182439.
- Srivastava DK, Thakur AK, Kumar P. Agricultural biotechnology: latest research and trends. US: Springer; 2021.
- Guan Z, Lu S-B, Huo Y-L, Guan Z-P, Liu B, Wei W. Do genetically modified plants affect adversely on soil microbial communities? Agric Ecosyst Environ. 2016;235:289–305. doi:10.1016/j.agee.2016.10.026.
- Qiu D, Vuong T, Valliyodan B, Shi H, Guo B, Shannon JG, Nguyen HT. Identification and characterization of a stachyose synthase gene controlling reduced stachyose content in soybean. Theor Appl Genet. 2015;128(11):2167–76. doi:10.1007/s00122-015-2575-0.
- Faragová N, Gottwaldová K, Faragó J. Effect of transgenic alfalfa plants with introduced gene for Alfalfa Mosaic virus coat protein on rhizosphere microbial community composition and physiological profile. Biologia. 2011;66(5):768–77. doi:10.2478/s11756-011-0082-6.
- Quemada H. Lessons learned from the introduction of genetically engineered crops: relevance to gene drive deployment in Africa. Transgenic Res. 2022;31(3):285–311. doi:10.1007/s11248-022-00300-2.
- Chen Z, Wei K, Chen L, Wu Z, Luo J, Cui J. Effects of the consecutive cultivation and periodic residue incorporation of Bacillus thuringiensis (Bt) cotton on soil microbe-mediated enzymatic properties. Agric Ecosyst Environ. 2017;239:154–60. doi:10.1016/j.agee.2017.01.017.
- Chen Z, Chen L, Wu ZJ. Relationships among persistence of Bacillus thuringiensis and Cowpea trypsin inhibitor proteins, microbial properties and enzymatic activities in rhizosphere soil after repeated cultivation with transgenic cotton. Appl Soil Ecol. 2012;53:23–30. doi:10.1016/j.apsoil.2011.10.019.
- Wei HW, Movahedi A, Liu G, Kiani-Pouya A, Rasouli F, Yu C, Chen Y, Zhong F, Zhang J. Effects of field-grown transgenic Cry1Ah1 poplar on the rhizosphere microbiome. Res Sq. 2022. doi:10.21203/rs.3.rs-78068/v2.
- Wang G, Jin Z, Wang X, George TS, Feng G, L Zhang. Simulated root exudates stimulate the abundance of Saccharimonadales to improve the alkaline phosphatase activity in maize rhizosphere. Appl Soil Ecol. 2022;170:104274. doi:10.1016/j.apsoil.2021.104274.
- Łukaszkiewicz J, Długoński A, Fortuna-Antoszkiewicz B, Fialová J. The potential of poplars (Populus L.) for the sustainable environment of cities. Preprints. 2024. doi:10.20944/preprints202402.1542.v1.
- Parsons TJ, Sinkar VP, Stettler RF, Nester EW, Gordon MP. Transformation of poplar by Agrobacterium tumefaciens. Bio/Technology. 1986;4(6):533–36. doi:10.1038/nbt0686-533.
- Hu J-Q, Qi Q, Zhao Y-L, Tian X-M, Lu H, Gai Y, Jiang X-N. Unraveling the impact of Pto4CL1 regulation on the cell wall components and wood properties of perennial transgenic populus tomentosa. Plant Physiol Bioch. 2019;139:672–80. doi:10.1016/j.plaphy.2019.03.035.
- Movahedi A, Zhang J, Gao P, Yang Y, Wang L, Yin T, Kadkhodaei S, Ebrahimi M, Zhuge Q. Expression of the chickpea CarNAC3 gene enhances salinity and drought tolerance in transgenic poplars. Plant Cell Tissue Organ Cult. 2015;120(1):141–54. doi:10.1007/s11240-014-0588-z.
- Ren Y, Zhang J, Liang H, Wang J, Yang M. Inheritance and expression stability of exogenous genes in insect-resistant transgenic poplar. Plant Cell Tiss Organ Cult. 2017;130(3):567–76. doi:10.1007/s11240-017-1247-y.
- Yang Y, Tang RJ, Jiang CM, Li B, Kang T, Liu H, Zhao N, Ma X-J, Yang L, Chen S-L, Zhang H-X. Overexpression of the P tSOS2 gene improves tolerance to salt stress in transgenic poplar plants. Plant Biotechnol J. 2015;13(7):962–73. doi:10.1111/pbi.12335.
- Li P, Ye S, Liu H, Pan A, Ming F, Tang X. Cultivation of drought-tolerant and insect-resistant rice affects soil bacterial, but not fungal, abundances and community structures. Front Microbiol. 2018;9:1390. doi:10.3389/fmicb.2018.01390.
