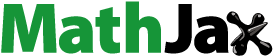
ABSTRACT
Phthalic acid esters (PAEs) are highly toxic compounds and can disrupt the hormonal balance of human, animal, and aquatic organisms. Due to the hazardous nature of such compounds, their removal from constituent wastewater before discharging into the environment is mandatory. This study focused on the biodegradation of dimethyl phthalates (DMP), di-n-butyl phthalates (DBP), and di-n-octyl phthalates (DnOP) by Gordonia sp. in a batch system. Initially, five different concentrations of DBP, DMP, and DnOP (200–1000 mg/L) were chosen individually as the sole carbon source to examine their effect on the biodegradation and biomass growth of Gordonia sp. Complete degradation of DBP and DMP was achieved up to 1000 mg/L initial concentration within 96 h, whereas in case of DnOP, the degradation value was only 83.5% at 120 h for the same initial concentration. The experimental data were fitted into various substrate inhibition kinetic models, and accurate predicted values of degradation of all the three PAEs were obtained using the Tiesser model in comparison with other models, which yielded the highest and lowest R2 and SSE values of 0.99 and 0.02 × 10−4, respectively. In addition, the phytotoxicity of PAEs degraded samples was assessed and more than 50% germination index value was observed for DMP and DBP degraded sample which established the treatment efficiency of Gordonia sp. in degrading DMP and DBP. Hence, high DMP and DEP degradation and phytotoxicity removal efficiency of Gordonia sp. demonstrate its potential for the treatment of PAEs contaminated wastewater.
HIGHLIGHTS
100% degradation of DBP and DMP was achieved at 200–1000 mg/L initial concentrations.
This is the first study on evaluation of DMP, DBP, and DnOP inhibition on Gordonia sp.
Teisser model accurately described the inhibitory effect of phthalate.
DMP and DEP degraded samples (1000 mg/L Initial concentration) showed negligible effect on chickpea seeds germination.
1. Introduction
Phthalic acid esters (PAEs) are referred as phthalate esters and commonly used to produce plastics for manufacturing of commercial and consumer goods [Citation1]. These compounds are chemically synthesized from phthalic anhydride and alcohols. In addition to their colorless, odorless, and flavorless nature, they are chemically stable and can exist in liquid form at a wide range of temperatures (25–50°C) [Citation2]. PAEs are nonsteroidal endocrine-disrupting compounds (EDCs) that mimic the functionality of natural hormones and interfere with their normal activity, thereby causing reproductive and behavioral disturbances [Citation3]. In addition, PAEs are hepatotoxic, carcinogenic, and teratogenic [Citation4]. The covalent bond between these compounds and the polymeric matrix is the reason for their accumulation in the environment which may happen during manufacturing or due to leaching from finished products [Citation5–7]. As a result, phthalates are commonly found in wastewater from industries that manufacture them and constitute more than 5% of the total organics present [Citation8]. Among different phthalates, di-(2-ethylhexyl) phthalate (DEHP) is the most regulated; however, other phthalates are also becoming a regulatory concern. The various phthalates of emerging concern include butyl benzyl phthalate (BBP), dibutyl phthalate (DBP), diethyl phthalate (DEP), di-(2-ethylhexyl) phthalate (DEHP), dimethyl phthalate (DMP), and di-n-octyl phthalate (DnOP) [Citation9].
DMP has been identified as a severe environmental pollutant due to its widespread use in different industries. It is a relatively stable molecule in the natural environment with a half-life of around 20 years, leads to chromosomal injuries in human leucocytes as an endocrine disruptor compound and negatively impacts animal reproduction and human development [Citation10,Citation11]. DBP is the most frequently utilized PAE in the cosmetics, baby care, and plastics industries. It poses a serious risk to the ecosystem and public health due to its occurrence in every segment of the environment, including air, dust, drinking water, surface water, sediments, and soil [Citation12]. DBP has been shown to impair human sperm production and motility, lower pregnancy rates, and increase miscarriages [Citation10]. DnOP is another priority pollutant identified by numerous regulatory agencies due to its low water solubility, high endurance in the ecosystem, and detrimental consequences on the liver, kidney, thyroid, and immune systems [Citation13].
PAEs, however, scarcely undergo natural environmental degradation due to their highly recalcitrant nature. For instance, DEHP has a half-life of around 2000 years in the natural aqueous environment [Citation14]. Therefore, removal or degradation of PAEs in the environment has been a key research focus. The removal or degradation of phthalates from the environment can be accomplished through a variety of abiotic methods, such as physical, chemical, advanced oxidation processes, and combinations of these [Citation14–17]. However, these techniques have a number of drawbacks, including the creation of secondary sludge, high process cost, and severe operating conditions [Citation18]. Compared to the physico-chemical methods, biodegradation is more favorable for PAE degradation in water, soil, and sediment.
The microbial breakdown of PAEs has been described in earlier investigations, and certain microbial species with PAE degradation capabilities have been identified [Citation19]. Achromobacter denitrificans SP1, Agromyces sp. MT-O, Bacilus sp. KS1, Micrococcus sp. KS2, Paenarthrobacter sp. Shss, Pseudomonas aeruginosa, Rhodococcus pyridinivorans XB, Ralstoia picketii, etc. are bacterial strains reported to degrade different PAEs [Citation20–25]. Microbial degradation provides a practical and eco-friendly approach to remediating PAE-contaminated environment [Citation26]. In the literature, PAE degradation by microorganisms is reported with the objective to elucidate their biodegradation pathways and mechanisms involved [Citation27–29]. Very few studies are available on their biodegradation kinetics or modeling which is important for successful development of such bioprocess systems. For instance, in a study on biodegradation of DEHP by halotolerant bacterial consortium, Li et al. [Citation30] reported that the degradation of DEHP, in the range of 100 to 2000 mg/L initial concentration, shows best fit to the first-order kinetic.
