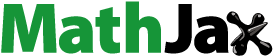
ABSTRACT
Residual antibiotics have become emerging contaminants of concern for their adverse impact on the ecosystem. Additionally, their accumulation in the environment is increasing antibiotic resistance among pathogens. This study assessed the impact of intensification of biochar, nutrients, aeration, and bacteria (BNAB) on the remediation potential of floating treatment wetlands (FTWs) to treat amoxicillin (AMX)-contaminated water. The FTWs were developed with saplings of Vetiveria zizanioides and intensified with biochar (1.5%), nutrients (25 mgL−1 N, 25 mgL−1 P, 20 mg L1 K), aeration (7 mg L−1), and AMX-degrading bacteria. The results showed that all the amendments enhanced the AMX degradation, while the maximum reduction in COD (89%), BOD (88%), TOC (87%), and AMX (97%) was shown by the combined application of all the amendments. The combined application also enhanced plant growth and persistence of the inoculated bacteria in the water, roots, and shoots. This approach can be employed for the low-cost, environment-friendly treatment, and recycling of antibiotic-contaminated wastewater, where BNAB intensification can further improve the bioremediation efficiency of FTWs in the case of heavily polluted waters.
Highlights
Vetiver grass floating treatment wetlands (FTWs) removed 83% amoxicillin.
Intensification of floating treatment wetlands enhanced amoxicillin removal to 97%.
Intensified-FTW removed COD, BOD, and TOC by 89%, 88%, and 87%, respectively.
Potential of Intensified-FTW for bioremediation of highly polluted water is shown.
GRAPHICAL ABSTRACT
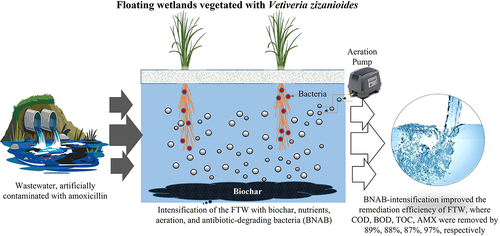
1. Introduction
Water pollution has become a serious concern in the modern world due to the growing industries, unprecedented increase in population, and over-urbanization. About 90% of the wastewater (WW) is generated from anthropogenic activities that has led to the contamination of water resources consequently affecting the aquatic fauna and flora and socio-economic conditions particularly in developing countries [Citation1]. This WW is polluted with various kinds of contaminants such as hydrocarbons, textile dyes, heavy metals, and antibiotics [Citation2,Citation3]. Antibiotics are the most significant pharmaceuticals related to human as well as animal health. Moreover, their use has been rising continuously for the last past decades, and a 36% increase was observed between 2000–2015 and 2015–2016, respectively [Citation3]. Based on the data collected from 76 countries, the total antibiotic consumption rate grew by 39% to 42.3 billion defined daily dose. The highest increase in global antibiotic consumption (75%) was reported in Brazil, China, Russia, India, and South Africa. Some European countries and USA are the principal users of antibiotics for animal food. In European countries, the consumption of penicillin ranges from 36% in Germany to 71% in Slovenia. In USA, the most prescribed (38%) antibiotic is penicillin followed by β-lactams (16%) and tetracyclines (15%) [Citation4,Citation5]. In addition, the global antibiotic expenditure is expected to increase by 15% in 2030 [Citation6]. Furthermore, penicillin is the third most prescribed (28%) group of antibiotics in India. Asia is major producer of aquaculture and contribute 90% in global food demand where China contributes 80–90% of shrimp and fish demands. Similarly, Chile is the major producer of Salmons which are raised with assortment of many antibiotics [Citation4]. Amoxicillin (AMX) is one of the most consumed antibiotics belonging to penicillin group and is highly effective against both Gram-positive and Gram-negative bacteria. It is also used to treat urinary and dermal bacterial infections as well as for the ailments related to pulmonary and gastrointestinal tracts [Citation7].
The primary sources (80%) of antibiotics include excretion via urine and secretion via milk of partially metabolized antibiotics by animals and humans [Citation8], while other sources include waste and unused antibiotics from hospitals, residential facilities, and pharmaceutical manufacturing process, where all of these are ended up in WW [Citation9]. The main reason regarding the prevalence of AMX in WW is due to the recommendation of high medicinal dosage (750–2250 mgd−1) and its low metabolic degradation [Citation10]. Moreover, the metabolic degradation rate of AMX is low that is why it has greater chances to be secreted in WW. In India, AMX, together with its degradation products, is found in effluent of sewage treatment plants at the rate of 62 ngL−1. AMX and its consumed products are also reported in effluent of WW treatment plant (WWTP) of many countries. For example, in Italy, AMX and AMX-diketopiperazine-2´,5´ are reported in three out of eight WWTP in Palermo (120 ngL−1), Varese-Olona (25 ngL−1) and Latina (15 ngL−1). In UK AMX is reported in 3 WWTP; 198–245 ngL−1 in Trefforest Estate, 56–60 ngL−1 in Cardiff. In Australia and Brisbane, AMX is detected in Sewage (280 ngL−1) and effluent (30 ngL−1) of WWTP, respectively [Citation8]. The various concentrations of AMX was also reported in the water, such as WW from German hospitals (28–87 mgL−1), and in surface waters of North Rhine West Phalia region (up to 48 ngL−1) [Citation7,Citation11] and it is a known emerging contaminant in the environment [Citation12]. Different concentrations of AMX ranging from ugL −1 to ngL−1 are also detected in river water samples in India and China. Yamuna river of India is contaminated with AMX [Citation13]. Li et al. (2018) analyzed water samples from the Mississippi River Basin and reported the detection of multiple antibiotics, including sulfamethoxazole, trimethoprim, and erythromycin. Yin et al (2021) investigated 70 antibiotics residues in the seven major river systems of China [Citation14]. Similarly, Zhou et al (2022) detected 14 antibiotics in surface water of Chaohu Lake and its tributaries. The amount of antibiotics ranged from 0 to 892 ngL−1 most of which detected in Shiwulihe and Nanfeihe rivers [Citation15]. The risk assessment of antibiotics revealed that ofloxacin, ciprofloxacin and sulfamethoxazole, erythromycin, and norfloxacin as well as sulfamethazine posed a high ecological risk to algae and plants [Citation15].