- Peña PA, Quach T, Sato S, Ge Z, Nersesian N, Dweikat IM, Soundararajan M, Clemente T. Molecular and phenotypic characterization of transgenic wheat and sorghum events expressing the barley alanine aminotransferase. Planta. 2017;246(6):1097–107. doi:10.1007/s00425-017-2753-1.
- Good A, Johnson S, de Pauw M, Carroll R, Savidov N, Vidmar J, Lu Z, Taylor G, Stroeher V. Engineering nitrogen use efficiency with alanine aminotransferase. Can J Bot. 2007;85(3):252–62. doi:10.1139/b07-019.
- K SA, T CR, M D, Taylor GJ, Good AG. Genetic engineering of improved nitrogen use efficiency in rice by the tissue‐specific expression of alanine aminotransferase. Plant Biotechnol J. 2008;6(7):722–32. doi:10.1111/j.1467-7652.2008.00351.x.
- Snyman SJ, Hajari E, Watt MP, Lu Y, Kridl JC. Improved nitrogen use efficiency in transgenic sugarcane: phenotypic assessment in a pot trial under low nitrogen conditions. Plant Cell Rep. 2015;34(5):667–69. doi:10.1007/s00299-015-1768-y.
- McAllister CH, Good AG. Alanine aminotransferase variants conferring diverse NUE phenotypes in Arabidopsis thaliana. PLoS One. 2015;10(4):e0121830. doi:10.1371/journal.pone.0121830.
- Hewitt EJ. Sand and culture methods used in the study of plant nutrition. Soil Sci. 1966;75(1):x+241:202–32. doi: 10.1097/00010694-195301000-00019.
- Bao S. Soil and agricultural chemistry analysis. US: China agriculture press; 2000.
- Walkley A, Black IA. An examination of Degtjareff method of determining soil organic matter and proposed modification of the method of the chromic acid titration method. Soil Sci. 1934;37(1):29–39. doi:10.1097/00010694-193401000-00003.
- Huse SM, Dethlefsen L, Huber JA, Welch DM, Relman DA, Sogin ML. Exploring microbial diversity and taxonomy using SSU rRNA hypervariable tag sequencing. PLoS Genet. 2008;4(11):e1000255. doi:10.1371/journal.pgen.1000255.
- Chen S, Zhou Y, Chen Y, Gu J. Fastp: an ultra-fast all-in-one FASTQ preprocessor. Bioinformatics. 2018;34(17):i884–90. doi:10.1093/bioinformatics/bty560.
- Magoč T, Salzberg SL. FLASH: fast length adjustment of short reads to improve genome assemblies. Bioinformatics. 2011;27(21):2957–63. doi:10.1093/bioinformatics/btr507.
- Edgar RC. UPARSE: highly accurate OTU sequences from microbial amplicon reads. Nat Methods. 2013;10(10):996–98. doi:10.1038/nmeth.2604.
- Qi Y, Wang Q, Xie Q, Wu C, Xu M, Han S, Zhou T, Li J, Xia L, Li WC, Pan W. Safety evaluation of FAD2 RNAi transgenic Brassica napus L. based on microbial diversity and metabonomic analysis. Front Plant Sci. 2022;13:953476. doi:10.3389/fpls.2022.953476.
- Wang K, Liu M, Cai C, Cai S, Ma X, Lin C, Zhu Q. The impact of genetic modified Ma bamboo on soil microbiome. Front Microbiol. 2022;13:1025786. doi:10.3389/fmicb.2022.1025786.
- Want EJ, Wilson ID, Gika H, Theodoridis G, Plumb RS, Shockcor J, Holmes E, Nicholson JK. Global metabolic profiling procedures for urine using UPLC–MS. Nat Protoc. 2010;5(6):1005–18. doi:10.1038/nprot.2010.50.
- Smith CA, Want EJ, O’Maille G, Abagyan R, Siuzdak G. XCMS: processing mass spectrometry data for metabolite profiling using nonlinear peak alignment, matching, and identification. Anal Chem. 2006;78(3):779–87. doi:10.1021/ac051437y.
- Xu X, Liu X, Li F, Hao C, Sun H, Yang S, Jiao Y, Lu X. Impact of insect-resistant transgenic maize 2A-7 on diversity and dynamics of bacterial communities in Rhizosphere soil. Plants. 2023;12(10):2046. doi:10.3390/plants12102046.
- Rennenberg H, Wildhagen H, Ehlting B. Nitrogen nutrition of poplar trees. Plant Biol. 2010;12(2):275–91. doi:10.1111/j.1438-8677.2009.00309.x.
- Delgado A, Gómez JA. The soil. Physical, chemical and biological properties. Princip Agron Sustain Agricul. 2016;15–26. doi:10.1007/978-3-319-46116-8_2.
