ABSTRACT
Compared with other biomass sources, the use of algae as a raw material to prepare biochar (BC) has important advantages including safety, high yield and economy. The protein content of algae cells is as high as 3.2 mg DCW/L, and the graphitic-N and N-O functional groups generated by the pyrolysis of proteins could effectively activate free radicals. Combined with the generated pore structure, the electron transfer/exchange capability was enhanced, which is conducive to improving its catalytic performance. Algae as a natural N source, the manuscript analyzed the surface properties and physicochemical properties of algae-based BC, and investigated its degradation effect on organic/inorganic pollutants in wastewater. Subsequently, the effect of nitrogen-doped BC on the adsorption/catalysis capacity was discussed. Finally, the directed preparation of algae-based BC applied in different scenarios was summarized. Algae-based BC has the property of N doping, which broadens its application efficiency in the environmental field. Overall, this manuscript reviews how to achieve efficient utilization of algae-based BC in wastewater.
GRAPHICAL ABSTRACT
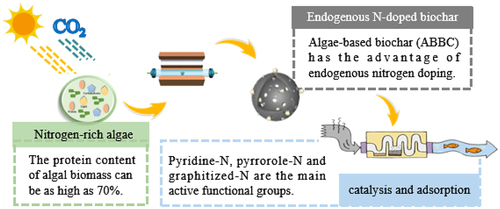
Highlights
Toxin type and domain sequence affect accumulation of recombinant immunotoxins.
Transient expression in plant cell packs and intact plants correlates well.
IC50 values of toxicity correlate with the cell surface receptor concentration
1. Introduction
1.1. Algae
Algae are the first photosynthesizing living organisms on the primitive earth and according to different metabolic modes, can be classified into photoautotrophs, heterotrophs, mixotrophs, and photoheterotrophs [Citation1]. Algae absorb a large amount of carbon dioxide (CO2) to produce nearly half of the oxygen (O2) in the atmosphere during their growth process, which is considered as an effective biological carbon capture technology () [Citation2,Citation3]. In particular, autotrophic or photosynthetic algae have a remarkable CO2 fixation capacity, which is even 50 times higher than that of terrestrial plants () [Citation4]. It is estimated that algae consume about 2.0 kg CO2 for accumulating 1.0 kg biomass during their growth process [Citation5,Citation6]. In addition, algae have good carbon dioxide tolerance. Chlorella, e.g. can thrive in carbon dioxide with a concentration as high as 40% [Citation7]. The growth process of algae has a loose demand for nutrients and environment, and mainly includes sunlight, organic or inorganic carbon sources, fresh water, seawater, or wastewater [Citation8,Citation9]. In particular, abundant nitrogen, phosphorus, organic and inorganic matter in wastewater are used as nutrient sources, which greatly reduce the production cost of algae [Citation9,Citation10]. Compared to most terrestrial plants, algae have the advantage of being independent of soil requirement and are able to grow in a wide range of aquatic environments [Citation11,Citation12]. More importantly, algae have high photosynthetic efficiency, strong carbon sequestration capability, and low nutrient requirements, which make them exhibit a higher yield even with a short life cycle and a large number of biochemical compositions, such as carbohydrates, proteins, lipids rich in pigments, secondary metabolites, and polysaccharides [Citation13–15]. Therefore, how to rationally utilize biomass resources, the use of algae for bioremediation and the conversion of them into value-added products such as bio-oil [Citation1], soil amendments [Citation16], poultry feed [Citation17], health-care products [Citation18], and cosmetics [Citation19], which is the issue worth considering with the backdrop of the gradual shortage of resources. The preparation of biochar from algae has attracted the attention of scholars from all over the world [Citation20].
Figure 1. (a) carbon enrichment mechanism and photosynthetic process of microalgae; (b) Species and percentage of microalgae commonly used for carbon fixation. Reprinted and modified with permissions from Refs [Citation2].
![Figure 1. (a) carbon enrichment mechanism and photosynthetic process of microalgae; (b) Species and percentage of microalgae commonly used for carbon fixation. Reprinted and modified with permissions from Refs [Citation2].](/cms/asset/530c5031-ca0b-42b1-ab96-ac5fecd64474/kbie_a_2252157_f0001_oc.jpg)
1.2. Biochar
Biochar (BC) is a kind of porous carbon material, which is thermally obtained through transformation from biomass under low temperature and anoxic conditions [Citation21]. The word, biochar, comes from two words, biomass (bio) and charcoal (char) [Citation22]. It is well-known that the raw material source of BC consists of a wide range of biomass such as fruit waste [Citation23], sawdust [Citation24], wood [Citation25], rice straw [Citation26], and other wastes. Biomass can undergo a series of reactions such as isomerization, dehydration, decarboxylation, depolymerization, and carbonization to generate BC during the pyrolysis process. The advantages of insolubility, stability, porosity, and aromatization of BC play an important role in controlling and remediating harmful environmental pollutants [Citation27]. It is recognized that the better hydrophilicity of the surface of BC is conducive to the adsorption of heavy metals, while the excellent hydrophobicity is favorable for the adsorption of organic pollutants [Citation27]. In particular, in recent years, BC has attracted more and more attention for the adsorption of heavy metals, pesticides, antibiotics, microplastics, and organic pollutants in water through different mechanisms such as electrostatic adsorption, physical adsorption, and hydrophobic force [Citation28–31]. The adsorption performance of BC is closely related to its surface parameters, such as specific surface area (SSA), surface charge, pore size, and type of functional group, while these physicochemical properties vary with the preparatory conditions and biomass sources [Citation28–31]. The preparation of BC has mainly conducted the form of thermochemical transformation (pyrolysis, thermal cracking, and hydrothermal carbonization) by adjusting various pyrolysis parameters (pyrolysis temperature, reaction time, and residence time) and/or by post-treatment (modification and activation) to improve its physicochemical properties, thereby increasing its adsorption/catalytic capacity.
However, only a handful studies have focused on the surface characteristics of algae BC, including surface morphology, chemical composition and so on. In particular, information about the correlation between the direct preparation and the application of algal BC is scarce in literature. Yu et al. [Citation32] introduced the influence of different algae (used as raw materials) on the production of BC, summarized their advantages and disadvantages, and finally, confirmed their application potential for adsorption. Gan et al. [Citation33] discussed the main morphology of algal BC, however the application of specific algal BC for different scenarios and uses was not comprehensively summarized. Moreover, the characteristics and superiorities of algal BC have not been explored in detail.
Therefore, this review analyzes the surface morphology of algal BC, the chemical combination of algal biomass components, and the preparation strategy of biochar, thus aiming at the establishment of a particular preparation strategy for algal BC and its subsequent application. Furthermore, this review provides new ideas for the transformation of waste algae/algal residue from different sources, directional preparation of functional algal BC, and their application prospects. In addition, the specific chemical properties and advantages of ABBC are discussed for improving its targeted application in the future.
2. Preparation and characteristics of algal BC
2.1. Biochemical composition of algae
Due to higher photosynthesis, algae contain abundant hydrocarbons including pigments, secondary metabolites, polysaccharides, and other bioactive substances, such as phenols, aldehydes, ketones, carboxylic acids and derivatives [Citation34,Citation35]. Moreover, proteins (6–70%), lipids (2–50%), and carbohydrates (4–64%) are the main components of algae ()[Citation15,Citation36]. Biochemical components of algae remain at a dynamic equilibrium and can be cultured or genetically modified according to various applications () [Citation5]. Furthermore, the pyrolysis temperature of biochemical components is the main factor affecting the surface properties of ABBC. It has been reported that the dehydration of biomass occurs at a pyrolysis temperature of 25–200°C. In addition, carbohydrates and proteins are decomposed at 200–500°C, while lipids are dissolved at 350–550°C. Other components of algal biomass are decomposed at 550–800°C [Citation36,Citation37]. The hypothetical path of product changes during the pyrolysis process, as shown in [Citation15,Citation36].
Figure 2. Regulatory mechanisms for lipid formation in algal cells. Reprinted and modified with permissions from Refs [Citation5].
![Figure 2. Regulatory mechanisms for lipid formation in algal cells. Reprinted and modified with permissions from Refs [Citation5].](/cms/asset/233113f9-e322-48a5-8047-c4cef01ff85c/kbie_a_2252157_f0002_oc.jpg)
Figure 3. Hypothetical pathway for pyrolysis of algal components. Reprinted and modified with permissions from refs [Citation36].
![Figure 3. Hypothetical pathway for pyrolysis of algal components. Reprinted and modified with permissions from refs [Citation36].](/cms/asset/04280d30-0021-4745-9f94-4f9c9bce1bf6/kbie_a_2252157_f0003_oc.jpg)
Table 1. Main chemical components of algae.Reprinted and modified with permissions from refs [Citation15].