Due to a significant increase of antibiotics in the environment, many nations are implementing regulatory actions to mitigate their negative environmental impacts. These methods include physical treatment such as membrane technology and adsorption. Membrane technology is a physical technique which involves removal of diverse-sized particles using specialized membranes based on surface charge, hydrophobicity, and pore size of membranes. Novel technologies like nanofiltration and reverse osmosis have been applied to remove pharmaceuticals with 99% efficiency [Citation16]. Adsorption is also an effective method to extract the chemical compounds from liquid phase and transferring them to solid phase and is efficient in terms of recycling, recovery, and multiple uses of adsorbent [Citation17]. In chemical treatment, conventional and advanced oxidation processes (AOPs) are used as polishing techniques to increase removal efficiency. Conventional oxidation includes photolysis, ozonation, chlorination, and Fenton processes in which either UV radiation ior bromine or chlorine is used for contaminant removal but are less efficient [Citation18]. AOPs is beneficial method and have shown 80–90% removal efficiency of ECs but unable to remove higher chemicals and is often used in combination with biological process [Citation19,Citation20]. However, in biological treatment, microorganisms such as algae, fungi, and bacteria are used to degrade complex organic compounds into inorganic molecules [Citation21]. Biological treatment can be conventional and non- conventional. Moving bed biofilm reactors, nitrification, activated sludge, and trickling filter are conventional methods. Non-conventional biological treatment is advanced and integrated technology in which sorption, oxidation, and biodegradation takes place in a single system and includes artificial wetlands such as FTWs and CWs. These wetlands are aquatic plant-based systems and are more efficient (99%) than any other WWT technology due to their low cost, environment friendliness, and simple operation, and are widely used for degradation of all types of organic and inorganic contaminants (pharmaceuticals, heavy metals etc.) in WW [Citation22–24]. However, floating treatment wetlands (FTWs) are becoming popular due to low capital and operation cost. They also cater as the auxiliary remunerations in development of wildlife habitats and eye-catching green territories [Citation25,Citation26]. The FTW is a simple but promising technology of WW treatment in which plants are grown in flowing water (soil-free) using solid and floatable mat. Various kinds of plants, such as reeds, sedges, and rushes, are used to develop FTWs for the treatment of various types of pollutants in WW [Citation1,Citation3,Citation7,Citation10,Citation27,Citation28]. The inoculation of pollutant degrading bacteria (endophytes/rhizospheric bacteria) in FTWs further enhances their effectiveness in degrading pollutants in WW [Citation28–30]. The plants provide habitat for bacteria to produce biofilms. On the other side, created biofilm entraps the nutrients and suspended solids from WW and subsequently increases the growth of plants [Citation29,Citation30].
The addition of nutrients (NPK), biochar (BC), and aeration also assist the FTWs by improving plant growth and their associated microorganisms [Citation31,Citation32]. Nutrients are required for the synthesis of amino acids, proteins, carbohydrates, and involve in the cell division of plants and bacteria [Citation32]. BC affects heavy metal transportation, mobilization, and precipitation as well as play a key role in the release of nutrients, and microbial diversity, thus supporting plant growth [Citation33,Citation34]. Aeration not only provides the dissolved oxygen for the plants and bacterial growth but also significantly removes the phosphorus and ammonium from WW [Citation32,Citation34].
Although FTWs are extensively used for the remediation of contaminated water with different types of pollutants [Citation28–30], there is no study reported on the effect of intensification of FTWs to remove the AMX contamination from water. The previous studies reported that BC has been extensively used as an adsorbent for the removal of nutrients, organic pollutants, and toxic metal from WW [Citation35,Citation36]. In addition, some studies reported that combined use of BC with bacteria or aeration has also removed the emerging contaminants from WW [Citation31,Citation33], Similarly, combined application of aeration and nutrients exhibited positive effect on hydrocarbon degradation in constructed wetlands [Citation31,Citation37].
Therefore, the aim of current study was to assess the effect of intensification of biochar, nutrients, aeration, and bacteria (BNAB) on the bioremediation efficiency FTWs vegetated with Vetiveria zizanioides to clean the amoxicillin-contaminated water. It was found that intensification had a positive impact on the plant growth in terms of biomass and root and shoot length. This study will extend the applications of FTWs to develop integrated biosystems for the efficient bioremediation of highly polluted waters.
2. Materials and methods
2.1. Chemicals and materials
Capsules of AMX (500 mg) were purchased from local pharmacy of Faisalabad (Medilane Pharmacy). The locally made polystyrene sheets (Diamond Foam Company) were used to develop the floating mat. The analytical chemicals were purchased from Merk (Germany) and Sigma-Aldrich (USA). While wheat straw biochar was obtained from University of Agriculture, Faisalabad. The ethical approval to conduct the study was approved by the Ethical Review Committee of National Institute for Biotechnology and Genetic Engineering vide letter number: NIBGE/ERC-Biosafe/2020–003.
2.2. Plant tolerance to amoxicillin (AMX)
V. zizanioides was selected for the development of FTWs due to its unique physiological and morphological characteristics such as deep root system, tolerance to extreme environmental challenges such as temperature and prolonged submergence as well as increased resistance to pesticides, insecticides, and herbicides [Citation38]. An experiment was conducted to evaluate the plant tolerance against five different concentrations of AMX. Plantlets of equal length were vegetated in plastic pots of 800 cm3 volume. For vegetation, polystyrene sheet was cut in a round shape with the diameter of about 8 cm and a hole was made in the center to hold the plant in place with the help of coconut shavings. Initially, plants were allowed to grow in tap water for the development of roots and acclimatization to aquatic environment for two weeks. Then, the plants were subjected to different concentrations (200, 400, 600, 800, and 1000 mgL−1) of AMX.
2.3. AMX degradative potential of the bacterial strains
Ten previously isolated strains were tested to determine their AMX degradative potential. These bacterial strains were Acinetobacter sp. BRSI56, Acinetobacter sp. BRRH61, Bacillus cereus LORH97, Bacillus flexus LERI71, Acinetobacter sp. ACRH77, Enterobacter cloacae LCRI86, Burkholderia phytofirmans PsJN, Pantoea gaviniae NT3, Acinetobacter junii NT5, and Bacillus subtilis NT23. These bacterial strains were already characterized for their plant growth promoting and hydrocarbons degrading abilities [Citation1,Citation39,Citation40]. The degradation potential of strains was measured by growing them on M9 media containing 800 ppm AMX at 37°C in a shaking incubator (shake flask study) [Citation41]. Among these, three bacterial strains (BRSI56, PsJN NT 23) were selected on the basis of their maximum growth and AMX degradation potential (data not shown). The chosen strains were cultivated in LB media for 24 h, and their cells were harvested by centrifugation at 4000 rpm at 4°C [Citation41]. 100 mL of inoculum (containing equal cells of each strain, 109 CFUmL−1) containing the consortium of bacterial strains was added into the selected treatments [Citation1].