Therefore, this study aimed to describe the kinetics of biodegradation of different molecular weight phthalates (DMP, DBP, and DnOP) by Gordonia sp. The results were fitted to different kinetic models for analyzing the kinetics of phthalate degradation, and biomass growth by Gordonia sp. Besides, phytotoxicity of the degraded phthalate was assessed by determining their effect on germination index of chickpea seeds.
2. Materials and methodology
2.1. Chemicals
The three phthalates, namely, dimethyl phthalate (DMP), dibutyl phthalate (DBP), and di-n-octyl phthalate (DnOP), used in this study were supplied by Tokyo Chemical Industry Co. Ltd. (TCI) located in Chennai, India. The chemical structure of the phthalates is shown in . Luria Bertani broth for culture growth was provided by High-Media, India.
2.2. Bacterial strain and culture conditions
Gordonia sp. used in this biodegradation study was previously reported in our earlier study [Citation31] and procured from Bose Institute (Kolkata, India). For biomass growth of the culture, Luria Bertani (LB) broth medium was used and the culture conditions were 30°C temperature & 150 rpm shaking in an orbital incubator shaker. For PAEs biodegradation by the bacterium, a minimal salt medium (MSM) was used along with the individual PAEs (DMP, DBP, or DnOP) as the sole carbon source and energy source. The effect of different composition of MSM along with other parameters that affect the PAE biodegradation by the bacterium was studied and their levels optimized as described in the next section.
2.3. Optimization of different process parameters on phthalate biodegradation
In order to optimize temperature, pH, and media composition which individually affect PAE biodegradation, batch experiments were conducted using Erlenmeyer flasks (250 mL). For optimizing the medium composition to support biomass growth and phthalate biodegradation, three MSM with different compositions were examined (). The effects of five different temperature and pH in the range of 26–34°C and 5–9, respectively, were investigated on biodegradation of PAEs (DMP, DBP, and DnOP) mixture by Gordonia sp. at 500 mg/L total initial phthalate concentration.
Table 1. Composition of different media used for optimization.
2.4. Biodegradation experiment
To study the biodegradation of phthalates, low molecular weight DMP, medium molecular weight DBP, and high molecular weight DnOP were used. The experiment was conducted with each substrate added separately to a 250-mL Erlenmeyer flask containing MSM-3. Five different initial concentrations of 200, 400, 600, 800, and 1000 mg/L of the three PAEs were chosen as the sole carbon source for their biodegradation and biomass growth by Gordonia sp. The bacterial inoculum was prepared by culturing Gordonia sp. in MSM containing 500 mg/L of the respective phthalate for 48 h at 30°C. A 10% (v/v) inoculum of mid-log phase growing culture (OD 1.0) was used for subsequent tests. In order to check the abiotic loss of PAEs, A control flask without any added bacterial inoculum was maintained at a concentration of 1000 mg/L for each phthalate.
2.5. Analytical methods
2.5.1. Determination of biomass growth
Biomass growth of Gordonia sp. was determined by UV-visible spectroscopy using Evolution 201TM spectrophotometer (Thermo Scientific, USA) set at a wavelength of 660 nm [Citation31]. The biomass concentration was calculated using a calibration curve that was previously generated between the culture’s dry biomass weight (DBW) and OD660. To determine the DBW, gravimetric analysis was performed by centrifuging the culture broth at 12,000 × g for 15 min at 25°C. After washing the cell pellet twice with phosphate buffer, it was lyophilized and the final weight was recorded.
2.5.2. Determination of phthalate concentration
To analyze the phthalate concentration, samples were centrifuged at 12,000 × g and the supernatant was extracted twice with an equivalent volume of dichloromethane (DCM). The extracted samples were kept at room temperature to let the solvent evaporate. The details of the sample preparation were reported in our earlier work [Citation31]. Afterward, methanol was added to the samples in equal amounts to dissolve phthalates. The concentration of phthalates was determined by high-pressure liquid chromatography (HPLC) (SPD-20 A, Shimadzu, Japan) using a C-18 column of internal diameter and length = 250 × 4.6 mm, size = 5 μm and a UV detector set at a wavelength of 254 nm. The mobile phase was composed of deionized water and methanol in a ratio 1:4 with a flow rate of 1.0 mL/min. Retention time of each phthalate in the experimental sample was compared to that of the corresponding standard in methanol for further analysis of the results. The percent degradation of phthalates in the experiment was expressed using the following equation:
where Cf and Ci are the final and initial phthalate concentrations in mg/L.
2.6. Phytotoxicity study
To evaluate the effectiveness of Gordonia sp. in PAEs degradation, a seed germination test was carried out on samples taken during the experiments. Phytotoxicity of raw/untreated as well as degraded DMP, DBP, and DnOP was assessed at a dilution of 1000 mg/L initial concentration of the PAEs, with distilled water used as a negative control. PAEs degraded samples were centrifuged at 8000 × g for 10 min, and supernatant was assessed for phytotoxicity. Cicer arietinum L. (Chickpea seeds) germination and their growth is very sensitive toward toxic compounds like phthalates; therefore, it is selected for this toxicity analysis [Citation34]. Chickpea seeds were placed on separate petri plates and 20 mL of distilled water, tap water, raw/untreated PAEs, and degraded PAEs were added individually to each plate. The plates were then incubated at 28°C for 24 h and the seeds were subsequently removed, wrapped in damp cotton cloths, and observed for 48 h. The GI (Germination Index) was calculated using the following formula, which takes into account the root length of each seed.