It is worth noticing that the chemical transformation of protein has a great effect on the adsorption performance of ABBC. Tan et al. [Citation6] found that the prepared BC had a good adsorption capacity (287.89 mg/g) for sulfamethoxazole, which was due to the high content (70%) of protein decomposition in algal cells. The reason is that proteins can be decomposed into aromatic hydrocarbons (benzene, phenol, m-cresol, toluene, xylene, and nitriles) and N-heterocyclic compounds (graphitized-N and oxidized-N) through pyrolysis [Citation36,Citation37]. The amino acid structure becomes pyridine-N and pyrrorole-N due to deamination or dehydrog [Citation38]. The decomposition, deoxidation and deamination of intermediate products can accelerate the formation of olefin [Citation39]. Furthermore, significant decomposition of endogenous protein can produce nitrogen-containing functional groups that are attached to the surface of algal BC (). Surface chemical properties will affect its adsorption and catalytic properties, which will be described in the following discussion.
Algal cells store carbohydrates in the form of polysaccharides and oligosaccharides, which exist in the form of anhydrous sugars and furfural in the processes of deoxygenation, decarbonization, dehydration, and lysis through high-temperature pyrolysis [Citation40]. Various by-products including ketones, aldehydes, acids, and alcohols are produced primarily due to the decarboxylation and deoxidation. These products can be converted into olefin compounds through deep cracking, whereas some of them can be converted into aromatic hydrocarbons using cyclic reactions () [Citation36,Citation41].
Pyrolysis in algae cells is mainly represented by polysaccharides and oligosaccharides. During the pyrolysis process, triglycerides first undergo hydrolysis/cleavage reactions to generate long-chain fatty acids due to the cleavage of acyl chains in the glycerol molecule () [Citation36,Citation41]. Therein, the decarboxylation reaction of saturated fatty acids can produce ketones, alkanes, and alkenes. Unsaturated fatty acids will be converted to volatile substances (CO2, and CO), fatty acids, hydrocarbons, alkanes, and alkenes due to the fracture of C=C bond at the pyrolysis temperature of less than 400°C [Citation42]. Steroids are carbonized through pyrolysis to form polycyclic aromatic hydrocarbons and other stable small molecules [Citation43]. The pyrolysis products of lipids are mainly alkanes, alkenes, and aromatic compounds [Citation41].
In summary, various products formed by the decomposition of carbohydrates, proteins, and lipids in algal cells will be loaded onto the surface of BC in the form of functional groups (). The surface properties will be regulated by intentionally changing the preparation conditions, which will ultimately affect its adsorption/catalytic performance.
2.2. Pyrolysis process for preparing algal biochar
Compared with traditional biotechnologies such as fermentation and anaerobic digestion, thermochemical conversion technologies including pyrolysis, hydrothermal, microwave pyrolysis, and torrefaction are currently the main methods for preparing BC () [Citation22,Citation44]. These processes are carried out under different conditions and media of heat treatment, such as different biomass sources, pyrolysis temperatures, and residence times, which affect the yield of BC and its surface physicochemical properties. The surface of BC has a unique morphology and properties, which can be tailored by mastering the preparation method according to the characteristics of algal biomass so as to enhance the selective removal of pollutants () [Citation22].
Figure 4. Chemical pyrolysis technology for producing BC from algal biomass. Reprinted and modified with permissions from refs [Citation22].
![Figure 4. Chemical pyrolysis technology for producing BC from algal biomass. Reprinted and modified with permissions from refs [Citation22].](/cms/asset/3025d9ee-e262-4a9b-87b5-86b6771d7610/kbie_a_2252157_f0004_oc.jpg)
Table 2. Preparation of algal BC by different modification methods and their effects on pollutant remediation. Reprinted and modified with permissions from refs [Citation22].
2.2.1. Pyrolysis
Pyrolysis is a conversion technology for preparing BC through heating (300–1000°C) under the protection of inert gases, and involves the processes of water loss, deoxidation, and lysis of organic matter [Citation32,Citation45]. According to the duration of pyrolysis and heating rate, it can be divided into fast and slow pyrolysis processes. Fast pyrolysis is a process within the temperature range of 400–600°C with a heating rate of more than 100°C/s and is usually used in the production of bio-oil [Citation46]. Slow pyrolysis is also called carbonization since it attains high yield of BC below 400°C with the heating rate of 0.1–1.0°C/s. The chemical properties of the surface of BC are closely related to the pyrolysis parameters (temperature, heating rate, and residence time) [Citation47]. When the temperature is lower than 300°C, although the surface morphology of BC does not change significantly, the rate of production of carbon can be as high as 15–43% [Citation39]. When the temperature is higher than 350°C, the rate of production of carbon will decrease due to the polymerization of intermediate products [Citation39]. When the temperature is within 500–700°C, the gas escaping from the pyrolysis process leaves abundant pore structures on the surface of BC [Citation6]. When the pyrolysis temperature is higher than 700°C, the degree of graphitization of BC increases [Citation48]. Jung et al. [Citation49,Citation50] found that the adsorption capacity of phosphate of ABBC decreased with the increase of pyrolysis temperature, resulting from the pore structure blocked by the intermediates and the decrease of SSA at high temperature. High-temperature pyrolysis of algal biomass for preparing BC can change its physical and chemical properties and the pyrolysis conditions will also change its structure and composition, thus affecting the adsorption/catalytic capacity and mechanism [Citation51–53].
2.2.2. Hydrothermal liquefaction
Hydrothermal liquefaction (HTL) is one of the main methods for rapid treatment of biomass. It takes water as the reaction medium and involves a series of structural and biochemical transformations of biomass [Citation51]. Biomass is usually rapidly converted into BC at the temperature of 180–250°C and pressure of 2 MPa, whereas bio-oil and biogas are produced as side-products due to the depolymerization of by-products (organic matter) [Citation54,Citation55]. Biogas is mainly composed of hydrogen, carbon dioxide, carbon monoxide, and methane, whose contents are 23.7, 19.1, 22.8, and 25.8%, respectively [Citation56]. The significant advantage of HTL technology is the use of environment-friendly water as a medium [Citation51]. The density, surface tension, and static dielectric constant of water would decrease in an environment with high temperature and high pressure, so that the chemical bonds inside the biomass cells are easily broken in water [Citation51]. Compared with other dry pyrolysis technologies, HTL can save the pretreatment time of biomass [Citation57]. The operation and the equipment for HTL are simple, and the process can be carried out in a batch autoclave, a large batch reactor, or a continuous reactor [Citation51].
2.2.3. Microwave pyrolysis
Microwave pyrolysis is a homogeneous heating process, in which the electromagnetic waves penetrate the walls of containers and directly heat the biomass inside. It is considered to be an efficient and suitable thermochemical conversion technology for the disposal of large biomass particles [Citation15,Citation58]. Different from traditional pyrolysis, the presence of water in raw material is conducive to the microwave pyrolysis technology, because water has a strong ability to absorb microwaves, which can effectively improve the heating efficiency [Citation36]. In addition, microwave absorbers and/or catalysts can be used during the microwave pyrolysis process for synthesizing certain products [Citation59]. In this process, a large amount of escaped biogas not only increases the surface porosity of BC, but also reduces the diversity of functional groups [Citation14]. In general, microwave heating technology can obtain BC with higher yield. However, the yield decreases with the increase in temperature, shortening of residence time, and increase in microwave power [Citation60,Citation61]. Microwave pyrolysis is an environment-friendly technology that prevents the use of toxic and harmful chemicals.
2.2.4. Torrefaction
Torrefaction is one of the main pathways to convert biomass into BC, which is generally divided into dry and wet processes. Dry torrefaction is also called the mild pyrolysis or low-temperature pyrolysis, and usually occurs at the temperatures of 200–300°C under nitrogen atmosphere [Citation62]. Various vital parameters of BC prepared using dry torrefaction such as calorific value, energy density, and carbon content are improved due to the discharge of water [Citation63,Citation64]. Carbohydrates, proteins, and lipids are retained in the algae cells. Meanwhile, the torrefaction process will disrupt their internal structures and have an impact on the surface morphology of BC. The diversity of functional groups (hydroxyl and carboxyl), hydrophilicity/hydrophobicity, porosity, and by-products (benzene, and acetic acid) generated on the surface of BC could be changed during this process [Citation65]. Chen et al. [Citation53] demonstrated that the type of elements and functional groups on the surface of BC were improved by torrefaction, thereby promoting the adsorption performance of BC. It is worth noticing that the torrefaction process assisted by microwaves improves the heating efficiency, so that better heat transfer is obtained, which can further promote the carbonization of biomass [Citation66,Citation67]. Gan et al. [Citation33] reported that the microwave-assisted biomass carbonization can improve the wear resistance, absorption of moisture, and carbon yield of BC. Microwave-assisted torrefaction technology with high operational feasibility not only improves the energy efficiency and reduces costs, but also can be used for large-scale applications [Citation63,Citation68].
2.3. Activation of algal biochar
The activation process is also important for the direct preparation of adsorbents/catalysts. The purpose of the modification is to improve the physicochemical properties of the surface of BC and further change its adsorption/catalytic properties, including SSA, pore volume, charge, elements, and functional groups [Citation69]. The activation process is mainly divided into physical and chemical activations [Citation34].