2.4. Nutrients
Stock solution of nutrients (nitrogen, phosphorus, and potassium) was prepared by adding recommended amounts of two fertilizers in water, diammonium phosphate (53.2 mgL−1), and muriate of potash (20 mgL−1). The prepared solution (2 mL) was initially added in all floating wetlands mesocosms to fulfill the nutrient demands of plant. The second dose (5 mL) was added in the selected treatments of the experiment [Citation31].
2.5. Biochar
Biochar (BC) was prepared using wheat straw in a furnace at 550°C for 1 h. The BC (0.425 mm porosity) was added (1.5% w/v of the water) to the selected treatments by packing it in the cotton cloth to avoid the suspension in water.
2.6. Aeration
An aeration pump was used to supply the air to selected mesocosms. The aeration rate was maintained by measuring the dissolved oxygen (DO) concentration (7 ± 2 mgL−1) in treatments. The DO was maintained continuously till the end of experiment [Citation31].
2.7. Development of floating treatment wetlands
The experiment was conducted for 8 weeks in ambient conditions to assess the effect of intensification of FTWs with BNAB on the AMX degradation potential of FTWs vegetated with V. zizanioides at NIBGE (31.39689º N, 73.02719º E), Faisalabad. The batch experiment was conducted in 20-L plastic tanks (36 cm height and 28 cm diameter) for the remediation of AMX-contaminated water (). A total of 27 mesocosms were established under ambient conditions of temperature and light. The floating mats were prepared by cutting 3—inchethick Diamond Jumbolon sheets [Citation42] in round shape with a hole in the center of the sheet to hold 10 saplings of the plant with the help of soil and coconut shavings. The floating mats were wrapped with aluminum foil to prevent damage from harmful ultraviolet radiations. Initially, saplings were allowed to grow and establish roots in tap water for 30 days. Then, the tap water was replaced with AMX-contaminated water (800 mgL−1). There were seven treatments in triplicates along with two controls ().
Figure 1. Development of floating treatment wetlands and preparation of experimental setup for remediation of AMX-contaminated water. Polystyrene sheet wrapped with aluminum foil (a), fixing of plant in plastic pot with the help of soil and coconut shaving (b, c), prepared plant pots for fixing in polystyrene sheet (d), addition of cloth wrapped biochar in FTWs tanks (e, f), placing of plant in floating mat in water containing tank (g), aeration pipes setup for treatments (h), experimental setup with all treatments (i).

Table 1. Floating treatment wetlands (FTWs) vegetated with Vetiveria zizanioides intensified with bacteria, nutrients, aeration, and biochar (BNAB) for the remediation of amoxicillin (AMX) contaminated water.
2.8. Collection of water samples and analyses
The water samples were collected every five days from each treatment and analyzed for COD, BOD, TOC, pH, EC, TDS, and dissolved oxygen (DO) as described earlier [Citation16]. The COD was determined by colorimetric method using UV-Vis Spectrophotometer, while a 5-day test was used to measure the BOD. The pH was measured using a benchtop digital pH meter (AccumetModel-25, Denver Instrument, USA). EC and TDS were measured using benchtop EC meter (CON 500 COLEPARMER, Singapore). Whereas DO in water was determined using titration method. In addition, phytotoxicity assessment was done using Triticum aestivum seeds as reported previously [Citation1].
2.9. Determination of persistence of inoculated bacteria
The plate count method was employed to estimate the number of inoculated bacteria in water samples as reported earlier [Citation41,Citation42]. Water samples were plated on LB plates. At the end of experiment, the plant roots and shoots were harvested, and their surface was sterilized by 70% ethanol followed by washing with 1% (v/v) sodium hypochlorite. Later, they were rinsed with sterilized distilled water thrice. The sterilized shoots and roots were mixed in a normal saline (0.9% NaCl) and their suspension were plated on LB plates. The petri plates were placed in an incubator at 37°C, and CFU was counted after 48 h [Citation3,Citation41,Citation43.].
2.10. Determination of AMX in water
The residual amount of AMX in the water was determined by a reported method [Citation44]. Briefly, in one mL of sample, 2 mL of potassium ferricyanide (3 gL−1), 1 mL of 0.1 N NaOH (4 g L−1), and 2 mL of 4-amino antipyrine (AAP) (3 gL−1) were added. The total volume was made to 10 mL by adding distilled water. For the preparation of blank, distilled water was added in place of sample. The prepared samples and standard solutions (100–800 mg L−1) were analyzed by UV-Vis Spectrophotometer at 509 nm. The residual amount was measured by multiplying the optical density (OD) of sample with standard factor Eq (1). The standard factor (SF) was calculated using Eq (2).
2.11. Assessment of plant growth
At the end of the experiment, the plants were harvested and the roots were gently washed with tap water to get rid of any debris and were dried using filter paper in open air. Growth of plants was determined by measuring the root and shoot length with the help of measuring tape. The fresh weight of shoots and roots was determined by a physical balance. Later, the plants were oven-dried at 60°C for 72 h and then weighed to get the dry weight [Citation45].
2.12. Phytotoxic analyses
The toxicity of treated water was determined in a lab-scale study. The seeds of wheat (Triticum aestivum) were soaked in treated and untreated water. Each treatment was conducted in triplicates, and the seeds were put on filter paper and seed germination as well as the root and shoot length were noted at 7th day of soaking [Citation1,Citation46].
2.13. Statistical analyses
Data of all physicochemical parameters (pH, EC, TDS, DO, COD, BOD, etc.), plant growth parameters and AMX degradation were calculated using Microsoft Excel 2016. The data then analyzed by one-way analysis of variance (ANOVA) using Statistix 8.1 (Tallahassee, USA). The difference between means was determined through least significant difference (LSD) followed by ANOVA.