2.7. Kinetic modeling
Kinetics of biomass growth of Gordonia sp. and biodegradation of DMP, DBP, and DnOP were investigated by fitting the experimental results to different biokinetic models reported in the literature.
2.7.1. Biomass growth kinetics
Assuming minimal cell decay occurs in batch cultures, the following first-order differential equation was used to describe the biomass (X) growth of Gordonia sp. on PAEs:
Integrating EquationEquation 4(4)
(4) while considering initial biomass concentration, X0 (mg/L):
2.7.2. Substrate degradation kinetics
Microorganisms degrade organic compounds by utilizing them as the carbon source for their biomass growth and cell maintenance activities. EquationEquation 7(7)
(7) provides a general mass balance expression for substrate degradation under batch system.
To get the following Equation 9 for describing substrate degradation as a function of time, Equation 8 is integrated and the value of X is substituted from Equation 6.
where S0 represents the initial concentration of substrate in mg/L.
2.7.3. Substrate inhibition on biomass growth and degradation kinetics
Different substrate inhibition models were employed to study the inhibition due to PAEs on biomass growth and PAEs degradation by Gordonia sp. The specific biomass growth rate was calculated using the biomass growth profile of Gordonia sp. for all initial concentrations of DMP, DBP, and DnOP. The maximum specific growth rates and other kinetic parameters of substrate inhibition on Gordonia sp. biomass growth was estimated using substrate inhibition models as shown in . The Equations were modified by replacing q with µ.
Table 2. Substrate inhibition biokinetic models applied to estimate kinetics parameters of DBP, DMP, and DnOP biodegradation by Gordonia sp.
The modified substrate inhibition biokinetic model expressions are given in . Specific degradation rates of PAEs were estimated based on the calculated specific growth rates of the bacterium and biomass yield obtained at various initial concentrations of DBP, DMP, and DnOP.
where qS is the specific substrate degradation rate in mg/mg·h.
S: substrate concentration (mg/L); K: constant of Edward and Webb models; KI: PAEs inhibition constant (mg/L), Ks: half saturation constant (mg/L); qmax: maximum specific substrate biodegradation rate (mg/mg·h); q: specific substrate biodegradation rate (mg/mg·h).
2.7.4. Estimation of biokinetic parameters
The model fitting exercise was carried out by non-linear least square method to minimize the sum of squared errors (SSE) between experimental and models predicted values, and using the solver function tool of Microsoft Excel 2019 [Citation39].
where Dexp and Dpred are the experimental and model predicted data, respectively, and n represents the number of observations.
3. Results and discussion
3.1. Effect of different process parameters on biodegradation of phthalates
The effect of various media (MSM) with different chemical compositions on biodegradation of phthalates by Gordonia sp. was first studied at 500 mg/L total initial concentration of PAEs, pH 7.0 and 30°C temperature. After 5 days of incubation, maximum phthalate degradation (>90%) was obtained in MSM 3. More than 80% degradation efficiency was achieved using MSM 1, whereas a very low efficiency was observed using MSM 2 (). The all three MSMs were composed with the combination of different compounds which were used at different concentrations. The results of the MSM screening reveal that the all compounds required for the growth and metabolic activity of Gordonia sp. were present in optimum concentration in MSM 3.
Figure 2. Effect of different parameters on phthalate degradation by Gordonia sp.: (a) MSM, (b) temperature and (c) pH.

The effect of five different temperatures in the range of 26–34°C was further investigated on biodegradation of phthalates at 500 mg/L total initial concentration and 7.0 pH. Complete degradation of the phthalates was achieved at 30°C after 5 days of incubation. The results further revealed that phthalate degradation was high in the temperature range 30–34°C, and temperature below 28°C did not yield sufficient phthalate degradation efficiency (). Several studies have been reported in the literature supporting that 30°C temperature is optimum for maximum growth and metabolism of Gordonia sp [Citation40–42].
shows the phthalate degradation profile obtained using the Gordonia sp. at different pH in the range of 5.0–9.0, 30°C temperature and 500 mg/L initial PAEs concentration. Very high phthalate degradation is observed in the pH range 6.0–8.0, whereas pH value below 6 or above 8 did not result in high degradation of the phthalates due to toxic effect of acidic and basic medium on the biomass growth of Gordonia sp. Thus, complete phthalate biodegradation was achieved at pH 7.0 after 5 days of incubation.
3.2. Kinetics of biomass growth and PAEs degradation by Gordonia sp
3.2.1. Biomass growth
depicts the biomass growth of Gordonia sp. on DBP, DMP, and DnOP as the sole carbon source in the batch experiments, which reveal that the maximum biomass concentration values of 566, 829, and 1340 mg/L were obtained at 1000, 1000, and 800 mg/L initial concentration of DMP, DBP, and DnOP within 84, 96, and 108 h, respectively. Estimated values of biokinetic parameters on Gordonia sp. biomass growth on different PAEs using the Verholt model are presented in , which reveals that maximum growth rate of Gordonia sp. was 12.35, 14.49, and 22.04 mg/L⋅ h for DMP, DEP, and DnOP at 1000, 1000, and 800 mg/L initial concentrations ().
Figure 3. Time profile of experimental and model predicted values of biomass growth of Gordonia sp. on (a) DMP, (b) DBP and (c) DnOP.

Table 3. Estimated biokinetic parameters of biomass growth of Gordonia sp. on PAEs.