2.3.1. Physical activation
Physical activation mainly refers to the processing of BC to increase its surface porosity through some activation means (CO2 and steam) within the pyrolysis temperature of 200–900°C. Physical activation is safer and more environment-friendly than chemical activation [Citation70,Citation71]. Carbon dioxide (CO2) is a common activator, mainly used to guide the oxidation reaction, and does not participate in the chemical changes of biomass pyrolysis process due to its low activity. Therefore, it is also conducive to the formation of pores on the surface of BC [Citation72]. In addition, various gases such as air, oxygen, and ozone are also commonly used in the physical activation process. However, because air and oxygen are combustible or oxidizing gases, high temperature will lead to intense combustion on the surface of BC, due to which, the internal porosity will not be achieved in the biochar [Citation72]. For ozone activation, the process can not only provide acidic functional groups on the surface of BC, but can also enhance the cation exchange capability, which is conducive to the adsorption of polar pollutants [Citation72].
Steam activation is implemented within the temperature of 200–800°C to mainly increase the adsorption capacity. This process contributes to the increase in carbon content and the formation of mesoporous and microporous structures on the surface of BC due to the release of volatile gases [Citation73,Citation74]. For example, Borchard et al. [Citation75] reported that the carbon content of BC increased by 10% (w/w) through the steam activation process. As tar and other components can be excluded in the process of steam activation, H and O contents in BC decrease, which is manifested by the decrease of H/C and O/C ratios [Citation76]. Therefore, the steam activation process also contributes to the increase in aromaticity and the decrease in polarity of BC [Citation77].
2.3.2. Chemical activation
Compared with physical activation, there are more chemical activation methods, and include oxidation, amination, sulfonation, and impregnation. Among them, acid (HNO3, HCl, H3PO4, and KMnO4), alkali (KOH and NaOH), and oxidation (H2O2) treatments are the most popular ones [Citation34]. The choice of activation method is determined according to the application of BC. In general, alkaline activators facilitate the generation of positive charges on the surface of BC, while acidic activators contribute to the appearance of negative charges. Researchers can select different activators to promote the affinity between BC and pollutants according to their valences, so as to obtain satisfactory adsorption/catalytic efficiency [Citation78]. Moreover, porosity is a critical factor for the adsorption/catalytic performance. Trakal et al. [Citation79] found that, after KOH activation, the pore volume of BC increased from 0.01 mL/g to 8.74 mL/g. As a liquid activator, ZnCl2 can be uniformly mixed with biomass. It penetrates the biomass at low temperature, which also promotes the expansion of pore volume in BC [Citation80]. It has been reported that BC activated by K2CO3 exhibits excellent adsorption performance due to the increase in SSA. Zhu et al. [Citation81] activated BC using K2CO3 and found that the SSA of BC increased to 815 m2/g, rendering BC the capacity of 130.7 mg/g for naphthene’s adsorption. The main advantage for the use of H2O2 as an activator is not just the low cost, but it also produces the degradation products of H2O and O2, making it an environment-friendly means of activation. Some studies have shown that the affinity of H2O2-activated BC for herbicides cyfluoxalol and clomazone was as high as 99% [Citation82]. The introduction of metals can also improve the adsorption capacity of BC. The ternary complexes formed by endogenous calcium minerals in ABBC through a metal bridging mechanism enhanced the adsorptions of rhodamine B, methylene blue, Congo red, and methyl orange [Citation83].
2.4. Characteristics of algae-based BC
BC exhibits different properties (pore structure, aromaticity, polarity, hydrophilicity/hydrophobicity, surface charge, and pH) that determine its adsorption/catalysis efficiency due to the different biomass sources and preparation methods.
2.4.1. Porous structure
SSA, BET, pore volume, and pore size are the necessary parameters to investigate the adsorption performance of BC. In general, with activation/pretreatment, these parameters of BC are lower. The BET of Chlorella sp. BC obtained by wet torrefaction method is 2.66 m2/g [Citation84], whereas the BET of Spirulina sp. BC prepared by hydrothermal liquefaction method is 1.56 m2/g [Citation85]. However, SSA, BET, and pore volume can be modified, and the most direct approach to do so is to increase them with the increase in pyrolysis temperature. For example, the SSA of Spirulina sp. BC increases to 2.63 m2/g at the pyrolysis temperature of 750°C [Citation86]. The BET of Chlorella sp. BC is 266 m2/g, whereas the pore volume is 0.61 cm3/g at the pyrolysis temperature of 450°C for 60 min [Citation27]. Compared with activated carbon, the capacity of activated BC for the adsorption of P-nitrophenol became higher by 140%, while the adsorption capacity reached the value of 204.8 mg/g [Citation87]. Moreover, it has also been reported that the adsorption capacity of BC for aromatic hydrocarbon pollution is positively correlated with the SSA and the correlation coefficient is 0.897 [Citation88]. It is worth noticing that, when the pore size greatly exceeds the molecular diameter of pollutants, the pores act as diffusion channels and greatly reduce the adsorption capacity. Melanie et al. [Citation89] found that, during the adsorption process of aromatic hydrocarbons, the diffusion coefficient of carbon materials with a pore size of 5.0 nm was twice that of the pore size of 1.8 nm. These results further prove that the appropriate pore space can promote the adsorption process [Citation90].
2.4.2. Aromaticity and polarity of BC
The carbonization process of algal biomass involves deoxygenation, decarbonization, dehydration, cracking, and other reactions, which will be deposited on the surface of BC in the form of carbon, hydrogen, nitrogen, oxygen, sulfur, and certain other elements [Citation68]. Generally speaking, the pyrolysis temperature lies within the range of 550–950°C with the heating rate of about 60°C/min, which is conducive to increasing the carbon content under the oxygen-limited conditions [Citation15,Citation91]. The carbon content increases with the increase in pyrolysis temperature, reaching the value of 41.0–70.0 wt.% [Citation13]. However, the contents of hydrogen and oxygen in the ABBC will greatly decrease [Citation33], which is expressed due to the decreased H/C and O/C ratios [Citation53,Citation68]. Therein, the H/C ratio decreases faster than the O/C ratio. Therefore, it has been reported that the increase in pyrolysis temperature contributes to the improvement of aromaticity and the reduction of polarity of ABBC. In addition, the increase of pyrolysis temperature enhances the stability of BC and increases the number of oxygen-containing functions, promoting the adsorption of nonpolar organic pollutants [Citation86,Citation92,Citation93]. It has been reported that BC prepared from Spirulina as the biomass source showed lower H/C and O/C ratios. The prepared BC possessed excellent aromatability and hydrophobicity, and its adsorption capacity for tetracycline reached the value of 132.8 mg/g [Citation86]. However, the high O/C ratio in BC caused by low pyrolysis temperature enhances its adsorption capability for heavy metals [Citation27]. For example, the adsorption capacities of Cr(VI), Zn(II), and Ni(II) in BC prepared using this method were found to be 15.94 mg/g, 17.62 mg/g, and 24.76 mg/g, respectively, which were twice those achieved using the BC obtained by other methods [Citation27]. In short, the adsorption capacity of ABBC can be adjusted through different pyrolysis methods to change its aromaticity, polarity, and porosity.
2.4.3. Surface functional groups
The functional groups distributed on the surface of BC largely determine its hydrophobicity/hydrophilicity, surface charge, and therefore, affect its adsorption/catalytic performance. According to the elemental compositions of the functional groups, it can be roughly divided into oxygen-containing, nitrogen-containing, and sulfur-containing functional groups. Oxygen-containing functional groups are the most widely studied and many reports have confirmed that high content of oxygen-containing functional groups in BC can promote its adsorption capacity [Citation94,Citation95]. Therein, hydroxyl and carboxyl groups are important functional groups that determine the hydrophobicity and adsorption mechanism of BC. The increase in temperature during pyrolysis will destroy the contents and existence of hydroxyl and carboxyl groups. Zhang et al. [Citation96] found that there were fewer acidic functional groups (such as hydroxyl, and carboxyl), while the yield of basic functional groups (such as amine groups) increased in BC obtained at higher pyrolysis temperatures. In general, the reduction of oxygen-containing functional groups will increase the hydrophobicity of the surface of BC, which results in the resistance to humidity [Citation90]. Therefore, it is helpful for the adsorption of hydrophobic organic pollutants (antibiotics, dyes and polycyclic aromatic hydrocarbons) [Citation92]. Similarly, high content of oxygen functional groups can increase the hydrophilicity of BC, which can improve the adsorption capacity of inorganic pollutants such as heavy metals. In addition, other acidic/basic functional groups (carboxyl, lactone and phenolic groups, sulfur-oxygen functional groups, and nitrogen-oxygen functional groups) in BC also participate in the chemical bonding between ABBC and pollutants [Citation93]. It has been reported that nitrogen-oxygen functional groups can significantly enhance the polarity of BC, whereas sulfur-oxygen functional groups can adjust its adsorption capacity [Citation90].