- Ahamd M, Abbasi WM, Jamil M, Iqbal M, Hussain A, Akhtar MF-Z, Nazli F. Comparison of rhizosphere properties as affected by different Bt-and non-Bt-cotton (Gossypium hirsutum L.) genotypes and fertilization. Environ Monit Assess. 2017;189(6):1–10. doi:10.1007/s10661-017-5994-3.
- Yang S, Liu X, Xu X, Sun H, Li F, Hao C, Lu X. Effects of insect-resistant Maize 2A-7 expressing mCry1ab and mCry2ab on the soil ecosystem. Plants. 2022;11(17):2218. doi:10.3390/plants11172218.
- Du J, Hou F, Zhou Q. Response of soil enzyme activity and soil bacterial community to PCB dissipation across different soils. Chemosphere. 2021;283:131229. doi:10.1016/j.chemosphere.2021.131229.
- Liang J, Xin L, Luan Y, Song X, Zhang ZG. Effect of Cry1Ie Bt maize on carbon source metabolism of rhizosphere microorganisms. J Agric Sci Technol. 2019;21:104–10. doi:10.13304/j.nykjdb.2018.0133.
- Li K, Wang C, Ow DW. Root microbiome changes associated with cadmium exposure and/or overexpression of a transgene that reduces Cd content in rice. Ecotox Environ Safe. 2022;237:113530. doi:10.1016/j.ecoenv.2022.113530.
- Yang MK, Luo FH, Song YC, Ma SL, Ma YD, Fazal A, Yin T, Lu G, Sun S, Qi J, Wen Z, Li Y, Yang Y. The host niches of soybean rather than genetic modification or glyphosate application drive the assembly of root-associated microbial communities. Microb Biotechnol. 2022;15(12):2942–57. doi:10.1111/1751-7915.14164.
- Greenfield LM, Hill PW, Seaton FM, Paterson E, Baggs EM, Jones DL. Is soluble protein mineralisation and protease activity in soil regulated by supply or demand? Soil Biol Biochem. 2020;150:108007. doi:10.1016/j.soilbio.2020.108007.
- Wu N, Shi W, Liu W, Gao Z, Han L, Wang X. Differential impact of Bt-transgenic rice plantings on bacterial community in three niches over consecutive years. Ecotox Environ Safe. 2021;223:112569. doi:10.1016/j.ecoenv.2021.112569.
- Fang M, Kremer RJ, Motavalli PP, Davis G. Bacterial diversity in rhizospheres of nontransgenic and transgenic corn. Appl Environ Microbiol. 2005;71(7):4132–36. doi:10.1128/aem.71.7.4132-4136.2005.
- Pack IS, Heo JH, Kim DY, Cho HJ, Oh S-D, Lee S-K, Suh E-J, Kim C-G. Bacterial communities associated with the rhizosphere of transgenic chrysanthemum. Singmul Hakhoe Chi. 2023;66(3):257–68. doi:10.1007/s12374-023-09392-7.
- Zhao X, Jiang Y, Liu Q, Yang H, Wang Z, Zhang M. Effects of drought-tolerant Ea-DREB2B transgenic sugarcane on bacterial communities in soil. Front Microbiol. 2020;11:704. doi:10.3389/fmicb.2020.00704.
- Ibarra JG, Colombo RP, Godeas AM, López NI. Analysis of soil bacterial communities associated with genetically modified drought-tolerant corn. Appl Soil Ecol. 2020;146:103375. doi:10.1016/j.apsoil.2019.103375.
- Sohn S-I, Oh Y-J, Kim B-Y, Cho HS. Effects of CaMSRB2-expressing transgenic rice cultivation on soil microbial communities. J Microbiol Biotechnol. 2016;26(7):1303–10. doi:10.4014/jmb.1601.01058.
- Colombo RP, Ibarra JG, Bidondo LF, Silvani VA, Bompadre MJ, Pergola M, Lopez NI, Godeas AM. Arbuscular mycorrhizal fungal association in genetically modified drought‐tolerant corn. J Environ Qual. 2017;46(1):227–31. doi:10.2134/jeq2016.04.0125.
- Chialva M, De Rose S, Novero M, Lanfranco L, Bonfante P. Plant genotype and seasonality drive fine changes in olive root microbiota. Curr Plant Biol. 2021;28:100219. doi:10.1016/j.cpb.2021.100219.
- Sun C, Jin L, Cai Y, Huang Y, Zheng X, Yu T. L-Glutamate treatment enhances disease resistance of tomato fruit by inducing the expression of glutamate receptors and the accumulation of amino acids. Food Chem. 2019;293:263–70. doi:10.1016/j.foodchem.2019.04.113.
- Wei B, Zhang J, Wen R, Chen T, Xia N, Liu Y, Wang Z. Genetically modified sugarcane intercropping soybean impact on rhizosphere bacterial communities and co-occurrence patterns. Front Microbiol. 2021;12:742341. doi:10.3389/fmicb.2021.742341.