An increase in the initial concentration of DBP, DMP, and DnOP from 200 to 1000 mg/L led to an increase in lag time of biomass growth. In case of DMP, lag phase was prolonged from 0.76 to 39.03 h, whereas the values were 0.43 to 32.11 h in case of DBP. In case of DnOP, lag phase values ranged from 3.49 to 39.11 h. Similar results of lag phase in biomass growth of Rhodococcus ruber and microbial consortium SK-1 during biodegradation of DMP, DEP, and DBP at different initial concentration were reported by Kolb et al. [Citation43]. The increase in lag time for biomass growth due to an increase in phthalate concentration is attributed to the inhibitory effect of PAEs on biomass growth [Citation44]. and reveal that the lag phase in biomass growth due to DBP and DnOP is more as compared to that with DMP. Compared to DMP, DBP and DnOP are chemically complex in structure (), which resulted in prolonged lag phase by the microbial enzymes to metabolize the PAEs. After a lag phase, an exponential growth phase followed till 84, 96, and 108 h for 1000, 1000, and 800 mg/L initial concentrations of DBP, DMP, and DnOP, respectively. Subsequently, a stationary phase is observed where the growth rate decreased with small fluctuation. All of these findings show that the Gordonia sp. biomass growth is highly dependent on the initial concentration of PAEs and their corresponding molecular weights in the various experimental runs. The similar observation has been reported by Wang et al. [Citation26] in their study on the biodegradation of DEHP by Gordonia sp. Value of coefficient of determination (R2) was examined to demonstrate the goodness of fit of experimental values with the model predicted data. From , it is apparent that R2 values due to the model varied between 0.91 and 0.99 which reveals a good agreement between the model predicted and experimental biomass values ().
3.2.2. Phthalates degradation
Biodegradation of DBP, DMP, and DnOP by Gordonia sp. served the bacterium to utilize these compounds as the sole carbon source for its biomass growth and maintenance. The degradation % of PAEs at different initial concentrations is present in . Complete degradation at all initial concentrations of DMP and DBP was observed, even at their high initial concentration of 1000 mg/L. Complete degradation of DMP was achieved within 84 h, and the value was 96 h in case of DBP (). In the case of DnOP, complete degradation was achieved up to 800 mg/L within 108 h, whereas at 1000 mg/L, the degradation value was 83% which was achieved in 120 h (). Compared to these degradation values, the value due to abiotic loss during the experiments is negligible. From these results, it could be concluded that the initial concentration, molecular weight, and structure of phthalates played a significant role on their biodegradation. Compared to the high molecular weight (HMW) phthalate (DnOP), low molecular weight (LMW) phthalates (DMP and DBP) contain simple structures and are quickly degraded, even at high initial concentrations (). The literature reported values are consistent with the findings of this study [Citation31]. It is known that the long ester side chains of high molecular weight phthalates pose a steric hindrance to the hydrolytic enzymes of the degrading bacteria, resulting in their low degradation rates [Citation28,Citation45]. Tuan Tran et al. [Citation2] also reported that the variation in phthalate biodegradability is due to the steric impact of the phthalates side ester chains, which prevents hydrolytic enzymes from adhering to the phthalates and their degradation. These results of PAEs degradation correlate well with the biomass growth by Gordonia sp. Hence, it could be concluded that LMW phthalates like DMP DBP can be degraded completely and quickly as compared with HMW phthalates. The finding of this study is consistent with the literature report by Nahurira [Citation42] for biodegradation of phthalate by a novel Gordonia alkanivorans YC-RL2, in which study they found that the bacterium can completely degrade LMW phthalates (DMP, DEP, and DBP), but low degradation was reported for DEHP (HMW phthalate). Also, in another study, complete degradation was reported for DMP and DEP, whereas low degradation was observed for DEHP degradation by bacterial consortium of Gordonia sp. and Pseudomonas sp [Citation46].
Figure 4. Time profile of percentage biodegradation of (a) DMP, (b) DBP and (c) DnOP at different initial concentration by Gordonia sp.

The acquired biodegradation results were further fitted to the modified substrate degradation expression (EquationEquation 8(8)
(8) ) for estimating the biokinetic parameters involved. Experimental and model predicted degradation profile of DMP, DBP, and DnOP are illustrated in and the estimated biokinetic parameters are presented in . It is clear that the predicted values, from the modified substrate degradation expression, accurately fitted the entire experimental data of DMP, DBP, and DnOP degradation with high coefficient of determination (R2) values in the range of 0.94–0.99. The inhibitory effects of the PAEs at high initial concentration are supported by the estimates of the model biokinetic parameters mentioned in , which reveals that the biomass yield (YX/S) on DMP, DBP, and DnOP was not constant, but varied insignificantly with initial PAEs concentration. In case of DMP and DBP, the biomass yield values increased slightly with an increase in initial concentration of the PAEs, whereas the cellular maintenance values are very high 0.47 and 2.13 g/g at 1000 mg/L as compared to the values 0.13 and 0.01 g/g at 200 mg/L initial concentration of DMP and DBP, respectively. Moreover, in case of DnOP, biomass yield decreased from 1.67 to 1.44 g/g along with sixfold increase in cellular maintenance from 0.18 to 1.06 g/g with an increase in initial concentration from 600 to 1000 mg/L, respectively. A decrease in biomass yield on substrate and an increase in maintenance energy indicates a significant inhibitory effect due to initial PAEs concentration.
Figure 5. Experimental v/s model predicted results of biodegradation of (a) DMP, (b) DBP, and (c) DnOP at different initial concentration ranging from 200 to 1000 mg/L.

Table 4. Estimated kinetic model parameters of DBP, DMP, and DnOP degradation.