2.4.4. Other properties
The surface of the ABBC generally exhibits a distribution of C, H, and O elements, while various other inorganic elements such as K, Ca, Na, and Mg are also distributed on it. After high temperature pyrolysis of these inorganic elements, inorganic salts will be formed on the surface of BC, which renders it a higher pH value (pH > 7). Inorganic salts can be used as endogenous pore-forming agents to create the pores and channels in BC [Citation48]. In addition, pH value is the most obvious factor affecting the properties of BC, whereas the effect on the surface charge is the most obvious. When the pH value of the solution is higher than the zero charge (pHpzc), the negative charge on the surface of BC is dominant. Under these conditions, it becomes easier to adsorb metal cations [Citation97]. When the pH < pHpzc, the positive charge is dominant, and BC can easily adsorb metal anions [Citation90]. With the increase of pyrolysis temperature, the decrease in functional groups such as -COOH and -OH will be accompanied by a decrease in negative charge on the surface of BC [Citation98].
3. Advantages and applications of algal biochar
3.1. Catalyst
Advanced oxidation technology is widely used in wastewater treatment. Free radicals (hydroxyl radical (˙OH), sulfate radical (SO4˙ˉ) or singlet oxygen (1O2)) produced by oxidation process are the main factors for the degradation of organic pollutants () [Citation37]. Generally, ABBC has abundant surface functional groups and larger SSA, especially the effect of endogenous nitrogen impurity, so that its surface has more flexible electronic properties and stronger electron transfer/exchange capability. Due to this, the ABBC exhibits efficient degradation of organic pollutants [Citation36,Citation98]. Hung et al. [Citation99] focused on the removal of 4-nonylphenol (4-NP) from marine sediments. Using red algal BC (RAB) as the catalyst, it was found that Ca2+ ions effectively activated sodium percarbonate and generated many active free radicals (). Moreover, various oxygen-containing functional groups (O-H, C-O-C, C-O-, -COOH, and -COO-) embedded on RAB surface not only provided more catalytic sites, but also formed OH groups with H2O2 to further accelerate electron transfer and promote the degradation of 4-NP (). Compared with OHˉ, SO4ˉ has the advantages of high REDOX potential and long half-life, which can be produced by the excitation of peroxydisulfate (PDS) or peroxymonomer (PMS) [Citation101,Citation102]. Moreover, the excitation method is relatively simple, which can be realized mainly by heating, ultraviolet irradiation, and addition of transition metals () [Citation100,Citation103,Citation104]. Ho et al. [Citation48] prepared an efficient catalyst of in situ nitrogen-doped PDS using Spirulina residue BC (SDBC) as raw material. SDBC is pyrolyzed at high temperature for 45 minutes to form an N-C-based complex, which can achieve 100% catalytic degradation of sulfamethoxazole (SMX). This is due to the fact that SDBC carbonized at high temperature has large SSA, which facilitates electrons to shuttle between SMX and PDS, thus exhibiting good electrical conductivity () [Citation48]. Furthermore, the multi-dimensional pore structure provides more contact sites for the interaction between pollutants and catalysts, realizing the efficient catalytic degradation of SMX using SDBC through π-π interaction between C=C pollutant in SMX and benzene ring in SDBC [Citation98]. It is worth noticing that the highly nitrogen-doped catalysts prepared by using algae as the raw material provide strong support for the degradation of organic pollutants in water. For example, Qi et al. [Citation105] used Enteromorpha (EGB) as the raw material and K2CO3 as the activator to form BC with three-dimensional porous surface morphology under high temperature. The graphite N in EGB promotes the adsorption and binding of PDS and SMX during the oxidation process, realizing the efficient degradation of SMX [Citation105]. Chen et al. [Citation106] synthesized an Fe/N co-doped algae-based catalyst from the same source of EGB using oxygen-limited pyrolysis and using KOH as the activator. Density functional theory (DFT) confirmed that the nitrogen-derived structure of catalyst had a good positive correlation with the removal of organic matters in water (). In short, the graphite-nitrogen structure formed by the catalyst prepared from algal biomass can effectively improve the catalytic activity of organic pollutants. In addition, oxygen-containing functional groups in algal carbon-based materials have irreplaceable advantages for catalytic activity. Ren et al. [Citation107] showed that various oxygen-containing functional groups such as carbonyl and carboxyl groups can affect the π-π interactions between the catalysts and the pollutants. A large number of oxygen-containing functional groups will reduce the zeta potential on the surface of catalyst [Citation107]. Therefore, the adsorption of negatively charged PDS and PMS can be achieved during the oxidation process, since the surface of the algal carbon-based catalyst has more oxygen-containing functional groups with positive charges [Citation107]. Qi et al. [Citation105] developed a novel algae-based carbon composite material using Enteromorpha as a precursor, which was activated by KOH to form a multi-dimensional pore structure (SSA of 1657.8 m2/g). The SSA of this composite material was 90 times higher than that of the original BC. In addition, the oxygen-containing functional groups on the surface could effectively remove nickel chelate and achieve the efficient degradation of N,N,N,’ N’-tetrakis (2-hydroxypropyl) ethylenediamine. The abundant functional groups of ABBC and endogenous N doping are conducive to the activation of PDS. The material preparation process is simple, economical, and environment-friendly, so that the catalyst prepared with algae as a raw material is a good choice [Citation42,Citation98].
Figure 5. (A) mechanism analysis of of loxacin degradation by activating peroxymonosulfate with N-doped biochar derived from kelp-derived N-doped biochar; (b) degradation of 4-nonylphenol by red algal BC; (c) mechanism of activation of PS and PMS by metal ions and metal oxides; (d) Introduction of catalytic mechanism of SMX in SDBCs/PS system. Reprinted and modified with permissions from refs [Citation37,Citation48,Citation99,Citation100].
![Figure 5. (A) mechanism analysis of of loxacin degradation by activating peroxymonosulfate with N-doped biochar derived from kelp-derived N-doped biochar; (b) degradation of 4-nonylphenol by red algal BC; (c) mechanism of activation of PS and PMS by metal ions and metal oxides; (d) Introduction of catalytic mechanism of SMX in SDBCs/PS system. Reprinted and modified with permissions from refs [Citation37,Citation48,Citation99,Citation100].](/cms/asset/1686c677-6077-4137-8f21-d544c6a49b06/kbie_a_2252157_f0005_oc.jpg)
3.2. Adsorption of organic pollutants
It has been shown that the adsorption of organic pollutants mainly depends on the surface morphology and chemical properties of BC [Citation36]. Tan et al. [Citation83] found that BC was formed under limited oxygen at 700°C, whereas high-temperature calcination rendered BC with a good capacity for adsorbing various dyes (rhodamine B, methylene blue, Congo red and methyl orange). The adsorption rate can be accelerated with the increase of pyrolysis temperature [Citation108,Citation109]. Compared with other algal BC derived from Ulva prolifera, the SSA of N-doped BC with abundant pore structure can be as high as 25.43 m2/g and its adsorption capacity of bisphenol A can reach the value of 33.33 mg/g [Citation32,Citation110]. Fourier transform infrared spectroscopy (FTIR) spectra showed that a large number of nitrogen-containing functional groups on its surface participated in the adsorption of bisphenol A. Zheng et al. [Citation87] prepared BC using Chlorella sp. Cha-01 as the raw material and its adsorption capacity for p-nitrophenol was higher than that of the original BC due to the strong polarity resulting from sufficient oxygen-containing functional groups on the surface of modified BC. The adsorption of ionized organic pollutants using BC is pH-dependent [Citation111,Citation112]. At neutral pH, BC showed good adsorption effect on malachite green and crystal violet dyes due to electrostatic attraction. However, it did not show obvious adsorption capacity for Congo red. At low pH, the π-π donor-receptor interaction is the main mechanism during the adsorption process. At high pH, it is mainly manifested that the adsorption mechanism of negative charge is assisted by H-bonding [Citation112]. In general, the mechanisms of SSA and surface functional groups for the adsorption process mainly include pore filling, ion exchange, polarity, electrostatic attraction, hydrophilic/hydrophobic, and π-π interaction ().
Figure 6. Adsorption mechanism of BC for organic (left) and inorganic pollutants (right). Reprinted and modified with permissions from refs [Citation36].