3. Results and discussion
Previously, intensification of FTWs with biochar, nutrients, aeration, and pollutant degrading bacteria has shown to increase the bioremediation of emerging contaminants from WW [Citation31,Citation33,Citation36,Citation47]. The current research work aimed to evaluate the bioremediation efficiency of intensified-FTWs to treat the AMX-contaminated water, where V. zizanioides-vegetated FTW was intensified with biochar, nutrients, aeration, and amoxicillin-degrading bacteria. Various water quality test parameters such as COD, BOD, TOC pH, TDS, EC, and DO were measured for the evaluation of effect intensification of BNAB on FTWs. The plant growth was assessed by measuring plant biomass and root and shoot length. The residual amount of AMX in contaminated water was also assessed. The experiment was performed for 20 days during the month of August 2022. During the whole period of experiment, there was minimum rainfall and average temperature remained around 41°C. The average precipitation recorded at experimental site was 15 mm. The pots were covered with plastic sheet during the rain.
3.1. Plants tolerance to different concentrations of AMX
The plant subjected to different concentrations of AMX showed different responses in terms of their shoot and root length, as well as fresh and dry weight. The increase in shoot and root length in treatments and control (plant vegetated in tap water) was in the following order: tap water (C0; FTWs vegetated with V. zizanioides in tap water, containing no AMX) > T4 (FTWs vegetated with V. zizanioides in tap water containing 800 mgL−1 AMX) > T1 (FTWs vegetated with V. zizanioides in tap water containing 200 mgL−1 AMX) > T2 (FTWs vegetated with V. zizanioides in tap water containing 400 mgL−1 AMX) > T3 (FTWs vegetated with V. zizanioides in tap water containing AMX 600 mgL−1 AMX) > T5 (FTWs vegetated with V. zizanioides in tap water containing 1000 mgL−1 of AMX). The increase in root and shoot length was 257%, 239%, 203%, 184%, 139%, and 121% for C0, T4, T3, T2, T1, and T5, respectively, after 35 days of vegetation (data not shown). Similar increasing trend was determined in fresh and dry biomass of the plant. The minimum biomass was observed in T5 having 1000 mg L−1 AMX, while maximum biomass was found in the treatment having 800 mg L−1 AMX. Plants survived till the end of experiment; however, in T5, some plant shoots became dry and exhibited severe chlorosis. These results were similar to the result of previous research in which biomass of Canna indica increased to 50% in the presence of gemifloxacin in water [Citation48]. The toxic effect of AMX on plant at higher concentration (600 mgL−1) was reported by an earlier study [Citation7]. Similarly, fresh and dry biomass and shoot length were increased at higher concentration such as 400 mgL−1 as compared to lower AMX concentrations 200 mgL−1 and higher concentrations as well at 600 mgL−1 [Citation7]. The negative effect on plants in T5 might be due to decrease in lipid, starch, protein and photopigments content and increase in carotenoids and total chlorophylls in the leaves of plants at higher concentration of AMX [Citation49]. V. zizanioides improves the effectiveness of FTWs by absorbing and degrading pollutants in its roots and transferring them to shoots [Citation50]. One study reported that vetiver completely eliminated the tetracycline from aqueous media in 40 days only [Citation51].
3.2. Effect of various treatments on physicochemical characteristics of water
The results of the present study showed that the values of all the physiological parameters were reduced in all treatments ( and ). The parameters like pH (25%), EC (79%), TDS (55%), COD (88%) BOD (88%), and TOC (87%) were substantially reduced in the treatment (T7) having BNAB. However, a small increase in EC and TDS was observed in T7 and T4 during initial stages of experiment. While minimum reduction in the values of all these parameters were seen in T5. The DO slightly decreased in T6 and T3 treatments but continued to rise in T7. The lowest DO concentration was determined in T5 treatment. The more reduction in the values of COD, BOD, TOC, EC, and TDS in T7 than other treatments may be due to the application of BNAB in FTWs. Many earlier studies also reported that the addition of BNAB in FTWs enhanced the removal of pollution parameters, such as COD, BOD, and TDS. For example, in an earlier study, the aeration aided in FTWs vegetated with vetiver caused 58% COD, 55% BOD, 39.3% EC, and 56% TDS, reduction [Citation52]. Similarly, in another studies, addition of BC and nutrients enhanced the efficiency of the wetlands to remove organic and inorganic pollutants from the water [Citation31,Citation32,Citation34,Citation53]. V. zizanioides showed the removal efficiencies of COD, phosphorus, and TDS by 97%, 66%, and 26%, respectively [Citation50]. A study revealed the performance of vetiver in remediation of secondary effluent where removal efficiencies of antibiotics, COD, and TOC were reported higher than 90%, 40%,, and 50%, respectively [Citation54]. The configuration of constructed wetlands with BC, substrate, and plant also increased the removal efficiency of COD to 88.8% [Citation55].
Figure 2. Reduction in chemical oxygen demand (COD). C1, AMX contaminated water; T1, AMX contaminated water with FTWs; T2, AMX contaminated water having FTWs inoculated with bacteria; T3, AMX contaminated water having FTWs, bacteria, and nutrients; T4 AMX contaminated water having FTWs, bacteria, and biochar; T5, AMX contaminated water having only bacteria; T6, AMX contaminated water having FTWs, bacteria and aeration; T7, AMX contaminated water having FTWs, bacteria, aeration, nutrients, and biochar. Data are mean of triplicates (±standard deviation is shown with mean). Values with different letters are significantly different from one another (LSD; p <0.05).

Figure 3. Reduction in biochemical oxygen demand (BOD). C1, AMX contaminated water; T1, AMX contaminated water with FTWs; T2, AMX contaminated water having FTWs inoculated with bacteria; T3, AMX contaminated water having FTWs, bacteria, and nutrients; T4 AMX contaminated water having FTWs, bacteria and biochar; T5, AMX contaminated water having only bacteria; T6, AMX contaminated water having FTWs, bacteria, and aeration; T7, AMX contaminated water having FTWs, bacteria, aeration, nutrients, and biochar. Data are mean of triplicates (±standard deviation is shown with mean). Values with different letters are significantly different from one another (LSD; p <0.05).