3.2.3. Substrate inhibition kinetics
To evaluate the PAEs inhibition on their degradation, the biomass-specific growth rate of the bacterium was calculated by EquationEquation 10(10)
(10) using the experimental biomass concentration (X) at varying initial PAEs concentrations. The experimental degradation values of the PAEs and biomass-specific growth rates were further used to calculate the specific degradation rates (EquationEquation 11
(11)
(11) ) for all initial concentrations of DMP, DBP, and DnOP. These calculated specific degradation rate values were thus used to assess the PAEs inhibition on their degradation employing different substrate inhibition models (). The biokinetic parameter values estimated from these substrate inhibition models are presented in . compares the experimental specific degradation rates and model predicted values. From the figure, decrease in specific degradation rate value with an increase in the initial DMP, DBP, and DnOP concentration is observed, which explains the inhibitory effect of high initial concentration of PAEs on their own biodegradation. Comparison of the data shown in reveals that the specific degradation rate values decreased substantially due to structural complexity of the PAEs [Citation45]. These results are also consistent with the previous results on PAE degradation at different initial concentration, presented in and .
Figure 6. Experimental specific substrate degradation rate values v/s model predicted data for various initial concentration of (a) DMP, (b) DBP, and (C) DnOP.

Table 5. Biokinetic model parameter values estimated using the substrate inhibition models.
The goodness of fit of the model predicted degradation rate values with the experimental data was assessed based on R2 and SSE values for the different models [Citation39]. From , it can be seen that Teisser model showed the best fit with high R2 value of 0.99 and least SSE value of less than 0.3 × 10−4 for DMP, DBP, and DnOP, whereas Monod and Webb models showed very poor fit. Hence, from the estimated biokinetic parameters of Teisser model, maximum specific degradation values of 0.0935, 0.0877, and 0.0383 h−1 agreed well with the experimental values for DMP, DBP, and DnOP, respectively. Moreover, the inhibitory concentration of DMP, DBP, and DnOP, estimated using the Teisser model, were 1274, 1109, and 885 mg/L which correlated well with the experimental values. Kanaujiya and Pakshirajan [Citation38] studied the inhibitory effect of DMP and DEP on their degradation and biomass growth by Cellulosimicrobium funkei and identified Teisser model as the best model to describe the inhibitory effect. The other models used in this study such as Andrews, Aiba, and Edward, also shown good fit with low SSE value (<13 × 10−5) and high R2 value (>0.97) for all the PAEs. However, the estimated maximum specific degradation rate and inhibitory concentration values due to these models do not correlate well with the experimentally determined values. Similar observations were reported by on the inhibitory effect of trichloroethylene on its biodegradation by Rhodococcus opacus [Citation47].
In order to estimate the biokinetic parameters of PAEs inhibition on biomass of Gordonia sp., the experimental specific growth rate values were fitted to different substrate inhibition models presented in . For this estimation, the q was replaced with µ in the equations of . The concentration profile of experimental and predicted values of specific biomass growth rates on DMP, DBP, and DnOP is depicted in . reveals that Teisser model best-fitted the biomass growth with high R2 value (more than 0.99) and low SEE (less than 0.23 × 10−4) for all the PAEs. The maximum specific growth rate of Gordonia sp., 0.044, 0.048, and 0.043 h−1, and substrate inhibitory concentrations, 1147, 1075, and 829 mg/L, were estimated by the Teisser model for DMP, DBP, and DnOP, respectively. These predicted values match well with the experimental values. The biokinetic parameters estimated by all other models are also presented in .
3.3. Phytotoxicity assessment
Following biodegradation of PAEs by Gordonia sp., the phthalate degraded samples were assessed for the toxicity removal by phytotoxicity assessment assay. Citrus arietinum L. (chickpeas) is a common plant seed used in phytotoxicity assessment due to its high sensitivity toward different xenobiotics [Citation38]. Germination index (GI) of chickpeas seeds was calculated to assess the toxicity removal of degraded PAEs in this study. Germination index (GI) of the seeds is widely used to assess if a medium contains noxious substances for germination and/or root growth of the seed. Although relative seed germination and relative root elongation index are commonly examined to better describe the germinability and root elongation, respectively, GI combines both these parameters for phytotoxicity assessment [Citation48]. The germinated seeds incubated in degraded and untreated/raw DMP, DBP, and DnOP samples at 1000 mg/L concentration along with that incubated in tap and distilled water as negative controls are depicted in . The results of GI % for germinated seeds are presented in , which reveals high GI values in case of distilled and tap water as they are generally free from any phytotoxicant. From the figure, no root was observed in case of raw/untreated DMP, DBP, and DnOP samples; the GI values were 1.75, 1.22, and 1.43, respectively. The low GI values indicate very high toxicity of the PAEs toward seed germination. In case of degraded PAEs sample, the maximum GI value of 77.06% was achieved in case of DMP, whereas the value was 57.31% for DBP. Very low GI of 37.96% was observed for DnOP. More than 50% GI value of a sample is regarded as nontoxic [Citation49]. Very high GI value (>50%) for the degraded DMP and DBP indicate that the water can be consumed safely for agricultural purposes.
Figure 8. Image of chickpea seeds soaked in (a) distilled water (b) tap water, (c - e) degraded and (f - h) raw/untreated DMP, DBP, and DnOP, respectively, after 48 h.

Table 6. Germination index values of treated and untreated phthalate samples along with that of distilled and tap water as negative controls.