![Figure 6. Adsorption mechanism of BC for organic (left) and inorganic pollutants (right). Reprinted and modified with permissions from refs [Citation36].](/cms/asset/6e334c10-c18d-45f2-a7cc-273cd3520ec2/kbie_a_2252157_f0006_oc.jpg)
3.3. Adsorption of inorganic pollutants
The growth environments of algae are mainly sea, river, lake, and pond. The content of inorganic salts in their cells is high, due to which, they have a good tolerance for exogenous inorganic salts. Therefore, inorganic salts are often used as activators during the preparation of heavy metal adsorbents using algae as the raw material. Johansson et al. [Citation113] used 12.5% (w/v) Fe3+ solution to modify Gracilaria sp. using slow heat delivery to obtain Fe-BC, which has good adsorption effect for oxyanions of Mo, As, Se, and other heavy metals (adsorption capacity of 78.5, 62.5 and 14.9 mg/g, respectively). With the Enteromorpha used as the raw material, the adsorption of Cr(VI) by γ-Fe2O3-activated BC was much higher than that of the original BC and the maximum adsorption capacity was 91.5 mg/g. According to the FTIR results, the electrostatic interaction induced by surface -OH was the main mechanism for adsorption [Citation114]. These studies show that the preparation of iron-BC can increase the adsorption capacity of BC and significantly improve the surface characteristics of carbon materials, including pore structure and surface functional groups. Poo et al. [Citation115] used Sargassum fusiforme as the raw material to prepare BC containing a large number of oxygen-containing functional groups, which can effectively adsorb and remove Cu, Cd, and Zn from aqueous solutions. This is not only due to the negative charge on the surface of BC that can interact with positively charged heavy metals, but also due to the complexation between the oxygen functional groups (-COOH and -OH) on the surface of BC. In addition, the pH value of algal BC is high and the precipitation is also the main factor in improving the removal efficiency of heavy metals. The pH value is always the key for the adsorption efficiency. The studies have shown that the increase in pH value in solution can accelerate the adsorption and precipitation of Cu(II), which results in the formation of Cu(OH)+ and Cu(OH)2 due to hydrolysis [Citation116]. Kim et al. [Citation117] reported that BC prepared using Enteromorpha compressa as the raw material shows maximum adsorption capacity for Cu(II) at the pH of 5.5. When pH < 5.5, Cu2+ is the main form. However, when pH > 6, Cu2+ is gradually transformed into Cu(OH)+ and finally exists in the form of Cu(OH)2. Generally, at neutral pH, the surface charge of unmodified BC is negative and positively charged ions (such as NH4+, Pb2+, Cu2+, Cd2+, and Zn2+) are easily adsorbed [Citation118]. The increase in the adsorption capacity of BC is also related to the change in porous structure and surface functional groups, especially the existence of oxygen-containing functional groups. Singh et al. [Citation22] prepared ABBC with alkali modification (2 M potassium hydroxide) and its adsorption capacity for Cu(II) was 3.36 times higher than that of the original BC. The reason is that the SSA of modified BC was increased by five times and the amount of surface functional groups was also higher (-OH, -CH, C=O, C-C, and =C-H) [Citation22]. Wang et al. [Citation119] synthesized a BC composite material with an SSA of 1657.8 m2/g and abundant oxygen-containing functional groups using cyanobacteria from Taihu Lake as the raw material and KOH as the activator. The removal rate of chelated nickel from electroplating wastewater was up to 98.87%. Wang et al. [Citation120] used vermiculite to treat algae and prepared BC with uniformly distributed nano-SiO2 particles. The material showed good adsorption effect (159.42 mg/g) for phosphate through electrostatic interactions and adsorption site interactions. For the Hg(II) adsorption [Citation121], it was observed that a large number of minerals (calcium and sodium salts) were derived from high-salinity spirulina residues during the preparation of BC. As the surface functional groups such as C-O, C=O, and C=C participated in the complexation and reduction of Hg(II), Cl− induced the formation of Hg2Cl2 precipitates. In addition, BC can also be modified by iron, magnesium, zinc, and other metals to promote its adsorption of heavy metal/inorganic ion pollutants [Citation56,Citation122]. Algae-based BC can be effectively activated by inorganic salts and the mechanism of removing heavy metal/inorganic ions mainly includes electrostatic interactions, precipitation, complexation with surface functional groups, and ion exchange () [Citation22,Citation36].
4. Conclusions
Compared with other biomass resources, algae has certain advantages in terms of its safety, economy, yield and source, and is a potential biomass source for preparing BC, especially in the field of sewage treatment. Algae come from a wide range of sources, and high protein content is their common feature. Proteins can form nitrogen-rich compounds on the surface of BC after high-temperature pyrolysis. Pyridine-N, pyrrorole-N and graphitized-N are the main products of oxygen-restricted decomposition of protein at high temperature. Previous studies have confirmed that ABBC acts as a catalyst, and its graphite-N and N-O functional groups formed by endogenous N-doped can effectively activate free radicals and promote electron transfer/exchange capabilities. As the adsorbent, in addition to the three-dimensional pore structure, the strong polarity shown by the presence of sufficient oxygen-containing functional groups on the surface of algal BC is the main factor for enhanced organic adsorption. Oxygen functional groups (-COOH and -OH) are mainly involved in the complexation of inorganic pollutants. It is an effective pathway to further realize the high-value conversion of algal biomass to fully grasp the endogenous nitrogen doping characteristics of algae and prepare ABBC based on the original preparation method for various purposes.
Data available on request from the authors
The data that support the findings of this study are available from the corresponding author, upon reasonable request.
Author contributions
Xuefei Tan: Conceptualization and drafting the manuscript; Fengfa Zhang: Data curation and original draft review; Huiwen Wang: Data curation and formal analysis; Shih-Hsin Ho: Project administration and original draft review.
Acknowledgments
The authors are highly thankful to Beijing Key Laboratory of Fuels Cleaning and Advanced Catalytic Emission Reduction Technology/College of New Materials and Chemical Engineering, Beijing Institute of Petrochemical Technology, Beijing 102617, China
Disclosure statement
No potential conflict of interest was reported by the author(s).
Additional information
Funding
References
- Khoo KS, Ahmad I, Chew KW, et al. Enhanced microalgal lipid production for biofuel using different strategies including genetic modification of microalgae: a review. Prog Energ Combust. 2023;96:101071–21. doi: 10.1016/j.pecs.2023.101071
- Li S-N, Wang R, Ho S-H. Algae-mediated biosystems for metallic nanoparticle production: from synthetic mechanisms to aquatic environmental applications. J Hazard Mater. 2021;420:126625–126635. doi: 10.1016/j.jhazmat.2021.126625
- Leong WH, Saman NAM, Kiatkittipong W, et al. Photoperiod-induced mixotrophic metabolism in Chlorella vulgaris for high biomass and lipid to biodiesel productions using municipal wastewater medium. Fuel. 2022;313:123052–123058. doi: 10.1016/j.fuel.2021.123052
- Singh HM, Kothari R, Gupta R, et al.bio-fixation of flue gas from thermal power plants with algal biomass: overview and research perspectives. J Environ Manage. 2019;245:519–539. doi: 10.1016/j.jenvman.2019.01.043
- Li L, Wang X, Miao J, et al. Carbon neutrality of wastewater treatment-A systematic concept beyond the plant boundary. Environ Sci Ecotech. 2022;11:100180–100186. doi: 10.1016/j.ese.2022.100180
- Li S, Li X, Ho S-H. Microalgae as a solution of third world energy crisis for biofuels production from wastewater toward carbon neutrality: an updated review. Chemosphere. 2022;291:132863–1328675. doi: 10.1016/j.chemosphere.2021.132863