Figure 4. Reduction in total organic carbon (TOC). C1, AMX contaminated water; T1, AMX contaminated water with FTWs; T2, AMX contaminated water having FTWs inoculated with bacteria; T3, AMX contaminated water having FTWs, bacteria, and nutrients; T4 AMX contaminated water having FTWs, bacteria, and biochar; T5, AMX contaminated water having only bacteria; T6, AMX contaminated water having FTWs, bacteria, and aeration; T7, AMX contaminated water having FTWs, bacteria, aeration, nutrients and biochar. Data are mean of triplicates (±standard deviation is shown with mean). Values with different letters are significantly different from one another (LSD; p <0.05).

Table 2. Effect of aeration, nutrients, biochar, and bacteria on pH, electrical conductivity (EC), total dissolved solids (TDS), and dissolved oxygen (DO) of amoxicillin-contaminated water treated by floating treatment wetlands planted with Vetiveria zizanioides.
The reduction in pH occurred due to the plant roots’ active mechanism that release some low molecular weight organic acids [Citation43,Citation56,Citation57]. However, EC and TDS reduction in FTWs might be attributed to the binding of inorganic minerals to plant roots. This reduction was further enhanced due to the excellent absorption performances of biochar. BC has been reported with negative surface charges, which enhance the absorption of positive charged minerals [Citation35,Citation58]. In this study, the increase in EC and TDS in T7 and T4 was observed at initial stages of experiment. This increase in EC and TDS may be associated with addition of nutrients in wetland [Citation58]. More reduction in COD, BOD and TOC was observed in T7 and T3 than other treatments. Similarly, BC intensified CWs increased the removal efficiency of COD (109%), ammonia (145%), and TN (178%) as compared to non-BC CWs [Citation59]. This reduction was due to the increase in growth of AMX degrading bacteria, and their population was enhanced by the addition of BNA [Citation58]. The increase in DO in the treatments without aeration might be due to the presence of oxygen-containing functional groups in BC [Citation34]. Moreover, plants also provide oxygen in the water through their extensive root system [Citation41,Citation42].
3.3. Effect of BNAB on AMX degradation
The residual amount of AMX was determined at regular intervals of experiment () and it was revealed that its concentration continued to decrease till the end of experiment and maximum reduction was observed at 20th day of experiment. Among different treatments, maximum (97%) reduction was exhibited by T7, followed by T3, T4, T6, T2, and T1. The minimum reduction (from 799.8 mgL−1 to 252 mgL−1) was observed in T5. In an earlier study, 92% removal of AMX was observed using FTWs having Lemna minor [Citation27]. Other earlier studies also reported that the addition of BC in FTWs reduced the concentration of sulfonamide, sulfapyridine, and sulfamethoxazole antibiotics in water [Citation36,Citation60]. It was found that BC increases the efficiency of constructed wetlands in removal of AMX (75.51%) from WW. The mechanism behind the enhanced antibiotic removal by the addition of biochar might be due to electron donor-acceptor interactions and with the attachment of the antibiotics on the surface area of BC [Citation36]. The remarkable decrease in AMX concentration in water in this study is supported by a previous study in which FTWs had been reported to decrease 84.5% of AMX from water [Citation10].
Figure 5. Reduction in concentration of AMX. C1, AMX contaminated water; T1, AMX contaminated water with FTWs; T2, AMX contaminated water having FTWs inoculated with bacteria; T3, AMX contaminated water having FTWs, bacteria, and nutrients; T4 AMX contaminated water having FTWs, bacteria, and biochar; T5, AMX contaminated water having only bacteria; T6, AMX contaminated water having FTWs, bacteria and aeration; T7, AMX contaminated water having FTWs, bacteria, aeration, nutrients, and biochar. Data are mean of triplicates (±Standard deviation is shown with mean). Values with different letters are significantly different from one another (LSD; p <0.05).

3.4. Effect of the inoculated bacteria in remediation of AMX-contaminated water
In this study, the inoculated bacteria were shown to present in the water, roots, and shoots persistently. The provision of BC, nutrients, and aeration (BNA) in the FTWs substantially increased the bacterial count in the mesocosms. The persistence and number of the bacteria were maximum in the water and roots and shoots of the plants of T7 (). In the water, the bacterial count was continuously increased from day 0 to day 10 of experiment in all treatment. The bacteria reached to the exponential growth at day 10. After that, the bacterial count remained approximately same up to day 15 and started to decrease onwards. Whereas in the case of root and shoot interior, bacterial population was maximum in T7. The lowest number of bacteria was exhibited in the roots and shoots of the plants of T2. A 98% removal of ciprofloxacin was exhibited when FTWs were supplied with consortium of three bacterial strains (Acinetobacter lwoffii ACRH76, Bacillus pumulis C2A1, and Acinetobacter sp.) and vegetated with Canna indica [Citation22].
Table 3. Effect of various treatments on the persistence of the inoculated bacteria in the water.
Table 4. Effect of various treatments on persistence of the inoculated bacteria in the root and shoot of the plant.
The continuous rise in bacterial population can be attributed to the BNA that were provided to plant and bacteria [Citation53]. Provision of aeration in FTWs also provide dissolved oxygen for exponential bacterial growth. The bacteria penetrate in the plant parts as well where they play a major role in the degradation of contaminants taken up by plant from WW [Citation34,Citation61]. The results of this study were similar to the results of a an earlier study where addition of BC-enhanced (161%) bacterial population [Citation62]. The addition of BC assists in increasing the bacterial colonization by providing large surface area for microbial attachment on BC [Citation58,Citation60]. In this study, the bacteria population was decreased during last days of experiment probably due to the decrease in nutrients as well as the contaminant [Citation28].
3.5. Evaluation of plant growth under different treatments
In this study, the growth parameters such as root and shoot length along with fresh and dry weight of the plant were significantly enhanced by the intensification of FTWs with BNAB (). The individual treatments of all these amendments although increased plant growth, but lesser as compared to their combined treatments. Maximum increase in the root and shoot length as well as in fresh and dry biomass was observed in treatment T7, while minimum growth was found in treatment T5 having only nutrients and bacteria. The increase in growth of plants in different treatments was in the order: T7> T3> T4> T6> T2> T1> T5. Similarly, increase in vetiver growth (root and shoot length, tiller number) was observed by Moula et al. (2020) when they supplied NPK nutrients to the plant. In another study, increase in plant length and fresh biomass due to the application of nutrients, surfactant and aeration has also been reported in Phragmites australis [Citation31]. Bhatt and Gauba also reported that the growth of Ocimum basilicum and the degradation of AMX were significantly improved by the application of nutrients [Citation7]. In this study, the presence of BC also positively affected the plant growth by providing large surface area for microbial attachment and oxygen-containing functional groups of BC [Citation35,Citation47]. The bacteria release plant growth promoting hormones, and biochar has the ability to provide source of organic carbon which in turn increase the plant growth [Citation58]. The growing plant uptake the nutrients from WW and provide these nutrients to the bacteria living in rhizosphere and endosphere in addition to supplying dissolved oxygen. The increasing number of bacteria assist in degrading pollutant and ultimately increasing the plant growth [Citation31,Citation34].