From the results of phytotoxicity assessment using chickpea seeds, the Gordonia bacterial species demonstrates its ability to degrade PAEs to nontoxic forms which is beneficial for cleaning up of contaminated environments, including wastewater, sludge sediment, and soil. As a result, it holds significant potential for the bioremediation of phthalate-contaminated wastewater. Further studies aimed at demonstrating its ability to degrade other phthalates, such as DEP, BBP, and DEHP, in bioreactor systems, e.g. membrane bioreactor or continuous stirred tank bioreactor will establish scale up potential of the treatment process. Assessment of toxicity of the treated water by using Daphnia magna, Danio rerio, Vibrio fischeri, etc. can be done for more clarity on toxicity removal. In addition, future research could also include a comprehensive techno-economic study that assesses the cost-effectiveness of using Gordonia sp. for bioremediation compared to other physico-chemical treatment methods. This could provide valuable insights into the feasibility of scaling up the technology for large-scale industrial applications.
4. Conclusion
This study demonstrated that Gordonia sp. is capable of completely degrading DBP and DMP, even at high initial concentrations, with a high specific degradation rate. However, the degradation of DnOP was found to be low due to its inhibitory effect on the degradation and biomass growth of Gordonia sp. Among the different substrate inhibition models investigated in this study, the Teisser model was found to be the most suitable for describing the inhibitory effect of all the phthalate esters. The high GI (germination index) values observed for the degraded DBP and DMP indicated that the water was free from phytotoxicants and could be safely used for agricultural purposes. Further studies aimed at establishing the scale-up of the bioprocess can prove helpful for large-scale applicability of the organism to treat PAEs contaminated system.
Author contributions statement
Dipak Kumar Kanaujiya: Conceptualization, Methodology, Data curation, Validation, Investigation, Writing- Original Draft, Visualization.
Atharva More: Investigation, Methodology.
Ajay Kumar Chhantyal: Data curation, Validation.
Rama Karn: Methodology.
Kannan Pakshirajan: Conceptualization, Resources, Supervision, Funding acquisition Writing- Review and Editing, Visualization.
Acknowledgments
The authors are grateful to the Department of Science and Technology, Government of India, for funding (DST/TM/INDOUK/2K17/30(G)) this research work. Authors thank analytical facility at Department of Biosciences and Bioengineering, Indian Institute of Technology Guwahati, for carrying out sample analysis.
Disclosure statement
No potential conflict of interest was reported by the author(s).
Data availability statement
Raw data were generated at Department of Biosciences and Bioengineering, Indian Institute of Technology Guwahati. Derived data supporting the findings of this study are available from the corresponding author [Prof. Kannan Pakshirajan] [email protected] on request.
Additional information
Funding
References
- Wang W, Leung AOW, Chu LH, et al. Phthalates contamination in China: status, trends and human exposure-with an emphasis on oral intake. Environ Pollut. 2018;238:771–782. doi:10.1016/j.envpol.2018.02.088
- Tuan Tran H, Lin C, Bui XT, et al. Phthalates in the environment: characteristics, fate and transport, and advanced wastewater treatment technologies. Bioresour Technol. 2022;344:126249. doi:10.1016/j.biortech.2021.126249
- Crisp TM, Clegg ED, Cooper RL, et al. Environmental endocrine disruption: an effects assessment and analysis. Environ Health Perspect. 1998;106(suppl 1):11–56. doi: 10.1289/ehp.98106s111
- Marlatt VL, Bayen S, Castaneda-Cortès D, et al. Impacts of endocrine disrupting chemicals on reproduction in wildlife and humans. Environ Res. 2022;208:112584. doi:10.1016/j.envres.2021.112584
- Kotowska U, Kapelewska J, Sawczuk R. Occurrence, removal, and environmental risk of phthalates in wastewaters, landfill leachates, and groundwater in Poland. Environ Pollut 2020; 267:115643.
- Delbes G, Blázquez M, Fernandino JI, et al. Effects of endocrine disrupting chemicals on gonad development: mechanistic insights from fish and mammals. Environ Res. 2022;204:112040. doi:10.1016/j.envres.2021.112040
- Paluselli A, Fauvelle V, Galgani F, et al. Phthalate release from plastic fragments and degradation in seawater. Environ Sci Technol. 2019;53(1):166–175. doi: 10.1021/acs.est.8b05083
- Rathi BS, Kumar PS, Show PL. A review on effective removal of emerging contaminants from aquatic systems: current trends and scope for further research. J Hazard Mater. 2021;409:124413. doi: 10.1016/j.jhazmat.2020.124413
- Singh N, Dalal V, Mahto JK, et al. Biodegradation of phthalic acid esters (PAEs) and in silico structural characterization of mono-2-ethylhexyl phthalate (MEHP) hydrolase on the basis of close structural homolog. J Hazard Mater. 2017;338:11–22. doi:10.1016/j.jhazmat.2017.04.055