- Tan X, Wei H, Zhou Y, et al. Adsorption of sulfamethoxazole via biochar: the key role of characteristic components derived from different growth stage of microalgae. Environ Res. 2022;210:112965–112971. doi: 10.1016/j.envres.2022.112965
- Sakai N, Sakamoto Y, Kishimoto N, et al. Chlorella strains from hot springs tolerant to high temperature and high CO2. Energy Convers Manag. 1995;36(6–9):693–696. doi: 10.1016/0196-8904(95)00100-R
- Li S, Show PL, Ngo HH, et al. Algae-mediated antibiotic wastewater treatment: a critical review. Environ Sci Ecotech. 2022;9:100145–100157. doi: 10.1016/j.ese.2022.100145
- Wang Y, Ho S-H, Cheng C-L, et al. Perspectives on the feasibility of using microalgae for industrial wastewater treatment. Bioresource Technol. 2016;222:485–497. doi: 10.1016/j.biortech.2016.09.106
- Kiran B, Kumar R, Deshmukh D. Perspectives of microalgal biofuels as a renewable source of energy. Energ Convers Manage. 2014;88:1228–1244. doi: 10.1016/j.enconman.2014.06.022
- Binda G, Spanu D, Bettinetti R, et al. Comprehensive comparison of microalgae-derived biochar from different feedstocks: a prospective study for future environmental applications. Algal Res. 2020;52:102103–102112. doi: 10.1016/j.algal.2020.102103
- Venkatachalam CD, Ravichandran SR, Sengottian M. Lignocellulosic and algal biomass for bio-crude production using hydrothermal liquefaction: conversion techniques, mechanism and process conditions: a review. Environ Eng Res. 2022;27(1):123416–123429. doi: 10.4491/eer.2020.555
- Law XN, Cheah WY, Chew KW, et al. Microalgal-based biochar in wastewater remediation: its synthesis, characterization and applications. Environ Res. 2022;204:111966–111978. doi: 10.1016/j.envres.2021.111966
- Wang K, Wang Y, Zhang S, et al. Tailoring a novel hierarchical cheese-like porous biochar from algae residue to boost sulfathiazole removal. Environ Sci Ecotech. 2022;10:100168–100176. doi: 10.1016/j.ese.2022.100168
- Lee XJ, Ong HC, Gan YY, et al. State of art review on conventional and advanced pyrolysis of macroalgae and microalgae for biochar, bio-oil and bio-syngas production. Energ Convers Manage. 2020 15;210:112707–112740. doi: 10.1016/j.enconman.2020.112707
- Fenton O, hUallachain DO. Agricultural nutrient surpluses as potential input sources to grow third generation biomass (microalgae): a review. Algal Res. 2012;1:49–56. doi: 10.1016/j.algal.2012.03.003
- Madeira MS, Cardoso C, Lopes PA, et al. Microalgae as feed ingredients for livestock production and meat quality: a review. Livest Sci. 2017;205:111–121. doi: 10.1016/j.livsci.2017.09.020
- Barba FJ, Grimi N, Vorobiev E. New approaches for the use of non-conventional cell disruption technologies to extract potential food additives and nutraceuticals from microalgae. Food Eng Rev. 2015;7:45–62. doi: 10.1007/s12393-014-9095-6
- Satya ADM, Cheah WY, Yazdi SK, et al. Progress on microalgae cultivation in wastewater for bioremediation and circular bioeconomy. Environ Res. 2023;218:114948–11512. doi: 10.1016/j.envres.2022.114948
- Dai Y, Zhang N, Xing C, et al. The adsorption, regeneration and engineering applications of biochar for removal organic pollutants: a review. Chemosphere. 2019;223:12–27. doi: 10.1016/j.chemosphere.2019.01.161
- Dissanayake PD, You S, Igalavithana AD, et al. Biochar-based adsorbents for carbon dioxide capture: a critical review. Renew Sust Energ Rev. 2020;119:109582–109595. doi: 10.1016/j.rser.2019.109582
- Selvarajoo A, Wong YL, Khoo KS, et al. Biochar production via pyrolysis of citrus peel fruit waste as a potential usage as solid biofuel. Chemosphere. 2022;294:133671–133682. doi: 10.1016/j.chemosphere.2022.133671
- Fan Y, Wang B, Yuan S, et al. Adsorptive removal of chloramphenicol from wastewater by NaOH modified bamboo charcoal. Bioresource Technol. 2010;101:7661–7664. doi: 10.1016/j.biortech.2010.04.046
- Zhou Y, Liu X, Xiang Y, et al. Modification of biochar derived from sawdust and its application in removal of tetracycline and copper from aqueous solution: adsorption mechanism and modelling. Bioresource Technol. 2017;245:266–273. doi: 10.1016/j.biortech.2017.08.178
- Vaughn SF, Kenar JA, Thompson AR, et al. Comparison of biochars derived from wood pellets and pelletized wheat straw as replacements for peat in potting substrates. Ind Crop Prod. 2013;51:437–443. doi: 10.1016/j.indcrop.2013.10.010
- Chen B, Gu Z, Wu M, et al. Advancement pathway of biochar resources from macroalgae biomass: a review. Biomass Bioenerg. 2022;167:106650–106659. doi: 10.1016/j.biombioe.2022.106650
- Amin M, Chetpattananondh P. Biochar from extracted marine Chlorella sp. residue for high efficiency adsorption with ultrasonication to remove Cr(VI), Zn(II) and Ni(II). Bioresource Technol. 2019;289:121578–121587. doi: 10.1016/j.biortech.2019.121578
- Wang J, Wang S. Preparation, modification and environmental application of biochar: a review. J Clean Prod. 2019;227:1002–1022. doi: 10.1016/j.jclepro.2019.04.282
- Francois M, Lin K-S, Rachmadona N, et al. Advancement of biochar-aided with iron chloride for contaminants removal from wastewater and biogas production: a review. Sci Total Environ. 2023;874:162437–162450. doi: 10.1016/j.scitotenv.2023.162437
- Mohan D, Sarswat A, Ok YS, et al. Organic and inorganic contaminants removal from water with biochar, a renewable, low cost and sustainable adsorbent - a critical review. Bioresource Technol. 2014;160:191–202. doi: 10.1016/j.biortech.2014.01.120
- Gai X, Wang H, Liu J, et al. Effects of feedstock and pyrolysis temperature on biochar adsorption of ammonium and nitrate. PLoS One. 2014;9:1–19. doi: 10.1371/journal.pone.0113888
- Yu KL, Lau BF, Show PL, et al. Recent developments on algal biochar production and characterization. Bioresource Technol. 2017;246:2–11. doi: 10.1016/j.biortech.2017.08.009
- Gan YY, Ong HC, Show PL, et al. Torrefaction of microalgal biochar as potential coal fuel and application as bio-adsorbent. Energ Convers Manage. 2018;165:152–162. doi: 10.1016/j.enconman.2018.03.046
- Anto S, Sudhakar MP, Ahamed TS, et al. Activation strategies for biochar to use as an efficient catalyst in various applications. Fuel. 2021;285:119205–119212. doi: 10.1016/j.fuel.2020.119205
- Hu Y, Wang S, Li J, et al. Co-pyrolysis and co-hydrothermal liquefaction of seaweeds and rice husk: comparative study towards enhanced biofuel production. J Anal Appl Pyrol. 2018;129:162–170. doi: 10.1016/j.jaap.2017.11.016
- Chen Y-D, Liu F, Ren N-Q, et al. Revolutions in algal biochar for different applications: state-of-the-art techniques and future scenarios. Chinese Chem Lett. 2020;31:2591–2602. doi: 10.1016/j.cclet.2020.08.019
- Huang Y-M, Li G, Li M, et al. Kelp-derived N-doped biochar activated peroxymonosulfate for ofloxacin degradation. Science Of The Total Environment query. 2021;754:141999–142009. doi: 10.1016/j.scitotenv.2020.141999
- Chen W, Yang H, Chen Y, et al. Transformation of nitrogen and evolution of n-containing species during algae pyrolysis. Environ Sci Technol. 2017;51:6570–6579. doi: 10.1021/acs.est.7b00434
- Yang C, Li R, Zhang B, et al. Pyrolysis of microalgae: a critical review. Fuel Process Technol. 2019;186:53–72. doi: 10.1016/j.fuproc.2018.12.012
- Pavlath AE, Gregorski KS. Atmospheric pyrolysis of carbohydrates with thermogravimetric and mass-spectrometric analyses. J Anal Appl Pyrol. 1985;8:41–48. doi: 10.1016/0165-2370(85)80013-9
- Maher KD, Bressler DC. Pyrolysis of triglyceride materials for the production of renewable fuels and chemicals. Bioresource Technol. 2007;98:2351–2368. doi: 10.1016/j.biortech.2006.10.025
- Chen Y-D, Duan X, Zhang C, et al. Graphitic biochar catalysts from anaerobic digestion sludge for nonradical degradation of micropollutants and disinfection. Chemical Engineering Journal query. 2020;384:123244–123254. doi: 10.1016/j.cej.2019.123244
- Atienza-Martinez M, Rubio I, Fonts I, et al. Effect of torrefaction in an auger reactor on the Fast pyrolysis of sewage sludge. Papers of the 23rd European Biomass Conference: Setting the Course for a Biobased Economy; Vienna, Austria; 2015. p. 1247–1251.