Figure 6. Growth of vetiver grass. Root and shoot length (a); fresh and dry weight of vetiver (b) C2, tap water having FTWs; T1, AMX contaminated water with FTWs; T2, AMX contaminated water having FTWs inoculated with bacteria; T3, AMX contaminated water having FTWs, bacteria and nutrients; T4 AMX contaminated water having FTWs, bacteria, and biochar; T5, AMX contaminated water having only bacteria; T6, AMX contaminated water having FTWs, bacteria, and aeration; T7, AMX contaminated water having FTWs, bacteria, aeration, nutrients, and biochar. Data are mean of triplicates (±Standard deviation is shown with mean). Values with different letters are significantly different from one another (LSD; p <0.05).

3.6. Phytotoxicity assessment of treated water
Phytotoxicity assessment disclosed that untreated AMX-contaminated water (C1) caused 60% inhibition in seed germination as well as restricted the growth of seedlings in terms of radicle and plumule length (). The maximum seed germination (90.34%) and radicle and plumule length (6.63 and 9.61, respectively) were exhibited by the seeds irrigated with the water treated by FTWs (T7) having the application of BNAB, followed by T3 where nutrients and bacteria were supplied to FTWs.
Table 5. Phytotoxicity assessment of treated and untreated amoxicillin-contaminated water.
4. Conclusions
Although floating wetlands have shown promising potential toward bioremediation of the contaminated waters, however, their efficiency is compromised when it comes to develop bioremediation systems for the highly polluted waters containing toxic emerging contaminants. Here, an integrated approach was employed through intensification of Vetiver grass-FTWs with biochar, nutrients, aeration, and selected bacteria (BNAB), which significantly improved amoxicillin degradation efficiency (97%) of the FTW. It is recommended that combined application of BNAB in FTWs vegetated with vetiver grass could be a promising and cost-effective approach for the bioremediation of heavily polluted waters to remove the emerging contaminants of concerns.
Author contributions statement
S. Bano: Methodology, Formal analysis, Investigation, Writing – original draft. SA Tahira: Supervision, Methodology, Investigation, Data curation. SNH Naqvi: Resources, Writing – review and editing. R Tehseen: Resources, Writing – review and editing. G Shabir: Resources, Formal analysis. S Iqbal: Resources, Writing – review and editing. M Afzal: Conceptualization, Project administration, Resources, Writing – review and editing. M Amin: Methodology, Data curation. MA Mehmood: Conceptualization, Writing – review and editing. R Boopathy: Resources, Writing – review and editing.
Ethical approvals
This study did not involve any Bioengineering and/or animal. However, for using antibiotics and wastewater, the ethical approval was obtained from Ethical Review Committee of National Institute for Biotechnology and Genetic Engineering, Faisalabad, Pakistan [Notification number: NIBGE/ERC-Biosafe/2020–003.
Disclosure statement
It is hereby disclosed that Raj Boopathy serves as Deputy Editor on the Bioengineered editorial board who is also a co-author in this manuscript. However, authors declare that they have no financial or other competing interests.
Data availability statement
Data will be made available if needed.
Additional information
Funding
References
- Tara N, Iqbal M, Mahmood Khan Q, et al. Bioaugmentation of floating treatment wetlands for the remediation of textile effluent. Water Environ J. 2019;33(1):124–17. doi: 10.1111/wej.12383
- Panda S, Kar R, Panda C. Isolation and identification of petroleum hydrocarbon degrading microorganisms from oil contaminated environment. Int J Environ Sci. 2013;3(5):1314.
- Afzal M, Arslan M, Müller JA, et al. Floating treatment wetlands as a suitable option for large-scale wastewater treatment. Nat Sustainability. 2019;2(9):863–871. doi: 10.1038/s41893-019-0350-y
- Kovalakova P, Cizmas L, McDonald TJ, et al. Occurrence and toxicity of antibiotics in the aquatic environment: A review. Chemosphere. 2020;251:126351. doi: 10.1016/j.chemosphere.2020.126351
- Klein EY, Van Boeckel TP, Martinez EM, et al. Global increase and geographic convergence in antibiotic consumption between 2000 and 2015. Proc Natl Acad Sci, USA. 2018;115(15):E3463–E3470. doi: 10.1073/pnas.1717295115
- Lv M, Zhang D, Niu X, et al. Insights into the fate of antibiotics in constructed wetland systems: Removal performance and mechanisms. J Environ Manage. 2022;321:116028. doi: 10.1016/j.jenvman.2022.116028
- Bhatt E, Gauba P. A sustainable approach for phytoremediation of amoxicillin using Ocimum basilicum. Current Trends Biotechnol Pharm. 2021;15(4):426–435.
- Sodhi KK, Kumar M, Singh DK. Insight into the amoxicillin resistance, ecotoxicity, and remediation strategies. Water Proc Eng. 2021;39:101858. doi: 10.1016/j.jwpe.2020.101858
- Elizalde-Velázquez A, Gómez-Oliván LM, Galar-Martínez M, et al. Amoxicillin in the aquatic environment, its fate and environmental risk. Environ Health Risk-Hazard Factors Living Species. 2016;1:247–267.