- Prasad B, Suresh S. Biodegradation of dimethyl phthalate ester using free cells, entrapped cells of Variovorax sp. BS1 and cell free enzyme extracts: a comparative study. Int Biodeterior Biodegrad. 2015;97:179–187. doi: 10.1016/j.ibiod.2014.11.004
- Surhio MA, Talpur FN, Nizamani SM, et al. Complete degradation of dimethyl phthalate by biochemical cooperation of the Bacillus thuringiensis strain isolated from cotton field soil. RSC Adv [Internet]. [cited 2023 May 19] 2014;4:55960–55966. Available from https://pubs.rsc.org/en/content/articlehtml/2014/ra/c4ra09465d
- Gao M, Xu Y, Dong Y, et al. Accumulation and metabolism of di(n-butyl) phthalate (DBP) and di(2-ethylhexyl) phthalate (DEHP) in mature wheat tissues and their effects on detoxification and the antioxidant system in grain. Sci Total Environ. 2019;697:133981. doi:10.1016/j.scitotenv.2019.133981
- Sarkar J, Chowdhury PP, Dutta TK. Complete degradation of di-n-octyl phthalate by Gordonia sp. strain Dop5. Chemosphere. 2013;90(10):2571–2577. doi: 10.1016/j.chemosphere.2012.10.101
- Das MT, Kumar SS, Ghosh P, et al. Remediation strategies for mitigation of phthalate pollution: challenges and future perspectives. J Hazard Mater. 2021;409:124496. doi: 10.1016/j.jhazmat.2020.124496
- Li X, Zhang Y, Xie Y, et al. Ultrasonic-enhanced Fenton-like degradation of bisphenol a using a bio-synthesized schwertmannite catalyst. J Hazard Mater. 2018;344:689–697. doi: 10.1016/j.jhazmat.2017.11.019
- Gu X, Qin N, Zhang P, et al. In-situ synthesis of {111}TiO2/Ti photoelectrode to boost efficient removal of dimethyl phthalate based on a bi-functional interface. Chem Eng J. 2021;422:129980. doi: 10.1016/j.cej.2021.129980
- Dueñas-Moreno J, Mora A, Cervantes-Avilés P, et al. Groundwater contamination pathways of phthalates and bisphenol A: origin, characteristics, transport, and fate – a review. Environ Int. 2022;170:107550. doi: 10.1016/j.envint.2022.107550
- Kanaujiya DK, Paul T, Sinharoy A, et al. Biological treatment processes for the removal of organic micropollutants from wastewater: a review. Curr Pollut Rep. 2019;5(3):112–128. doi: 10.1007/s40726-019-00110-x
- Zhang H, Lin Z, Liu B, et al. Bioremediation of di-(2-ethylhexyl) phthalate contaminated red soil by Gordonia terrae RL-JC02: characterization, metabolic pathway and kinetics. Sci Total Environ. 2020;733:139138. doi: 10.1016/j.scitotenv.2020.139138
- Zhang H, Zhao C, Na H. Enhanced biodegradation of phthalic acid esters’ derivatives by plasticizer-degrading bacteria (Burkholderia cepacia, archaeoglobus fulgidus, pseudomonas aeruginosa) using a correction 3d-qsar model. Int J Environ Res Public Health. 2020;17(15):1–17. doi: 10.3390/ijerph17155299
- Manzi HP, Zhang P, Zhang L, et al. Effect of dibutyl phthalate on microalgal growth kinetics, nutrients removal, and stress enzyme activities. Mar Environ Res. 2022;181:105741. doi: 10.1016/j.marenvres.2022.105741
- Zhao HM, Hu RW, Chen XX, et al. Biodegradation pathway of di-(2-ethylhexyl) phthalate by a novel Rhodococcus pyridinivorans XB and its bioaugmentation for remediation of DEHP contaminated soil. Sci Total Environ. 2018;640–641:1121–1131. doi: 10.1016/j.scitotenv.2018.05.334
- Zhao HM, Du H, Lin J, et al. Complete degradation of the endocrine disruptor di-(2-ethylhexyl) phthalate by a novel Agromyces sp. MT-O strain and its application to bioremediation of contaminated soil. Sci Total Environ. 2016;562:170–178. doi: 10.1016/j.scitotenv.2016.03.171
- Benjamin S, Kamimura N, Takahashi K, et al. Achromobacter denitrificans SP1 efficiently utilizes 16 phthalate diesters and their downstream products through protocatechuate 3,4-cleavage pathway. Ecotoxicol Environ Saf. 2016;134:172–178. doi: 10.1016/j.ecoenv.2016.08.028
- Shariati S, Ebenau-Jehle C, Pourbabaee AA, et al. Degradation of dibutyl phthalate by paenarthrobacter sp. Shss isolated from Saravan landfill, Hyrcanian Forests, Iran. 2022. [[cited 2023 May 19]]. Available from Biodegradation [Internet]. 33(1):59–70. https://link.springer.com/article/10.1007/s10532-021-09966-7.
- Wang Y, Zhan W, Ren Q, et al. Biodegradation of di-(2-ethylhexyl) phthalate by a newly isolated Gordonia sp. and its application in the remediation of contaminated soils. Sci Total Environ. 2019;689:645–651. doi: 10.1016/j.scitotenv.2019.06.459
- Tao Y, Li H, Gu J, et al. Metabolism of diethyl phthalate (DEP) and identification of degradation intermediates by Pseudomonas sp. DNE-S1. Ecotoxicol Environ Saf. 2019;173:411–418. doi: 10.1016/j.ecoenv.2019.02.055
- Song X, Zhang Z, Dai Y, et al. Biodegradation of phthalate acid esters by a versatile PAE-degrading strain Rhodococcus sp. LW-XY12 and associated genomic analysis. Int Biodeterior Biodegrad. 2022;170:105399. doi: 10.1016/j.ibiod.2022.105399
- Xu Y, Zhao J, Huang H, et al. Biodegradation of phthalate esters by Pantoea dispersa BJQ0007 isolated from Baijiu. J Food Compost Anal. 2022;105:104201. doi: 10.1016/j.jfca.2021.104201
- Li F, Liu Y, Wang D, et al. Biodegradation of di-(2-ethylhexyl) phthalate by a halotolerant consortium LF [Internet]. PLoS One. 2018;13(10):e0204324. [cited 2023 May 24]. Available from: https://journals.plos.org/plosone/article?id=10.1371/journal.pone.0204324.