- Zhang Z, Zhu Z, Shen B, et al. Insights into biochar and hydrochar production and applications: a review. Energy. 2019;171:581–598. doi: 10.1016/j.energy.2019.01.035
- Gao W, Chen K, Zeng J, et al. Thermal pyrolysis characteristics of macroalgae Cladophora glomerata. Bioresource Technol. 2017;243:212–217. doi: 10.1016/j.biortech.2017.06.041
- Manara P, Zabaniotou A. Towards sewage sludge based biofuels via thermochemical conversion – a review. Renew Sust Energ Rev. 2012;16(5):2566–2582. doi: 10.1016/j.rser.2012.01.074
- Chen Y-D, Li S, Ho S-H, et al. Integration of sludge digestion and microalgae cultivation for enhancing bioenergy and biorefinery. Renewable And Sustainable Energy Reviews query. 2018;96:76–90. doi: 10.1016/j.rser.2018.07.028
- Ho S-H, Chen Y-D, Li R, et al. N-doped graphitic biochars from C-phycocyanin extracted Spirulina residue for catalytic persulfate activation toward nonradical disinfection and organic oxidation. Water Research query. 2019;159:77–86. doi: 10.1016/j.watres.2019.05.008
- Jung K-W, Kim K, Jeong T-U, et al. Influence of pyrolysis temperature on characteristics and phosphate adsorption capability of biochar derived from waste-marine macroalgae (undaria pinnatifida roots). Bioresource Technol. 2016;200:1024–1028. doi: 10.1016/j.biortech.2015.10.016
- Paethanom A, Yoshikawa K. Influence of pyrolysis temperature on rice husk char characteristics and its tar adsorption capability. Energies. 2012;5:4941–4951. doi: 10.3390/en5124941
- Feng H, Jia Y, Shen D, et al. The effect of chemical vapor deposition temperature on the performance of binder-free sewage sludge-derived anodes in microbial fuel cells. Sci Total Environ. 2018;635:45–52. doi: 10.1016/j.scitotenv.2018.04.124
- Yuan Y, Yuan T, Wang D, et al. Sewage sludge biochar as an efficient catalyst for oxygen reduction reaction in an microbial fuel cell. Bioresource Technol. 2013;144:115–120. doi: 10.1016/j.biortech.2013.06.075
- Chen Y-C, Chen W-H, Lin B-J, et al. Impact of torrefaction on the composition, structure and reactivity of a microalga residue. Appl Energy. 2016;181:110–119. doi: 10.1016/j.apenergy.2016.07.130
- Mathimani T, Mallick N. A review on the hydrothermal processing of microalgal biomass to bio-oil - knowledge gaps and recent advances. J Clean Prod. 2019;217:69–84. doi: 10.1016/j.jclepro.2019.01.129
- Kim D, Park S, Park KY. Upgrading the fuel properties of sludge and low rank coal mixed fuel through hydrothermal carbonization. Energy. 2017;141:598–602. doi: 10.1016/j.energy.2017.09.113
- Arun J, Varshini P, Prithvinath PK, et al. Enrichment of bio-oil after hydrothermal liquefaction (HTL) of microalgae C. vulgaris grown in wastewater: bio-char and post HTL wastewater utilization studies. Bioresource Technol. 2018;261:182–187. doi: 10.1016/j.biortech.2018.04.029
- Alvarez-Murillo A, Sabio E, Ledesma B, et al. Generation of biofuel from hydrothermal carbonization of cellulose. Kinetics Modelling Energy. 2016;94:600–608. doi: 10.1016/j.energy.2015.11.024
- Zhang Y, Cui Y, Liu S, et al. Fast microwave-assisted pyrolysis of wastes for biofuels production - a review. Bioresource Technol. 2020;297:122480–122488. doi: 10.1016/j.biortech.2019.122480
- Wahi R, Zuhaidi N, Yusof Y, et al. Chemically treated microwave-derived biochar: an overview. Biomass And Bioenergy query. 2017;107:411–421. doi: 10.1016/j.biombioe.2017.08.007
- Fernandez Y, Arenillas A, Diez MA, et al. Pyrolysis of glycerol over activated carbons for syngas production. J Anal Appl Pyrol. 2009;84:145–150. doi: 10.1016/j.jaap.2009.01.004
- Wang N, Tahmasebi A, Yu J, et al. A comparative study of microwave-induced pyrolysis of lignocellulosic and algal biomass. Bioresource Technol. 2015;190:89–96. doi: 10.1016/j.biortech.2015.04.038
- Zhang C, Ho S-H, Chen W-H, et al. Torrefaction performance and energy usage of biomass wastes and their correlations with torrefaction severity index. Appl Energy. 2018;220:598–604. doi: 10.1016/j.apenergy.2018.03.129
- Sukiran MA, Abnisa F, Daud WMAW, et al. A review of torrefaction of oil palm solid wastes for biofuel production. Energ Convers Manage. 2017;149:101–120. doi: 10.1016/j.enconman.2017.07.011
- Rudolfsson M, Stelte W, Lestander TA. Process optimization of combined biomass torrefaction and pelletization for fuel pellet production - a parametric study. Appl Energy. 2015;140:378–384. doi: 10.1016/j.apenergy.2014.11.041
- Dai L, Yang B, Li H, et al. A synergistic combination of nutrient reclamation from manure and resultant hydrochar upgradation by acid-supported hydrothermal carbonization. Bioresource Technol. 2017;243:860–866. doi: 10.1016/j.biortech.2017.07.016
- Huang Y-F, Sung H-T, Chiueh P-T, et al. Microwave torrefaction of sewage sludge and leucaena. J Taiwan Inst Chem E. 2017;70:236–243. doi: 10.1016/j.jtice.2016.10.056
- Huang Y-B, Shen M, Wang X, et al. Water-medium C-H activation over a hydrophobic perfluoroalkane-decorated metal-organic framework platform. J Catal. 2016;333:1–7. doi: 10.1016/j.jcat.2015.10.012
- Wu K-T, Tsai C-J, Chen C-S, et al. The characteristics of torrefied microalgae. Appl Energy. 2012;100:52–57. doi: 10.1016/j.apenergy.2012.03.002
- Zhao C, Lv P, Yang L, et al. Biodiesel synthesis over biochar-based catalyst from biomass waste pomelo peel. Energ Convers Manage. 2018;160:477–485. doi: 10.1016/j.enconman.2018.01.059
- Qian K, Kumar A, Zhang H, et al. Recent advances in utilization of biochar. Renew Sust Energ Rev. 2015;42:1055–1064. doi: 10.1016/j.rser.2014.10.074
- Liu W-J, Jiang H, Yu H-Q. Development of biochar-based functional materials: toward a sustainable platform carbon material. Chem Rev. 2015;115:12251–12285. doi: 10.1021/acs.chemrev.5b00195
- Contescu CI, Adhikari SP, Gallego NC, et al. Activated carbons derived from high-temperature pyrolysis of lignocellulosic biomass. C-J Carbon Res. 2018;4:1–16. doi: 10.3390/c4030051
- Yu KL, Show PL, Ong HC, et al. Biochar production from microalgae cultivation through pyrolysis as a sustainable carbon sequestration and biorefinery approach. Clean Technol Envir. 2018;20:2047–2055. doi: 10.1007/s10098-018-1521-7
- Zhang H, Voroney RP, Price GW. Effects of temperature and activation on biochar chemical properties and their impact on ammonium, nitrate, and phosphate sorption. J Environ Qual. 2017;46:889–896. doi: 10.2134/jeq2017.02.0043
- Borchard N, Wolf A, Laabs V, et al. Physical activation of biochar and its meaning for soil fertility and nutrient leaching - a greenhouse experiment. Soil Use Manage. 2012;28:177–184. doi: 10.1111/j.1475-2743.2012.00407.x
- Lou K, Rajapaksha AU, Ok YS, et al. Pyrolysis temperature and steam activation effects on sorption of phosphate on pine sawdust biochars in aqueous solutions. Chem Speciation Bioavailability. 2016;28:42–50. doi: 10.1080/09542299.2016.1165080
- Shim T, Yoo J, Ryu C, et al. Effect of steam activation of biochar produced from a giant miscanthus on copper sorption and toxicity. Bioresource Technol. 2015;197:85–90. doi: 10.1016/j.biortech.2015.08.055
- Tan X-F, Liu S-B, Liu Y-G, et al. Biochar as potential sustainable precursors for activated carbon production: multiple applications in environmental protection and energy storage. Bioresource Technol. 2017;227:359–372. doi: 10.1016/j.biortech.2016.12.083
- Trakal L, Sigut R, Sillerova H, et al. Copper removal from aqueous solution using biochar: effect of chemical activation. Arab J Chem. 2014;7:43–52. doi: 10.1016/j.arabjc.2013.08.001
- Angin D, Altintig E, Kose TE. Influence of process parameters on the surface and chemical properties of activated carbon obtained from biochar by chemical activation. Bioresource Technol. 2013;148:542–549. doi: 10.1016/j.biortech.2013.08.164
- Zhu L, Zhao N, Tong L, et al. Structural and adsorption characteristics of potassium carbonate activated biochar. RSC Adv. 2018;8:21012–21019. doi: 10.1039/C8RA03335H
- Gamiz B, Hall K, Spokas KA, et al. Understanding activation Effects on low-temperature biochar for optimization of herbicide sorption. Agronomy-Basel. 2019;9:1–16. doi: 10.3390/agronomy9100588
- Tan X, Zhu S, Show PL, et al. Sorption of ionized dyes on high-salinity microalgal residue derived biochar: electron acceptor-donor and metal-organic bridging mechanisms. J Hazard Mater. 2020;393:122435–122443. doi: 10.1016/j.jhazmat.2020.122435