- Singh V, Pandey B, Suthar S. Phytotoxicity of amoxicillin to the duckweed Spirodela polyrhiza: Growth, oxidative stress, biochemical traits and antibiotic degradation. Chemosphere. 2018;201:492–502. doi: 10.1016/j.chemosphere.2018.03.010
- Githinji LJ, Musey MK, Ankumah RO. Evaluation of the fate of ciprofloxacin and amoxicillin in domestic wastewater. Water Air Soil Pollut. 2011;219(1):191–201. doi: 10.1007/s11270-010-0697-1
- Li M, Cha DJ, Lai Y, et al. The antimicrobial peptide‐sensing system aps of Staphylococcus aureus. Mol Microbiol. 2007;66(5):1136–1147. doi: 10.1111/j.1365-2958.2007.05986.x
- Sodhi KK, Kumar M, Balan B, et al. Isolation and characterization of amoxicillin-resistant bacteria and amoxicillin-induced alteration in its protein profiling and RNA yield. Arch Microbiol. 2020;202(2):225–232. doi: 10.1007/s00203-019-01737-6
- Yin Z. Distribution and ecological risk assessment of typical antibiotics in the surface waters of seven major rivers, China. Environ Sci Processes Impacts. 2021;23(8):1088–1100. doi: 10.1039/D1EM00079A
- Zhou Q, Liu G, Arif M, et al. Occurrence and risk assessment of antibiotics in the surface water of Chaohu Lake and its tributaries in China. Sci Total Environ. 2022;807:151040. doi: 10.1016/j.scitotenv.2021.151040
- Rice EW, Baird RB, Eaton AD, et al. Standard methods for the examination of water and wastewater. Vol. 10. Washington, DC: American public health association; 2012.
- Urtiaga A. Electrochemical technologies combined with membrane filtration. Curr Opin Electrochem. 2021;27:100691. doi: 10.1016/j.coelec.2021.100691
- Scaria J, Gopinath A, Nidheesh P. A versatile strategy to eliminate emerging contaminants from the aqueous environment: Heterogeneous Fenton process. J Clean Prod. 2021;278:124014. doi: 10.1016/j.jclepro.2020.124014
- Rathi BS, Kumar PS. Application of adsorption process for effective removal of emerging contaminants from water and wastewater. Environ Pollut. 2021;280:116995. doi: 10.1016/j.envpol.2021.116995
- Ahmed S, Mofijur M, Nuzhat S, et al. Recent developments in physical, biological, chemical, and hybrid treatment techniques for removing emerging contaminants from wastewater. J Hazard Mater. 2021;416:125912. doi: 10.1016/j.jhazmat.2021.125912
- Zhao L, Deng J, Sun P, et al. Nanomaterials for treating emerging contaminants in water by adsorption and photocatalysis: Systematic review and bibliometric analysis. Sci Total Environ. 2018;627:1253–1263. doi: 10.1016/j.scitotenv.2018.02.006
- Shah SWA, Rehman M, Hayat A, et al. Enhanced degradation of ciprofloxacin in floating treatment wetlands augmented with bacterial cells Immobilized on Iron oxide Nanoparticles. Sustainability. 2022;14(22):14997. doi: 10.3390/su142214997
- Ávila C, García-Galán MJ, Borrego CM, et al. New insights on the combined removal of antibiotics and ARGs in urban wastewater through the use of two configurations of vertical subsurface flow constructed wetlands. Sci Total Environ. 2021;755:142554. doi: 10.1016/j.scitotenv.2020.142554
- Kumar M, Sridharan S, Sawarkar AD, et al. Current research trends on emerging contaminants pharmaceutical and personal care products (PPCPs): A comprehensive review. Sci Total Environ. 2023;859:160031. doi: 10.1016/j.scitotenv.2022.160031
- Dutta A, Banerjee P, Sarkar D, et al. Degradation of Trypan Blue in wastewater by sunlight-assisted modified photo-Fenton reaction. Desalin Water Treat. 2015;56(6):1498–1506. doi: 10.1080/19443994.2014.950341
- Benvenuti T, Hamerski F, Giacobbo A, et al. Constructed floating wetland for the treatment of domestic sewage: a real-scale study. J Environ Chem Eng. 2018;6(5):5706–5711. doi: 10.1016/j.jece.2018.08.067
- Cerbaro KA, da Rocha RDC. Tolerance and phytoremediation capacity of the Lemna minor in an aqueous medium contaminated by the amoxicillin. Res, Soc Dev. 2022;11(7):e45711730251–e45711730251. doi: 10.33448/rsd-v11i7.30251
- Fahid M, Arslan M, Shabir G, et al. Phragmites australis in combination with hydrocarbons degrading bacteria is a suitable option for remediation of diesel-contaminated water in floating wetlands. Chemosphere. 2020;240:124890. doi: 10.1016/j.chemosphere.2019.124890
- Nakai S, Zou G, Okuda T, et al. Anti-cyanobacterial allelopathic effects of plants used for artificial floating islands. Allelopathy J. 2010;26(1):113–121.
- Srivastava JK, Chandra H, Kalra SJ, et al. Plant–microbe interaction in aquatic system and their role in the management of water quality: a review. Appl Water Sci. 2017;7(3):1079–1090. doi: 10.1007/s13201-016-0415-2
- Hashmat AJ, Afzal M, Arias CA, et al. Enhanced degradation of hydrocarbons in constructed wetlands aided with nutrients, surfactant, and aeration. Int J Phytoremediation. 2022;24(11):1163–1172. doi: 10.1080/15226514.2021.2021140
- Li X, Li Y, Xu D, et al. Effects of solar aeration on purification capacity of floating constructed wetlands with biochar. Environ Sci Technol (China). 2018;41(7):54–59.