- Kanaujiya DK, Sivashanmugam S, Pakshirajan K. Biodegradation and toxicity removal of phthalate mixture by Gordonia sp. in a continuous stirred tank bioreactor system. Environ Technol Innov. 2022;26:102324. doi: 10.1016/j.eti.2022.102324
- Zagrodnik R. Optimization of hydrogen production by co-culture of clostridium beijerinckii and rhodobacter sphaeroides Bacteria. Adv Sci Technol [Internet]. 2014;93:90–95. [[cited 2023 May 20]]. Available from https://www.scientific.net/AST.93.90
- Kanaujiya DK, Chhantyal AK, Pugazhenthi G, et al. A novel hybrid system for continuous biodegradation and toxicity removal of low molecular weight phthalates. J Environ Chem Eng. 2023;11(3):109983. doi: 10.1016/j.jece.2023.109983
- Kanaujiya DK, Pakshirajan K. Two liquid phase partitioning bioreactor system for toxicant free water production from phthalates contaminated aqueous medium. J Clean Prod. 2022;378:134428. doi: 10.1016/j.jclepro.2022.134428
- Rigo A, Plastino AR, Casas M, et al. Anomalous diffusion coupled with Verhulst-like growth dynamics: exact time-dependent solutions. Phys Lett A. 2000;276(1–4):97–102. doi: 10.1016/S0375-9601(00)00639-3
- Peleg M. A new look at models of the combined effect of temperature, pH, water activity, or other factors on microbial growth rate. Food Eng Rev. 2022;14(1):31–44. doi: 10.1007/s12393-021-09292-x
- Sharma A, Mukherjee S, Reddy Tadi SR, et al. Kinetics of growth, plantaricin and lactic acid production in whey permeate based medium by probiotic Lactobacillus plantarum CRA52. LWT. 2021;139:110744. doi: 10.1016/j.lwt.2020.110744
- Kanaujiya DK, Pakshirajan K. Mass balance and kinetics of biodegradation of endocrine disrupting phthalates by Cellulosimicrobium funkei in a continuous stirred tank reactor system. Bioresour Technol. 2022;344:126172. doi: 10.1016/j.biortech.2021.126172
- Rohit SG, Jyoti PK, Subbi RRT, et al. Kinetic modeling of hyaluronic acid production in palmyra palm (Borassus flabellifer) based medium by Streptococcus zooepidemicus MTCC 3523. Biochem Eng J. 2018;137:284–293. doi: 10.1016/j.bej.2018.06.011
- Loh WLC, Huang KC, Ng HS, et al. Exploring the fermentation characteristics of a newly isolated marine bacteria strain, Gordonia terrae TWRH01 for carotenoids production. J Biosci Bioeng. 2020;130(2):187–194. doi: 10.1016/j.jbiosc.2020.03.007
- Sowani H, Deshpande A, Gupta V, et al. Biodegradation of squalene and n-hexadecane by Gordonia amicalis HS-11 with concomitant formation of biosurfactant and carotenoids. Int Biodeterior Biodegrad. 2019;142:172–181. doi: 10.1016/j.ibiod.2019.05.005
- Nahurira R, Ren L, Song J, et al. Degradation of Di(2-Ethylhexyl) phthalate by a novel gordonia alkanivorans strain YC-RL2. Curr Microbiol [Internet]. 2017;74(3):309–319. [cited 2022 Jun 22]. Available from: https://link.springer.com/article/10.1007/s00284-016-1159-9.
- Kolb SA, O’Loughlin EJ, Gsell TC. Data on the characterization of phthalate-degrading bacteria from Asian carp microbiomes and riverine sediments. Data Brief. 2019;25:104375. doi: 10.1016/j.dib.2019.104375
- Xu Y, KAHM M, Wang X, et al. Biodegradation of phthalate esters by Paracoccus kondratievae BJQ0001 isolated from Jiuqu (Baijiu fermentation starter) and identification of the ester bond hydrolysis enzyme. Environ Pollut. 2020;263:114506. doi: 10.1016/j.envpol.2020.114506
- Huang H, Zhang XY, Chen TL, et al. Biodegradation of structurally diverse phthalate esters by a newly identified esterase with catalytic activity toward Di(2-ethylhexyl) phthalate. 2019. [[cited 2023 May 21]]. Available from J Agric Food Chem [Internet]. 67(31):8548–8558. https://pubs.acs.org/doi/full/10.1021/acs.jafc.9b02655.
- Shariati S, Pourbabaee AA, Alikhani HA, et al. Biodegradation of DEHP by a new native consortium An6 (Gordonia sp. and Pseudomonas sp.) adapted with phthalates, isolated from a natural strongly polluted wetland. Environ Technol Innov. 2021;24:101936. doi: 10.1016/j.eti.2021.101936
- Baskaran D, Paul T, Kannan P, et al. Batch degradation of trichloroethylene using oleaginous Rhodococcus opacus in a two-phase partitioning bioreactor and kinetic study. Bioresour Technol Rep. 2020;11:100437. doi: 10.1016/j.biteb.2020.100437
- Enaime G, Baçaoui A, Yaacoubi A, et al. Phytotoxicity assessment of olive mill wastewater treated by different technologies: effect on seed germination of maize and tomato. Environ Sci Pollut Res. 2020;27(8):8034–8045. doi: 10.1007/s11356-019-06672-z
- Tiquia SM, Tam NFY, Hodgkiss IJ. Effects of composting on phytotoxicity of spent pig-manure sawdust litter. Environ Pollut. 1996;93(3):249–256. doi: 10.1016/S0269-7491(96)00052-8