- Yu KL, Chen W-H, Sheen H-K, et al. Production of microalgal biochar and reducing sugar using wet torrefaction with microwave-assisted heating and acid hydrolysis pretreatment. Renew Energ. 2020;156:349–360. doi: 10.1016/j.renene.2020.04.064
- Leng L-J, Yuan X-Z, Huang H-J, et al. Characterization and application of bio-chars from liquefaction of microalgae, lignocellulosic biomass and sewage sludge. Fuel Processing Technology query. 2015;129:8–14. doi: 10.1016/j.fuproc.2014.08.016
- Choi Y-K, Choi T-R, Gurav R, et al. Adsorption behavior of tetracycline onto Spirulina sp. (microalgae)-derived biochars produced at different temperatures. Sci Total Environ. 2020;710:136282–136292. doi: 10.1016/j.scitotenv.2019.136282
- Zheng H, Guo W, Li S, et al. Adsorption of p-nitrophenols (PNP) on microalgal biochar: analysis of high adsorption capacity and mechanism. Bioresource Technol. 2017;244:1456–1464. doi: 10.1016/j.biortech.2017.05.025
- Lv SW, Liu JM, Ma H, et al. Simultaneous adsorption of methyl orange and methylene blue from aqueous solution using amino functionalized Zr-based MOFs. Micropor Mesopor Mat. 2019;282:179–187. doi: 10.1016/j.micromeso.2019.03.017
- Kah M, Sigmund G, Xiao F, et al. Sorption of ionizable and ionic organic compounds to biochar, activated carbon and other carbonaceous materials. Water Res. 2017;124:673–692. doi: 10.1016/j.watres.2017.07.070
- Tan X-F, Zhu S-S, Wang R-P, et al. Role of biochar surface characteristics in the adsorption of aromatic compounds: pore structure and functional groups. Chinese Chem Lett. 2021;32:2939–2946. doi: 10.1016/j.cclet.2021.04.059
- Yu KL, Lee XJ, Ong HC, et al. Adsorptive removal of cationic methylene blue and anionic Congo red dyes using wet-torrefied microalgal biochar: equilibrium, kinetic and mechanism modeling. Environ Pollut. 2021;272:115986–115997. doi: 10.1016/j.envpol.2020.115986
- Enaime G, Bacaoui A, Yaacoubi A, et al. Biochar for wastewater treatment—conversion technologies and applications. Appl Sci-Basel. 2020;10(10):1–29. doi: 10.3390/app10103492
- Nartey OD, Zhao B. Biochar preparation, Characterization, and Adsorptive capacity and its effect on bioavailability of contaminants: an overview. Advances In Materials Science And Engineering. 2014;2014:1–13. doi: 10.1155/2014/715398
- Zhu S, Huang X, Ma F, et al. Catalytic removal of aqueous contaminants on N-Doped graphitic biochars: inherent roles of adsorption and nonradical mechanisms. Environ Sci Technol. 2018;52:8649–8658. doi: 10.1021/acs.est.8b01817
- Luz FC, Cordiner S, Manni A, et al. Biochar characteristics and early applications in anaerobic digestion-a review. J Environ Chem Eng. 2018;6:2892–2909. doi: 10.1016/j.jece.2018.04.015
- Zhang J, Liu J, Liu R. Effects of pyrolysis temperature and heating time on biochar obtained from the pyrolysis of straw and lignosulfonate. Bioresource Technol. 2015;176:288–291. doi: 10.1016/j.biortech.2014.11.011
- Li H, Dong X, da Silva EB, et al. Mechanisms of metal sorption by biochars: biochar characteristics and modifications. Chemosphere. 2017;178:466–478. doi: 10.1016/j.chemosphere.2017.03.072
- Chen Y-D, Bai S, Li R, et al. Magnetic biochar catalysts from anaerobic digested sludge: production, application and environment impact. Environment International query. 2019;126:302–308. doi: 10.1016/j.envint.2019.02.032
- Fang G, Gao J, Dionysiou DD, et al. Activation of persulfate by quinones: free radical reactions and implication for the degradation of PCBs. Environ Sci Technol. 2013;47:4605–4611. doi: 10.1021/es400262n
- Hung C-M, Huang CP, Hsieh S-L, et al. Biochar derived from red algae for efficient remediation of 4-nonylphenol from marine sediments. Chemosphere. 2020;254:126916–126925. doi: 10.1016/j.chemosphere.2020.126916
- Li J, Li Y, Xiong Z, et al. The electrochemical advanced oxidation processes coupling of oxidants for organic pollutants degradation: a mini-review. Chinese Chem Lett. 2019;30:2139–2146. doi: 10.1016/j.cclet.2019.04.057
- Zhu M, Zhang L, Liu S, et al. Degradation of 4-nitrophenol by electrocatalysis and advanced oxidation processes using Co3O4@C anode coupled with simultaneous CO2 reduction via SnO2/CC cathode. Chinese Chem Lett. 2020;31:1961–1965. doi: 10.1016/j.cclet.2020.01.017
- Wang J, Wang S. Activation of persulfate (PS) and peroxymonosulfate (PMS) and application for the degradation of emerging contaminants. Chem Eng J. 2018;334:1502–1517. doi: 10.1016/j.cej.2017.11.059
- Liu G, Zhou J, Zhao W, et al. Single atom catalytic oxidation mechanism of formaldehyde on al doped graphene at room temperature. Chinese Chem Lett. 2020;31:1966–1969. doi: 10.1016/j.cclet.2019.12.023
- Qi Y, Ge B, Zhang Y, et al. Three-dimensional porous graphene-like biochar derived from Enteromorpha as a persulfate activator for sulfamethoxazole degradation: role of graphitic N and radicals transformation. J Hazard Mater. 2020;399:123039–123050. doi: 10.1016/j.jhazmat.2020.123039
- Chen C, Ma T, Shang Y, et al. In-situ pyrolysis of Enteromorpha as carbocatalyst for catalytic removal of organic contaminants: considering the intrinsic N/Fe in Enteromorpha and non-radical reaction. Appl Catal B-Environ. 2019;250:382–395. doi: 10.1016/j.apcatb.2019.03.048
- Ren W, Xiong L, Nie G, et al. Insights into the electron-transfer regime of peroxydisulfate activation on carbon nanotubes: the role of oxygen functional groups. Environ Sci Technol. 2020;54:1267–1275. doi: 10.1021/acs.est.9b06208
- Praveen S, Jegan J, Pushpa TB, et al. Biochar for removal of dyes in contaminated water: an overview. Biochar. 2022;4:10–25. doi: 10.1007/s42773-022-00131-8
- Chen Y-D, Lin Y-C, Ho S-H, et al. Highly efficient adsorption of dyes by biochar derived from pigments-extracted macroalgae pyrolyzed at different temperature. Bioresource Technology query. 2018;259:104–110. doi: 10.1016/j.biortech.2018.02.094
- Llanos J, Williams PM, Cheng S, et al. Characterization of a ceramic ultrafiltration membrane in different operational states after its use in a heavy-metal ion removal process. Water Res. 2010;44:3522–3530. doi: 10.1016/j.watres.2010.03.036
- Xiao X, Chen B, Chen Z, et al. Insight into multiple and multilevel structures of biochars and their potential environmental applications: a critical review. Environ Sci Technol. 2018;52:5027–5047. doi: 10.1021/acs.est.7b06487
- Teixido M, Pignatello JJ, Beltran JL, et al. Speciation of the ionizable antibiotic sulfamethazine on black carbon (biochar). Environ Sci Technol. 2011;45:10020–10027. doi: 10.1021/es202487h
- Johansson CL, Paul NA, de Nys R, et al. Simultaneous biosorption of selenium, arsenic and molybdenum with modified algal-based biochars. J Environ Manage. 2016;165:117–123. doi: 10.1016/j.jenvman.2015.09.021
- Chen Y, Wang B, Xin J, et al. Adsorption behavior and mechanism of Cr(VI) by modified biochar derived from Enteromorpha prolifera. Ecotox Environ Safe. 2018;164:440–447. doi: 10.1016/j.ecoenv.2018.08.024
- Poo K-M, Son E-B, Chan J-S, et al. Biochars derived from wasted marine macro-algae (saccharina japonica and Sargassum fusiforme) and their potential for heavy metal removal in aqueous solution. J Environ Manage. 2018;206:364–372. doi: 10.1016/j.jenvman.2017.10.056
- Tong X-J, Li J-Y, Yuan J-H, et al. Adsorption of Cu(II) by biochars generated from three crop straws. Chem Eng J. 2011;172:828–834. doi: 10.1016/j.cej.2011.06.069
- Liang L, Xi F, Tan W, et al. Review of organic and inorganic pollutants removal by biochar and biochar-based composites. Biochar. 2021;3:255–281. doi: 10.1007/s42773-021-00101-6
- Ho S-H, Chen Y-D, Yang Z-K, et al. High-efficiency removal of lead from wastewater by biochar derived from anaerobic digestion sludge. Bioresource Technology query. 2017;246:142–149. doi: 10.1016/j.biortech.2017.08.025
- Wang H, Wang H, Zhao H, et al. Adsorption and Fenton-like removal of chelated nickel from Zn-Ni alloy electroplating wastewater using activated biochar composite derived from Taihu blue algae. Chem Eng J. 2020;379:122372–122382. doi: 10.1016/j.cej.2019.122372
- Wang Y-Y, Lu H-H, Liu Y-X, et al. Removal of phosphate from aqueous solution by SiO2-biochar nanocomposites prepared by pyrolysis of vermiculite treated algal biomass. RSC Adv. 2016;6:83534–83546. doi: 10.1039/C6RA15532D
- Ge Y, Zhu S, Chang J-S, et al. Immobilization of Hg(II) on high-salinity Spirulina residue-induced biochar from aqueous solutions: sorption and transformation mechanisms by the dual-mode isotherms. Environ Pollut. 2020;265:115087–115095. doi: 10.1016/j.envpol.2020.115087