- Li G, Tao L, Li X-L, et al. Design and performance of a novel rice hydroponic biofilter in a pond-scale aquaponic recirculating system. Ecol Eng. 2018;125:1–10. doi: 10.1016/j.ecoleng.2018.10.001
- Park JB, Sukias JP, Tanner CC. Floating treatment wetlands supplemented with aeration and biofilm attachment surfaces for efficient domestic wastewater treatment. Ecol Eng. 2019;139:105582. doi: 10.1016/j.ecoleng.2019.105582
- Yang X, Zhang S, Ju M, et al. Preparation and modification of biochar materials and their application in soil remediation. Appl Sci. 2019;9(7):1365. doi: 10.3390/app9071365
- Xiang W, Zhang X, Chen J, et al. Biochar technology in wastewater treatment: A critical review. Chemosphere. 2020;252:126539. doi: 10.1016/j.chemosphere.2020.126539
- Syafruddin S, Wieshammer G, Puschenreiter M, et al. Effect of N and P fertilisation and aeration on biodegradation of crude oil in aged hydrocarbon contaminated soils. Plant Soil Environ. 2010;56(4):149–155. doi: 10.17221/146/2009-PSE
- Ghosh K, Sarkar A. Evaluating urban wastewater remediation efficiency of the hydroponic vetiver System through predictive modelling using artificial neural network. Environ Technol Innov. 2021;24:102007. doi: 10.1016/j.eti.2021.102007
- Afzal M, Yousaf S, Reichenauer TG, et al. Soil type affects plant colonization, activity and catabolic gene expression of inoculated bacterial strains during phytoremediation of diesel. J Hazard Mater. 2011;186(2–3):1568–1575. doi: 10.1016/j.jhazmat.2010.12.040
- Afzal M, Yousaf S, Reichenauer TG, et al. The inoculation method affects colonization and performance of bacterial inoculant strains in the phytoremediation of soil contaminated with diesel oil. Int J Phytoremediation. 2012;14(1):35–47. doi: 10.1080/15226514.2011.552928
- Yasin M, Tauseef M, Zafar Z, et al. Plant-microbe synergism in floating treatment wetlands for the enhanced removal of sodium dodecyl sulphate from water. Sustainability. 2021;13(5):2883. doi: 10.3390/su13052883
- Ijaz A, Shabir G, Khan QM, et al. Enhanced remediation of sewage effluent by endophyte-assisted floating treatment wetlands. Ecol Eng. 2015;84:58–66. doi: 10.1016/j.ecoleng.2015.07.025
- Fernandes JP. Response of microorganisms from natural and constructed wetlands to veterinary drugs. 2014. doi: 10.1016/j.jsps.2013.12.005
- Aljeboree AM, Alshirifi AN, editors. Colorimetric determination of amoxicillin using 4-aminoantipyrine and the effects of different parameters. Journal of Physics: Conference Series; College of Science, University of Al-Qadisiyah, Iraq; IOP Publishing; 2019.
- Rehman K, Imran A, Amin I, et al. Inoculation with bacteria in floating treatment wetlands positively modulates the phytoremediation of oil field wastewater. J Hazard Mater. 2018;349:242–251. doi: 10.1016/j.jhazmat.2018.02.013
- Dhanve R, Jadhav J, Govindwar S. A study of textile effluent ecotoxicity and its biodegradation by an Exiguobacterium sp. RD3. Int J Curr Biotechnol. 2014;2(4):45–50.
- Saeed T, Sun G. A review on nitrogen and organics removal mechanisms in subsurface flow constructed wetlands: dependency on environmental parameters, operating conditions and supporting media. J Environ Manage. 2012;112:429–448. doi: 10.1016/j.jenvman.2012.08.011
- Bali A. Bioremediation of pharmaceutical wastes. In: Handbook of research on inventive bioremediation techniques. IGI Global; 2017. pp. 364–393.
- Singh T, Awasthi G, Tiwari Y. Recruiting endophytic bacteria of wetland plants to phytoremediate organic pollutants. Int J Environ Sci Technol. 2021;19(9):1–12. doi: 10.1007/s13762-021-03476-y
- Mahmoudpour M, Gholami S, Ehteshami M, et al. Evaluation of phytoremediation potential of vetiver grass (chrysopogon zizanioides (L.) roberty) for wastewater treatment. Adv Mater Sci Eng. 2021;2021:1–12. doi: 10.1155/2021/3059983
- Datta R, Das P, Smith S, et al. Phytoremediation potential of vetiver grass [Chrysopogon zizanioides (L.)] for tetracycline. Int J Phytoremediation. 2013;15(4):343–351. doi: 10.1080/15226514.2012.702803
- Davamani V, Parameshwari CI, Arulmani S, et al. Hydroponic phytoremediation of paperboard mill wastewater by using vetiver (Chrysopogon zizanioides). J Environ Chem Eng. 2021;9(4):105528. doi: 10.1016/j.jece.2021.105528
- Shaofeng Q, Hanxin T, Yingxue L, et al. Effect of combination biochar and magnesium oxide on nitrogen and phosphorus transformation in floating wetland. Environ Chem. 2022;41(7):2425–2434.
- Panja S, Sarkar D, Zhang Z, et al. Removal of antibiotics and nutrients by vetiver grass (Chrysopogon zizanioides) from a plug flow reactor based constructed wetland model. Toxics. 2021;9(4):84. doi: 10.3390/toxics9040084
- Chand N, Suthar S, Kumar K, et al. Removal of pharmaceuticals by vertical flow constructed wetland with different configurations: Effect of inlet load and biochar addition in the substrate. Chemosphere. 2022;307:135975. doi: 10.1016/j.chemosphere.2022.135975
- Demirezen DA, Yıldız YŞ, Yılmaz DD. Amoxicillin degradation using green synthesized iron oxide nanoparticles: Kinetics and mechanism analysis. Environ Nanotechnol, Monit Manage. 2019;11:100219. doi: 10.1016/j.enmm.2019.100219
- Liu N, Han H, Yin H, et al. Variations in the fate and risk analysis of amoxicillin and its degradation products during pig manure aerobic composting. J Hazard Mater. 2018;346:234–241. doi: 10.1016/j.jhazmat.2017.11.050
- Wang Y, Villamil MB, Davidson PC, et al. A quantitative understanding of the role of co-composted biochar in plant growth using meta-analysis. Sci Total Environ. 2019;685:741–752. doi: 10.1016/j.scitotenv.2019.06.244
- Zhuang L-L, Li M, Li Y, et al. The performance and mechanism of biochar-enhanced constructed wetland for wastewater treatment. Water Proc Eng. 2022;45:102522. doi: 10.1016/j.jwpe.2021.102522
- Sun P, Li Y, Meng T, et al. Removal of sulfonamide antibiotics and human metabolite by biochar and biochar/H2O2 in synthetic urine. Water Res. 2018;147:91–100. doi: 10.1016/j.watres.2018.09.051
- Zhang P, Peng Y, Lu J, et al. Microbial communities and functional genes of nitrogen cycling in an electrolysis augmented constructed wetland treating wastewater treatment plant effluent. Chemosphere. 2018;211:25–33. doi: 10.1016/j.chemosphere.2018.07.067
- Ming L, Ming L, Li Z-P, et al. Soil N transformation and microbial community structure as affected by adding biochar to a paddy soil of subtropical China. J Integr Agric. 2016;15(1):209–219. doi: 10.1016/S2095-3119(15)61136-4